CuO nanosheet as a recyclable Fenton-like catalyst prepared from simulated Cu(II) waste effluents by alkaline H2O2 reaction†
Received
23rd August 2018
, Accepted 31st October 2018
First published on 13th November 2018
Abstract
The direct discharge of copper containing wastewater into the water body not only causes serious harm to the ecological environment, but also results in copper resource wastage. A green approach was successfully developed to prepare CuO nanosheets (NSs) from simulated Cu(II) waste effluents by alkaline H2O2 reaction. The FTIR, EXAFS and XPS results revealed that peroxide group exists on the surface of the as-prepared CuO nanosheets, and the formation mechanism of copper peroxide has been proposed. Assisted by visible light irradiation, the CuO NS as a recyclable Fenton-like catalyst achieved complete degradation of phenol within 40 min. Moreover, the copper ions leached during the Fenton-like process were recycled via alkaline H2O2 reaction and their concentration in the solution reduced to below 1 mg L−1, therefore realizing a copper life cycle and Fenton-like degradation of organic pollutants.
Environmental significance
The direct discharge of copper ion effluents not only causes serious environmental issues, but also results in wastage of copper resource. Copper oxide nanomaterials have a wide range of applications due to their special physicochemical properties. Herein, a green and simple approach was successfully developed to prepare CuO nanosheets (NSs) from simulated Cu(II) waste effluents by alkaline H2O2 reaction. The as-prepared CuO NSs functioned as efficient Fenton-like catalysts with assistance of visible light irradiation. Moreover, the copper ions leached during the Fenton-like process were recycled via alkaline H2O2 reaction, therefore realizing a copper life cycle and Fenton-like degradation of organic pollutants.
|
Introduction
Copper oxide (CuO) nanomaterial has been widely studied for application in gas sensors,1 photocatalysis,2 lithium-ion batteries,3,4 and solar cell devices5 due to its abundance, nontoxicity and chemical stability. Up to now, CuO nanowires,6 nanorods,7 nanotubes,8 hollow structures,9 and nanosheets structures10 have been synthesized for specific utilization. Moreover, mine drainage, electroplating wastewater, and printed circuit board production process of ammonia–alkali etching solution contain a certain concentration of copper ions; for instance, the concentration of copper in pyrophosphate copper plating wastewater is around 50 mg L−1 and the corresponding pH value is about 7.11
Copper contamination has been a priority pollution in China.12 The direct discharge of copper-contaminated wastewater into the water body causes serious harm to the ecological environment. Therefore, it is very promising to achieve the effective recovery of copper ions for simultaneous wastewater treatment of heavy metals and recycling of Cu resource.
Traditional methods for removal of copper ions, such as chemical precipitation, ion exchange, adsorption and membrane filtration, produce a large amount of copper sludge, which results in secondary pollution, and some of them are costly and cannot be reused.13–15 To achieve an effective treatment of copper-containing wastewater as well as recovery of copper ions, considerable efforts have been made. Li et al. prepared a functionalized flexible carbonaceous aerogels/metal oxide hybrid based on capacitive deionization technology to retrieve small amounts of copper ions from solutions.16 Troupis et al. proposed that polyoxometalates can be used as photocatalysts to absorb near-visible and UV light for photocatalytic reduction and recovery of copper from aqueous solution.17 In addition, Zhao et al. found that Cu recovery can be achieved from Cu–EDTA complex by persulfate activated through UV and cathodic reduction.18
The Fenton process, as one of the advanced oxidation processes (AOPs), has been widely used in wastewater treatment due to the ability to generate hydroxyl radicals that can oxidize organic pollutants to non-toxic carbon dioxide and water.19 However, the classical homogenous Fenton process has two distinct drawbacks: (1) operation condition is limited to pH 2–3 and (2) iron sludge is generated during the reaction, which causes secondary pollution. Continuous efforts have been devoted to solving the above problems and exploring the alternative metallic materials, particularly iron (hydro)xide-based heterogeneous Fenton-like nanocatalysts.20–23 Copper-based Fenton-like catalysts have been widely investigated due to their ability to react with H2O2 and generate hydroxyl radicals.24,25 Similar to the redox properties of iron, both Cu+ and Cu2+ can react with H2O2. However, it is easier to convert copper from high to low valence state compared with iron (eqn (1) to (4)).21,26,27 Moreover, the insoluble iron hydroxide complex blocks the cycle of Fe3+/Fe2+, whereas the copper hydra complex [Cu(H2O)6]2+ is predominant at pH > 5, revealing that copper-based heterogeneous catalysts can work at a broader pH range.28,29
| Cu2+ + H2O2 → Cu+ + HO2˙ + OH− (k = 4.6 × 102 M−1 s−1) | (1) |
| Cu+ + H2O2 → Cu2+ + OH˙ + OH− (k = 1.0 × 104 M−1 s−1) | (2) |
| Fe3+ + H2O2 → Fe2+ + HO2˙ + H+ (k = 0.001 − 0.01 M−1 s−1) | (3) |
| Fe2+ + H2O2 → Fe3+ + HO˙ + OH− (k = 63 − 76 M−1 s−1) | (4) |
H2O2 as a mild and clean chemical product is widely used in blanching, sterilization and oxidation. Herein, we propose a green and environment-friendly method to recover copper ions from wastewater and obtain CuO NSs by an alkaline H2O2 reaction. The obtained CuO NSs were used as efficient visible light Fenton-like catalysts for oxidation of organic pollutants (Scheme 1). Following this, around 95% of the Cu ions released during the Fenton-like reaction were recycled again by the alkaline H2O2 reaction. The recycled CuO NSs were successively used to degrade phenol for 4 runs, and all the contaminants were removed within 40 minutes. The copper ions leached during the degradation process were continually recovered to ensure that the copper ion concentration was lower than 1 mg L−1.
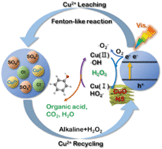 |
| Scheme 1 | |
Experimental section
Chemicals and reagents
Phenol, copper sulfate (CuSO4·5H2O), sulfuric acid, and ethylenediaminetetraacetic acid disodium salt were obtained from Sinopharm Chemical Reagent Co., Ltd. Sodium hydroxide (NaOH), hydrogen peroxide (H2O2, 30%), and copper chloride were obtained from Shanghai Lingfeng Chemical Reagent Co., Ltd. All chemicals were used without further purification and all aqueous solutions were prepared by using distilled and deionized water.
Characterization
X-ray diffraction (XRD) patterns were measured on a Shimadzu XRD-6100 diffractometer using Cu Kα as radiation. Fourier transform infrared (FT-IR) experiments were conducted on a Tensor 27 FTIR spectrometer (Nicolet 6700) using KBr pellets of the solid samples. A UV/vis/near IR spectrophotometer (Lambda 750S) was used to obtain the diffuse reflectance spectra. Raman spectra were recorded on a dispersive Raman Microscope (Senterra R200-L) with an excitation wavelength of 532 nm. Scanning electron microscopy (SEM) measurements were conducted on a MIRA3 (SEM) & INCA X-Act (EDS) with a 5 kV accelerating voltage. Transmission electron microscopy (TEM) results were obtained on a JEM 2100F microscope operated at 200 kV. X-ray photoelectron spectroscopy (XPS) data were obtained on a Kratos Axis Ultra DLD.
Electron paramagnetic resonance (EPR) measurement
All the EPR measurements were recorded at −20 °C using a JES FA200 EPR spectrometer (JEOL, Japan). For EPR measurements, the solid particles (15 mg) were directly filled into 4 mm quartz EPR tubes. Typical EPR instrument parameters were as follows: microwave frequency, 9064.544 MHz (X-band); microwave power, 0.99800 mW; modulation frequency, 100 kHz; sweep time, 2.0 min; sweep width, 2.5 × 10 mT; centre field, 323.9 mT; and time constant, 0.03 s.
Copper ions detection
The concentration of copper ions was measured on a contrAA800 continuous light source atomic absorption spectrometer.
Chloride ions detection
Chloride ions were measured using ion chromatography (IC, MIC system, Metrohm) equipped with a Metrosep A Supp 5–520 column (4.0 × 250 mm, 5 μm). The elution model was 3.2 mM Na2CO3/1.0 mM NaHCO3 with a flow rate of 0.70 mL min−1.
Recycling of CuO nanosheets by alkaline H2O2 reaction from Cu(II) waste solution
Laboratory simulated copper recovery was performed in a beaker with 40 mg L−1 copper ions. In a typical Cu2+ recovery procedure, 60 mL of simulated CuSO4 wastewater (0.15 mmol L−1) was adjusted to different pH values (pH 5–12) with 1 M NaOH. Then, 600 μL H2O2 (30%) was added into the above solution. After magnetic stirring, a brown precipitate appeared, which was collected by filtration and washed three times with distilled water and dried at 30 °C overnight.
Cu(II) waste solution with chloride
Recovery of copper from copper chloride solution was conducted under an initial concentration of 40 mg L−1 copper ions (about 45 mg L−1 chloride ion) by the same procedure mentioned above. The recovery efficiency of Cu2+ ions is defined as RCu(%), as shown in the following equation:
RCU(%) = 100 × (initial concentration of Cu2+ ion − remaining concentration of Cu2+ ions)/initial concentrations of Cu2+ ions. |
Fenton-like oxidation of phenol
Fenton-like oxidation of phenol was performed in dark and visible light irradiation for evaluating the CuO NSs recycled from Cu(II) wastewater. Typically, 10 mg of CuO NSs and 60 mL of 50 mg L−1 phenol was added into a beaker and stirred for 30 min to achieve uniform dispersion and adsorption/desorption equilibrium of phenol. Then, pH was adjusted to a desired value using 0.1 M NaOH and 0.1 M H2SO4. Next, 600 μL H2O2 was added and the beaker was placed under a Xe lamp with two 420 nm cut filters (100 mW cm−2) with a water jacket at 30 °C. Samples were taken at different time intervals using 2 mL syringes, filtered through 0.22 μm filter film, and quenched with excess isopropanol.
The concentration of phenol was determined by high-performance liquid chromatography (HPLC, SPD-16, Shimadzu Co., Japan) equipped with a UV-vis detector and a WondaSil C18-WR column (4.6 mm × 250 mm, 5 μm). The mobile phase was a binary mixture of methanol and ultra-pure water (50
:
50, v/v) with a flow rate of 1.0 mL min−1; the injection volume was 20 μL, and the UV/vis detector was operated at 270 nm.
Results and discussion
Recycling of CuO NSs
The effect of pH values and H2O2 dosages on recycling efficiency of Cu2+ ions was investigated. As shown in Fig. 1A, the precipitation process rapidly reaches equilibrium with the addition of H2O2 at pH ranging from 5–12. The recovery efficiency of Cu2+ ions at initial pH of 5, 7 and 9 are 48%, 51% and 50%, respectively. It increases significantly to 88% and 99% with pH further increasing to 11 and 12, respectively. The above results indicate that high alkalinity is beneficial for the recovery of Cu2+ ions. Accordingly, pH 12 was chosen as the optimal pH value in the subsequent experiment. Subsequently, we explored the effect of H2O2 during the Cu2+ ions recovery. Fig. 1B shows that the Cu ions were precipitated within the first 1 min with H2O2 dosages in the range of 0.6–100 mmol L−1, which indicates that the precipitation process occurs irrespective of the concentration of H2O2 and alkalinity should be responsible for Cu ion sedimentation. The photograph in Fig. S1 (ESI†) shows that the collected fresh copper precipitate is deep brown, while the commercial CuO is black.
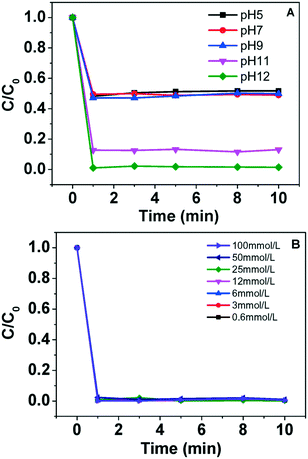 |
| Fig. 1 Effects of pH (A) and H2O2 dosage (B) on recovery of Cu2+ ions. Experimental conditions: 40 mg L−1 Cu2+ ions, [H2O2] = 0.6–100 mmol L−1, initial pH = 12. | |
Purity of the recycled CuO is very important for practical use. Accordingly, the recycling of Cu in the presence of chloride is a big challenge for traditional precipitation method.30 The recycled CuO with low chloride content becomes highly desirable. Our results show that the recycled CuO NSs have only about 5% chloride after recycling of copper chloride wastewater with initial chloride concentration of 45 mg L−1, which indicates that recycling of copper via alkaline H2O2 reaction is one of the promising wet chemistry methods of Cu ion recycling from wastewater.
Characterization of the recycled CuO NSs
XRD patterns of CuO recycled with different H2O2 concentrations and commercial CuO are shown in Fig. 2. The dominant diffraction peaks at 32.5°, 35.5°, 38.7°, and 48.7° are ascribed to (110), (002), (111), and (202) planes of CuO (JCPDS: 48-1548), respectively. The diffraction peaks of recycled CuO are consistent with those of commercial CuO. However, the peak at 23.8° appears in the XRD patterns of the precipitates obtained using 6 mmol L−1 and 12 mmol L−1 H2O2, which is ascribed to the peak of Cu(OH)2,31 suggesting that Cu(OH)2 appears as H2O2 concentration decreases.
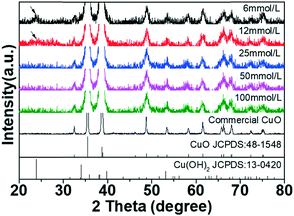 |
| Fig. 2 Wide angle XRD patterns of commercial CuO and recycled CuO NSs collected with different H2O2 concentrations. | |
SEM images of the recycled CuO NSs show wrinkled nanosheet-like aggregate structures (Fig. 3A). TEM images clearly show that the recycled CuO possesses very thin nanosheet structure (Fig. 3B, Fig. S3†). HR-TEM images show domains with lattice spacings of 0.23 nm, 0.25 nm and 0.27 nm, which are consistent with the (111), (002) and (110) planes of CuO, respectively, and agree with the XRD results. Based on the HR-TEM images, many tiny nanocrystals were separated from the amorphous domains, indicating the poor crystallinity of recycled CuO nanosheets (Fig. 3C and D).
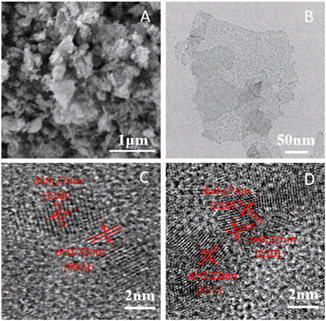 |
| Fig. 3 SEM images (A) and TEM images of recycled CuO NSs (B–D). | |
In the FT-IR spectra of CuO NSs, the evident peak positioned at 1116 cm−1 can be assigned to the stretching vibration of the –O–O– bond (Fig. 4A).32 The stretching vibrations at 596 cm−1, 529 cm−1 and 435 cm−1 are three characteristic peaks of CuO.33 Moreover, the vibrational peak at 844 cm−1 is assigned to Cu–O–H groups present in CuO NSs recycled in low H2O2 concentrations (6 and 12 mmol L−1). The above results are consistent with the XRD results. The peaks at 1388 and 1471 cm−1 are characteristic of CO32− due to dissolved CO2 from the air under alkaline conditions.33,34 The broad peaks at 3049 cm−1 and 1625 cm−1 indicate the existence of the surface –OH groups.35 In the Raman spectrum, the 875 cm−1 stretching mode, which is ascribed to O–O stretching, appears for the precipitation collected in H2O2 concentration above 25 mmol L−1 (Fig. 4B).36 The above results reflect that peroxo–copper bonds were present in the recycled CuO.
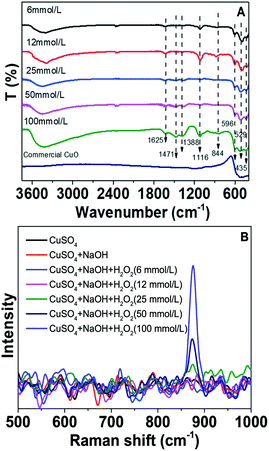 |
| Fig. 4 FT-IR spectra (A) and Raman spectra (B) of commercial CuO and CuO NSs recycled in different H2O2 concentrations. | |
UV-vis diffuse reflectance spectra of recycled CuO NSs and commercial CuO show that CuO NSs possess stronger absorption in the visible region (<700 nm) compared with that of the commercial CuO powders, while absorption in near infrared region (700–900 nm) is weaker (Fig. S4†). The estimated Eg of recycled CuO NSs and commercial CuO powders according to the formula αhν = A(hν·Eg)n/2,37 were 1.51 and 1.39 eV, respectively. The increased bandgap for recycled CuO nanosheets can be associated to the crystallite size and surface defects.37
The wide scan XPS spectrum of photoelectron peaks in Fig. S5† shows the presence of C 1s, O 1s, and Cu 2p. For O 1s spectrum, besides the peak at 530.0 eV and 531.6 eV ascribed to Cu–O, another peak at 533.4 eV correlates to the peroxo complexes (–O–O–) for fresh CuO NSs (Fig. 5A).38,39 The Cu 2p XPS spectrum of commercial CuO contains a peak at 933.6 eV, which confirms the existence of Cu2+ (Fig. 5B).40 The peak present at 932.2 eV in the spectrum of commercial Cu2O can be assigned to Cu+.41 Compared to commercial CuO and Cu2O, the recycled CuO NSs display similar peaks at 933.6 eV, which are assigned to Cu2+ species.42 XANES results show that the absorption edge position of recycled CuO nanosheets is similar to that of commercial CuO and the shape of the spectrum also resembles that of CuO (Fig. 5C). FT-EXAFS of the recycled CuO NSs shows peaks at 1.5 Å (Cu–O bond) and 2.6 Å (Cu–O–Cu bond), which are also obtained for commercial CuO. Therefore, the recycled CuO NSs are mostly composed of Cu(II) (Fig. 5D). Characteristic EPR signals of CuO NSs and commercial CuO are given in Fig. S6.† As shown in Fig. S6,† EPR data of the CuO NSs and commercial CuO show that a distinct singlet signal appears with g-factor of 1.9995 and 1.9994, respectively, which are similar. It has been reported that the characteristic resonance of Cu(II) with g-factor of 2.049 corresponds to the lattice trapped electron, while EPR resonance with lower g-factor of 1.9970–2.0020 corresponds to an electron trapped in the anionic vacancy of metal oxide.43
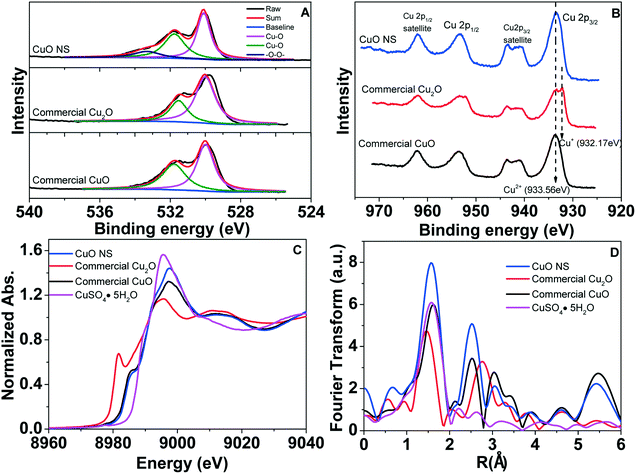 |
| Fig. 5 High resolution XPS spectra of the O 1s (A) and Cu 2p peak (B). XANE (C) and Fourier transforms of Cu K-edge XAFS (D) spectra. | |
The recycling mechanism of CuO NSs
The following reactions occurred for Cu(II) ions in alkaline H2O2 solution:44–47 | H2O2 + OH− → HO2− + H2O | (6) |
| Cu2+ + HO2− + OH− → CuO2 + H2O | (7) |
| CuO2 + H2O2 → CuO + O2 + H2O | (8) |
Based on the aforementioned results, CuO2 should be formed. The reaction rate of eqn (7) (4.8 × 1017 M−1) is much faster than that of eqn (6) (2.4 × 102), indicating that eqn (6) is the rate-determining step. This is consistent with the aforementioned results, indicating that excess H2O2 is crucial for obtaining pure CuO NSs. In contrast, Cu(OH)2 is formed as the concentration of H2O2 becomes lower than 12 mmol L−1. Additionally, alkaline medium is also very important. In basic solution, hydrogen peroxide reacts with OH− to form OOH− (eqn (6)). The formation of copper peroxide intermediates is promoted by increasing H2O2 and NaOH concentrations.44 Copper peroxide is unstable and further causes the catalytic decomposition of H2O2 into CuO and O2 with a small amount of peroxide remaining on the surface of CuO.45
Fenton-like degradation of phenol
Fenton oxidation of refractory organic pollutants in wastewater has been widely studied.19,22 In addition to Fe-based Fenton reagents and catalysts, CuO/H2O2 has also received significant attention recently due to its high efficiency and promotion effect on iron.42,48 As shown in Fig. 6A, the recycled CuO NSs are more active than the commercial CuO. The kinetics of phenol oxidation were investigated (Fig. 6B). The Fenton-like oxidation of phenol on CuO NSs and commercial CuO both displayed the pseudo-first-order kinetics model. The rate constants were 0.024 min−1 and 0.0081 min−1 for CuO NSs and commercial CuO, respectively, indicating much higher Fenton-like efficiency of CuO NSs than the commercial Fenton-like catalysts.
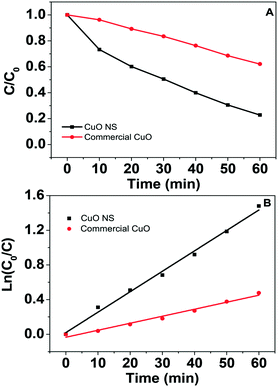 |
| Fig. 6 Degradation of phenol with recycled CuO NS and commercial CuO under dark condition (A) and the corresponding first order kinetic results (B). Experimental conditions: 50 mg L−1 phenol, 150 mg L−1 catalyst, initial pH = 6, [H2O2] = 100 mmol L−1. | |
Moreover, CuO is a narrow bandgap photocatalyst. Upon illumination in light with energy higher than the band gap of CuO, the electrons in the valence band of CuO can be excited to its conduction band. Accordingly, we measured the photo-Fenton performances of CuO NSs under visible light irradiation.
The effects of H2O2 dosage, catalyst concentration and initial pH value on the degradation of phenol were investigated under visible light condition. As can be seen from Fig. 7A, the removal rate of phenol on the catalyst increases gradually with the increase in H2O2 dosage. Fig. 7B illustrates that the removal rate increases through increasing the catalysts concentration from 0.080 to 0.15 g L−1, whereas on further increasing the concentration of the catalysts, the removal rate does not increase, which may be attributed to the light blocking effect of the catalyst. Fig. 7C shows that lower initial pH value is beneficial to accelerate the degradation of phenol. Metal leaching with pH value variation during the degradation process is shown in Fig. 7D. The concentration of leached copper ions is 20.34 mg L−1, 13.58 mg L−1 and 10.45 mg L−1 at pH 3, 6 and 9, respectively.
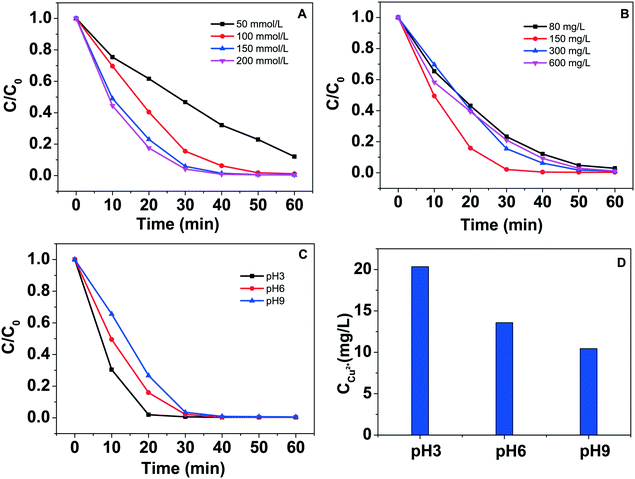 |
| Fig. 7 Effects of H2O2 dosage (A), catalysts dosages (B) and pH value (C) on Fenton-like degradation of phenol under visible light irradiation. Cu ion leaching with pH value variation during the phenol degradation (D). Experimental conditions: 50 mg L−1 phenol, 150 mg L−1 catalyst, initial pH = 6, [H2O2] = 100 mmol L−1. | |
According to the results shown in Fig. 7D, Cu ion leaching is considerable during the visible light photo-Fenton degradation of phenol. The amount of leached Cu ions increases on lowering the pH values from 9 to 3. In order to collect the leached Cu ion, we also recycled the copper ions via alkaline H2O2 strategy (pH = 12). After addition of H2O2, the copper ion concentration in the solution reduced to below 1 mg L−1, and the recycling efficiency was around 95%.11,49,50 The successive four-round phenol oxidation and Cu ion recycling were implemented and the recycled CuO NSs maintained excellent Fenton-like oxidation performance (Fig. 8A). For the four recycling runs of Cu ions, the concentration of soluble copper ions after alkaline H2O2 reaction reduced to 0.91 mg L−1, 0.80 mg L−1, 0.60 mg L−1 and 0.46 mg L−1, and the corresponding recovery efficiencies were 93%, 93%, 94% and 96%, respectively (Fig. 8B). Both natural water and industrial wastewater contain a certain concentration of inorganic anions, particularly SO42− and Cl−. These inorganic anions may have a great influence on the decomposition of H2O2 and the degradation of organic matter. As reported previously, in the phenol degradation Fenton-like reaction, the addition of ClO4−, NO3− and SO42− has negligible effect on phenol degradation and H2O2 decomposition, but the addition of Cl− has a great influence on the reaction.51,52 The main reason is that Cl− can capture free radicals, resulting in a decrease in H2O2 decomposition rate and phenol degradation (Fig. S7†).51
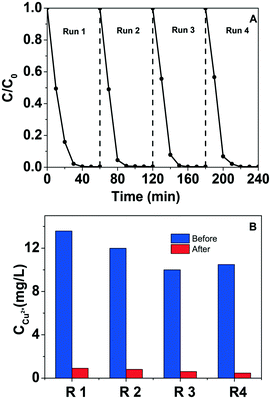 |
| Fig. 8 Reusability of recycled CuO NSs under visible irradiation (A) and the leached copper ion concentrations before and after alkaline-H2O2 recycling (B). Experimental conditions: 50 mg L−1 phenol, 150 mg L−1 catalyst, initial pH = 6, [H2O2] = 100 mmol L−1. | |
The mechanism of Fenton-like degradation of phenol
The Fenton-like oxidation of phenol in different conditions is shown in Fig. 9A. Phenol was hardly removed by the CuO NSs photocatalyst without H2O2 and visible light irradiation, whereas the degradation efficiency slightly improved to 23% within 30 min in the presence of H2O2 with visible light irradiation. However, it can be seen that the intermediate benzoquinone was still present after 1 h-reaction, indicating that phenol was partially oxidized with H2O2 in visible light (Fig. S8A†). Oxidation efficiency greatly improved to 100% within 30 min with CuO/H2O2 under visible light irradiation and all of the benzene rings were opened during the Fenton-like reaction (Fig. S8B†). In comparison, only 50% of phenol was degraded with CuO/H2O2 in dark (Fig. 9A) and intermediate benzoquinone was not found (Fig. S8C†). We also measured the concentration of leached Cu2+ ion (14 mg L−1) during the above reaction with CuO/H2O2 upon visible light irradiation. As shown in Fig. 9A, ∼75% of phenol was degraded with 14 mg L−1 Cu2+/H2O2 under visible light. The corresponding reaction rate constants at different reaction conditions were 0.0015 min−1, 0.0080 min−1, 0.0085 min−1, and 0.13 min−1 (Fig. 9B). The above results indicate that the visible light-assisted Fenton-like degradation performances of CuO NSs significantly enhanced in comparison with that of the homogeneous Cu2+ ions in the same condition. Moreover, we compared the visible light-assisted Fenton-like degradation performance of organic pollutants between CuO NSs and the reported iron oxides. We found that CuO NS is a promising Fenton-like catalyst in the presence of H2O2.
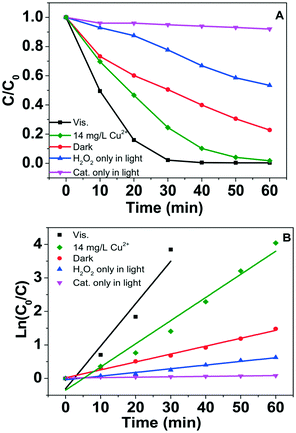 |
| Fig. 9 Degradation of phenol with various system (A) and the relative first order kinetic results (B). Experimental conditions: 50 mg L−1 phenol, 150 m L−1 catalyst, initial pH = 6, [H2O2] =100 mmol L−1. | |
Radical trapping experiments were conducted to indicate the contribution of ˙OH and/or ˙HO2/O2˙− to phenol degradation. In the presence of isopropanol, which is used to quench ˙OH, phenol was hardly removed during the 60 min-reaction (Fig. 10A). On addition of benzoquinone for ˙HO2/O2˙− quenching, only about 53.4% of phenol was removed at 30 min in comparison with the control experiment. The above results reveal that ˙OH radicals are the predominant oxidants for phenol degradation, while ˙HO2/O2˙− species also participate in the phenol degradation process. It is well-established that free copper ions (Cu(I, II)) are redox-active species that produce active oxygen species (˙OH, ˙HO2, eqn (1) and (2)). Our results also indicate that Cu ion leaching is significant during the Fenton-like reaction (Fig. 8B). Accordingly, the radicals such as ˙OH and ˙HO2 should be produced by the free copper ions, i.e., Cu(I, II). However, CuO NSs showed higher Fenton-like degradation performance in comparison with Cu2+ ions. Our previous results indicate that photo-generated e− on semiconductor Fenton-like catalysts can participate in the recycling of Fe(III)/Fe(II) at the interface of iron compounds.22,23 Fig. S9† shows cyclic voltammograms of the CuO NSs electrode obtained at a scan rate of 100 mV s−1 in a solution of 0.5 M Na2SO4 (pH = ∼6). As shown in the figure, the current increased from ∼0.18 mA cm−2 to ∼0.47 mA cm−2 after introducing visible light irradiation. The performance enhancement of CuO NS should be related to the photo-generated charges that can promote the generation of Cu(I). Auger Cu LMM spectrum of the catalyst was recorded, as shown in Fig. 10B. After the Fenton-like reaction, the shoulder peak of fresh CuO nanosheets at 568.9 eV shift to 569.3 eV, confirming the formation of lower valence state Cu+ species after the reaction, indicating that Cu+ was involved in the phenol oxidation reaction.53,54
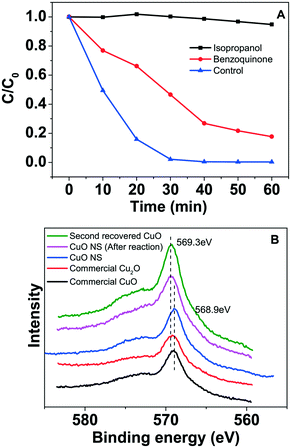 |
| Fig. 10 Effects of radical scavengers on the phenol degradation under visible light irradiation (A), Cu LMM Auger spectra of commercial CuO, commercial Cu2O, CuO NS before and after reaction and second recovered CuO NS (B). | |
Conclusions
In summary, CuO nanosheet was successfully collected from simulated Cu(II) waste effluents by alkaline H2O2 reaction for preparing a recyclable Fenton-like catalyst. The copper ion concentrations in the effluents can be significantly reduced to meet the emission standards through the alkaline H2O2 reaction. Various characterizations were employed to identify the structural, morphological and elemental features of the obtained CuO NSs. In addition, the CuO NSs presented promising Fenton-like degradation performance for phenol assisted with visible light irradiation. Both ˙OH and ˙HO2/O2˙− were found to be the active species in the Fenton-like degradation of phenol on CuO NSs. Overall, our study provides a new strategy for obtaining CuO NS, which is an efficient Fenton-like catalyst for phenol degradation. The leached copper ions can also be recycled by alkaline H2O2 reaction.
Conflicts of interest
There are no conflicts to declare.
Acknowledgements
This study was supported by National Natural Science Foundation of China (21777097, 21507083, 21777096, 21522703, 21876114), IJLRC-Ministry of Education and the China Postdoctoral Science Foundation (Grant 2017M621483 and 2018T110397).
Notes and references
- J. Li, B. E. Bursten, B. Liang and L. Andrews, Noble Gas-Actinide Compounds: Complexation of the CuO Molecule by Ar, Kr, and Xe Atoms in Noble Gas Matrices, Science, 2002, 295, 2242–2245 CrossRef CAS PubMed.
- Z. Jin, X. Zhang, Y. Li, S. Li and G. Lu, 5.1% Apparent quantum efficiency for stable hydrogen generation over eosin-sensitized CuO/TiO2 photocatalyst under visible light irradiation, Catal. Commun., 2007, 8, 1267–1273 CrossRef CAS.
- S. Ko, J.-I. Lee, H. S. Yang, S. Park and U. Jeong, Mesoporous CuO Particles Threaded with CNTs for High-Performance Lithium-Ion Battery Anodes, Adv. Mater., 2012, 24, 4451–4456 CrossRef CAS PubMed.
- A. Banerjee, U. Singh, V. Aravindan, M. Srinivasan and S. Ogale, Synthesis of CuO nanostructures from Cu-based metal organic framework (MOF-199) for application as anode for Li-ion batteries, Nano Energy, 2013, 2, 1158–1163 CrossRef CAS.
- C. Zuo and L. Ding, Solution-Processed Cu2O and CuO as Hole Transport Materials for Efficient Perovskite Solar Cells, Small, 2015, 11, 5528–5532 CrossRef CAS PubMed.
- X. Wen, Y. Xie, C. L. Choi, K. C. Wan, X.-Y. Li and S. Yang, Copper-Based Nanowire Materials: Templated Syntheses, Characterizations, and Applications, Langmuir, 2005, 21, 4729–4737 CrossRef CAS PubMed.
- B. Liu and H. C. Zeng, Mesoscale Organization of CuO Nanoribbons: Formation of “Dandelions”, J. Am. Chem. Soc., 2004, 126, 8124–8125 CrossRef CAS PubMed.
- L. Gou and C. J. Murphy, Solution-Phase Synthesis of Cu2O Nanocubes, Nano Lett., 2003, 3, 231–234 CrossRef CAS.
- Y. Chang, J. J. Teo and H. C. Zeng, Formation of Colloidal CuO Nanocrystallites and Their Spherical Aggregation and Reductive Transformation to Hollow Cu2O Nanospheres, Langmuir, 2005, 21, 1074–1079 CrossRef CAS PubMed.
- G. Wang, J. Huang, S. Chen, Y. Gao and D. Cao, Preparation and supercapacitance of CuO nanosheet arrays grown on nickel foam, J. Power Sources, 2011, 196, 5756–5760 CrossRef CAS.
- L. Liu, R. Li, Y. Liu and J. Zhang, Simultaneous degradation of ofloxacin and recovery of Cu(II) by photoelectrocatalysis with highly ordered TiO2 nanotubes, J. Hazard. Mater., 2016, 308, 264–275 CrossRef CAS PubMed.
- X. Yue, W. Liu, Z. Chen and Z. Lin, Simultaneous removal of Cu(II) and Cr(VI) by Mg–Al–Cl layered double hydroxide and mechanism insight, J. Environ. Sci., 2017, 53, 16–26 CrossRef PubMed.
- L. Ma, Q. Wang, S. M. Islam, Y. Liu, S. Ma and M. G. Kanatzidis, Highly Selective and Efficient Removal of Heavy Metals by Layered Double Hydroxide Intercalated with the MoS42− Ion, J. Am. Chem. Soc., 2016, 138, 2858–2866 CrossRef CAS PubMed.
- M. Rahimi, Z. Schoener, X. Zhu, F. Zhang, C. A. Gorski and B. E. Logan, Removal of copper from water using a thermally regenerative electrodeposition battery, J. Hazard. Mater., 2017, 322, 551–556 CrossRef CAS PubMed.
- M. R. Awual, New type mesoporous conjugate material for selective optical copper(II) ions monitoring & removal from polluted waters, Chem. Eng. J., 2017, 307, 85–94 CrossRef CAS.
- J. Li, X. Wang, H. Wang, S. Wang, T. Hayat, A. Alsaedi and X. Wang, Functionalization of biomass carbonaceous aerogels and their application as electrode materials for electro-enhanced recovery of metal ions, Environ. Sci.: Nano, 2017, 4, 1114–1123 RSC.
- A. Troupis, A. Hiskia and E. Papaconstantinou, Photocatalytic Reduction and Recovery of Copper by Polyoxometalates, Environ. Sci. Technol., 2002, 36, 5355–5362 CrossRef CAS PubMed.
- H. Zeng, S. Liu, B. Chai, D. Cao, Y. Wang and X. Zhao, Enhanced Photoelectrocatalytic Decomplexation of Cu–EDTA and Cu Recovery by Persulfate Activated by UV and Cathodic Reduction, Environ.
Sci. Technol., 2016, 50, 6459–6466 CrossRef CAS PubMed.
- E. Neyens and J. Baeyens, A review of classic Fenton's peroxidation as an advanced oxidation technique, J. Hazard. Mater., 2003, 98, 33–50 CrossRef CAS PubMed.
- A. K. Patra, A. Dutta and A. Bhaumik, Mesoporous Core–Shell Fenton Nanocatalyst: A Mild, Operationally Simple Approach to the Synthesis of Adipic Acid, Chem. – Eur. J., 2013, 19, 12388–12395 CrossRef CAS.
- A. D. Bokare and W. Choi, Review of iron-free Fenton-like systems for activating H2O2 in advanced oxidation processes, J. Hazard. Mater., 2014, 275, 121–135 CrossRef CAS PubMed.
- X. Qian, M. Ren, Y. Zhu, D. Yue, Y. Han, J. Jia and Y. Zhao, Visible Light Assisted Heterogeneous Fenton-Like Degradation of Organic Pollutant via α-FeOOH/Mesoporous Carbon Composites, Environ. Sci. Technol., 2017, 51, 3993–4000 CrossRef CAS PubMed.
- X. Qian, Y. Wu, M. Kan, M. Fang, D. Yue, J. Zeng and Y. Zhao, FeOOH quantum dots coupled g-C3N4 for visible light driving photo- Fenton degradation of organic pollutants, Appl. Catal., B, 2018, 237, 513–520 CrossRef CAS.
- H. Lee, H.-J. Lee, J. Seo, H.-E. Kim, Y. K. Shin, J.-H. Kim and C. Lee, Activation of Oxygen and Hydrogen Peroxide by Copper(II) Coupled with Hydroxylamine for Oxidation of Organic Contaminants, Environ. Sci. Technol., 2016, 50, 8231–8238 CrossRef CAS PubMed.
- H. Jin, X. Tian, Y. Nie, Z. Zhou, C. Yang, Y. Li and L. Lu, Oxygen Vacancy Promoted Heterogeneous Fenton-like Degradation of Ofloxacin at pH 3.2–9.0 by Cu Substituted Magnetic Fe3O4@FeOOH Nanocomposite, Environ. Sci. Technol., 2017, 51, 12699–12706 CrossRef CAS PubMed.
- C. Walling and A. Goosen, Mechanism of the ferric ion catalyzed decomposition of hydrogen peroxide. Effect of organic substrates, J. Am. Chem. Soc., 1973, 95, 2987–2991 CrossRef CAS.
- Y. Wang, H. Zhao and G. Zhao, Iron-copper bimetallic nanoparticles embedded within ordered mesoporous carbon as effective and stable heterogeneous Fenton catalyst for the degradation of organic contaminants, Appl. Catal., B, 2015, 164, 396–406 CrossRef CAS.
- J. I. Nieto-Juarez, K. Pierzchła, A. Sienkiewicz and T. Kohn, Inactivation of MS2 coliphage in Fenton and Fenton-like systems: role of transition metals, hydrogen peroxide and sunlight, Environ. Sci. Technol., 2010, 44, 3351–3356 CrossRef CAS PubMed.
- Y. Ling, M. Long, P. Hu, Y. Chen and J. Huang, Magnetically separable core–shell structural γ-Fe2O3@Cu/Al-MCM-41 nanocomposite and its performance in heterogeneous Fenton catalysis, J. Hazard. Mater., 2014, 264, 195–202 CrossRef CAS PubMed.
- T. Kawashima, Recovering copper and copper chemicals from spent alkaline etchant, JOM, 1997, 49, 40 CrossRef CAS.
- X. Wen, W. Zhang, S. Yang, Z. R. Dai and Z. L. Wang, Solution Phase Synthesis of Cu(OH)2 Nanoribbons by Coordination Self-Assembly Using Cu2S Nanowires as Precursors, Nano Lett., 2002, 2, 1397–1401 CrossRef CAS.
- M. Schatz, V. Raab, S. P. Foxon, G. Brehm, S. Schneider, M. Reiher, M. C. Holthausen, J. Sundermeyer and S. Schindler, Combined Spectroscopic and Theoretical Evidence for a Persistent End-On Copper Superoxo Complex, Angew. Chem., Int. Ed., 2004, 43, 4360–4363 CrossRef CAS PubMed.
- Q. Yu, H. Huang, R. Chen, P. Wang, H. Yang, M. Gao, X. Peng and Z. Ye, Synthesis of CuO nanowalnuts and nanoribbons from aqueous solution and their catalytic and electrochemical properties, Nanoscale, 2012, 4, 2613–2620 RSC.
- H. Hadj Mokhtar, B. Boukoussa, R. Hamacha, A. Bengueddach and D. El Abed, CuCO3-CuO nanocomposite as a novel and environmentally friendly catalyst for triazole synthesis, RSC Adv., 2015, 5, 93438–93446 RSC.
- T. Zhang, H. Zhu and J.-P. Croué, Production of Sulfate Radical from Peroxymonosulfate Induced by a Magnetically Separable CuFe2O4 Spinel in Water: Efficiency, Stability, and Mechanism, Environ. Sci. Technol., 2013, 47, 2784–2791 CrossRef CAS PubMed.
- D. E. Root, M. Mahroof-Tahir, K. D. Karlin and E. I. Solomon, Effect of Protonation on Peroxo−Copper Bonding: Spectroscopic and Electronic Structure Study of [Cu2((UN−O−)(OOH)]2+, Inorg. Chem., 1998, 37, 4838–4848 CrossRef CAS PubMed.
- Y. Lu, X. Liu, K. Qiu, J. Cheng, W. Wang, H. Yan, C. Tang, J. K. Kim and Y. Luo, Facile synthesis of graphene-like copper oxide nanofilms with enhanced electrochemical and photocatalytic properties in energy and environmental applications, ACS Appl. Mater. Interfaces, 2015, 7, 9682–9690 CrossRef CAS PubMed.
- S. Lan, Y. Xiong, S. Tian, J. Feng and T. Xie, Enhanced self-catalytic degradation of CuEDTA in the presence of H2O2/UV: Evidence and importance of Cu-peroxide as a photo-active intermediate, Appl. Catal., B, 2016, 183, 371–376 CrossRef CAS.
- J. Seo, H. Lee, H.-J. Lee, M. S. Kim, S. W. Hong, J. Lee, K. Cho, W. Choi and C. Lee, Visible light-photosensitized oxidation of organic pollutants using amorphous peroxo-titania, Appl. Catal., B, 2018, 225, 487–495 CrossRef CAS.
- S. Jiao, X. Zhang, G.-H. Zhang, S. Wang and S. Li, Porous CuO microspheres architectures as high-performance cathode materials for aluminum-ion battery, J. Mater. Chem. A, 2018, 6, 3084–3090 RSC.
- X. Zhao, J. Zhang, M. Qiao, H. Liu and J. Qu, Enhanced Photoelectrocatalytic Decomposition of Copper Cyanide Complexes and Simultaneous Recovery of Copper with a Bi2MoO6 Electrode under Visible Light by EDTA/K4P2O7, Environ. Sci. Technol., 2015, 49, 4567–4574 CrossRef CAS PubMed.
- W. Zhao, C. Liang, B. Wang and S. Xing, Enhanced Photocatalytic and Fenton-Like Performance of CuOx Decorated ZnFe2O4, ACS Appl. Mater. Interfaces, 2017, 9, 41927–41936 CrossRef CAS PubMed.
- H. Zhao, X. Chen, X. Li, C. Shen, B. Qu, J. Gao, J. Chen and X. Quan, Photoinduced formation of reactive oxygen species and electrons from metal oxide–silica nanocomposite: An EPR spin-trapping study, Appl. Surf. Sci., 2017, 416, 281–287 CrossRef CAS.
- A. Lasner, The Constitution of Copper Peroxide and the Catalytic Decomposition of Hydrogen Peroxide, J. Chem. Soc., 1951, 904–910 RSC.
- K. K. Yin Luo and R. Irving, Epstein, Kinetics and Mechanism of H202 Decomposition Catalyzed by Cu2+ in Alkaline Solution, Inorg. Chem., 1988, 27, 2489–2496 CrossRef.
- M. K. Eberhardt, G. Ramirez and E. Ayala, Does the reaction of copper(I) with hydrogen peroxide give hydroxyl radicals? A study of aromatic hydroxylation, J. Org. Chem., 1989, 54, 5922–5926 CrossRef CAS.
- Y. Luo, M. Orban, K. Kustin and I. R. Epstein, Mechanistic study of oscillations and bistability in the copper(II)-catalyzed reaction between hydrogen peroxide and potassium thiocyanate, J. Am. Chem. Soc., 1989, 111, 4541–4548 CrossRef CAS.
- M. B. Gawande, A. Goswami, F.-X. Felpin, T. Asefa, X. Huang, R. Silva, X. Zou, R. Zboril and R. S. Varma, Cu and Cu-Based Nanoparticles: Synthesis and Applications in Catalysis, Chem. Rev., 2016, 116, 3722–3811 CrossRef CAS PubMed.
- Z. Wei, Y. Xiong, J. Chen, J. Bai, J. Wu, J. Zuo and K. Wang, Recovery of Cu(II) from aqueous solution by induced crystallization in a long-term operation, J. Environ. Sci., 2017, 69, 183–191 CrossRef PubMed.
- F. Liu, C. Shan, X. Zhang, Y. Zhang, W. Zhang and B. Pan, Enhanced removal of
EDTA-chelated Cu(II) by polymeric anion-exchanger supported nanoscale zero-valent iron, J. Hazard. Mater., 2017, 321, 290–298 CrossRef CAS PubMed.
- J. De Laat, G. Truong Le and B. Legube, A comparative study of the effects of chloride, sulfate and nitrate ions on the rates of decomposition of H2O2 and organic compounds by Fe(II)/H2O2 and Fe(III)/H2O2, Chemosphere, 2004, 55, 715–723 CrossRef CAS PubMed.
- W.-M. Wang, J. Song and X. Han, Schwertmannite as a new Fenton-like catalyst in the oxidation of phenol by H2O2, J. Hazard. Mater., 2013, 262, 412–419 CrossRef CAS PubMed.
- J. Li, Z. Zhang, Y. Ji, Z. Jin, S. Zou, Z. Zhong and F. Su, One-dimensional Cu-based catalysts with layered Cu–Cu2O–CuO walls for the Rochow reaction, Nano Res., 2016, 9, 1377–1392 CrossRef CAS.
- C. Song, Z. Zhao, H. Li, D. Wang and Y. Yang, CeO2 decorated CuO hierarchical composites as inverse catalyst for enhanced CO oxidation, RSC Adv., 2016, 6, 102931–102937 RSC.
Footnote |
† Electronic supplementary information (ESI) available. See DOI: 10.1039/c8en00930a |
|
This journal is © The Royal Society of Chemistry 2019 |
Click here to see how this site uses Cookies. View our privacy policy here.