Photochemical interactions between n-Ag2S and n-TiO2 amplify their bacterial stress response†
Received
18th October 2018
, Accepted 7th December 2018
First published on 10th December 2018
Abstract
Under the reducing conditions of wetlands and anaerobic wastewater treatment processes, nanosilver (n-Ag) released from consumer products reacts with sulfide to form Ag2S, which is believed to be stable and practically benign. However, in the natural environment, these nanosized silver sulfide particles (n-Ag2S) may interact with light and other nanomaterials to create oxidizing conditions that may destabilize n-Ag2S, releasing Ag+ and causing stress to microorganisms. We investigate this scenario by monitoring the ATP levels of E. coli exposed to mixtures of n-Ag2S and n-TiO2, another nanomaterial with high likelihood of environmental release. Under dark conditions, we find that sulfidation increases the threshold concentration for microbial stress relative to n-Ag. Under simulated solar irradiation (SSI), exposure to n-Ag2S alone does not depress bacterial ATP levels in the concentration range tested (≤1 mg L−1 as Ag). However in the presence of 1 mg L−1 n-TiO2 and under SSI, n-Ag2S causes synergistic toxic stress and enhances the production of reactive oxygen species (ROS). Based on the photochemistry of these nanomaterials and our measurements of dissolved Ag, we propose that under environmental conditions the ROS produced by n-TiO2 dissolves n-Ag2S, releasing Ag+ that can then be photoreduced on the surface of n-TiO2 to form a highly photoactive and phototoxic nanocomposite. This work reveals that under oxidizing conditions n-Ag2S is prone to transformations that cause microbial stress. Overall, we provide further evidence that chemical interactions between multiple nanomaterials under irradiation can dramatically change their toxic effects.
Environmental significance
The microbial stress caused by mixtures of nanomaterials can differ greatly from those of individual nanomaterials due to their photochemical interactions. In this report, we investigate mixtures of nanotitania (n-TiO2) and nanoscale Ag2S (n-Ag2S), nanomaterials that may occur together, for example, in wastewater treatment effluent. n-Ag2S is generally thought to be stable and benign. However, in natural water systems, the presence of light and other nanomaterials like n-TiO2, which create oxidizing conditions, may lead to the release of Ag+ from n-Ag2S. Under dark conditions, we observe that sulfidation increases the threshold concentration for bacterial stress. Yet, under simulated solar irradiation, n-Ag2S and n-TiO2 together induce synergistic toxic effects in exposed bacteria because of their enhanced production of reactive oxygen species.
|
Introduction
Sulfidation has been promoted as the “natural antidote” to the oxidative dissolution of n-Ag:1 transforming soluble and highly toxic n-Ag to Ag2S, a more stable material with lower or negligible toxicity.2–6 Following the detection of nanoscale silver sulfides (n-Ag2S) in the biosolids from a wastewater treatment plant (WWTP),7 many studies have investigated the sulfidation of n-Ag,8–14 which can occur in sulfide-rich reducing environments including wetlands,15 municipal sewer systems,16 and the anaerobic processes of wastewater treatment plants.12,17 Sulfidation can occur within a few hours at the sulfide concentrations detected in WWTPs (ppb – 10 ppm).17 Even partial transformation of n-Ag to Ag2S has been shown to decrease the toxicity of n-Ag.1 Given the likelihood of n-Ag sulfidation in reducing environments, it is unknown to what extent n-Ag2S is stable in photo-oxidizing environments and if its biological stress effects are modified.
Thus far, a few studies have demonstrated that sulfidation of n-Ag fails to completely eliminate its toxic potential. For instance, Li, et al. observed that n-Ag2S released dissolved Ag under conditions mimicking the disinfection of water with hypochlorite.18 They attributed the dissolution of n-Ag2S to attack by reactive oxygen species (ROS), produced by HClO decomposition, and observed an increase in lethality to exposed zebrafish embryos. Thalmann, et al. investigated the stability of silver sulfides under ozonation in a WWTP effluent and found that Ag2S was oxidized and silver chloride was formed.19 Their acute toxicity tests showed that the response of C. reinhardtii algae to n-Ag2S containing effluent after ozone treatment was similar to that of an effluent spiked with Ag+.
Transformations of n-Ag2S have also been examined under several environmental conditions. Probing the stability of silver sulfides in a simulated soil pore water, Collin, et al. found slow dissolution of n-Ag2S in the presence of NOM.20 While the majority of tests on the stability and toxic effects of silver sulfide have occurred under dark conditions, light can also play an important role in transforming nanomaterials.21,22 In two investigations examining sunlit aquatic environments, Li, et al. found that nanosized Ag2S did not release dissolved Ag under light alone.21 However, with light and Fe(III), they observed dissolution of Ag2S and reduction of Fe(III) to Fe(II) and that dissolved Ag later formed nanoparticles of metallic Ag.21,23 These results demonstrate that in aquatic environments and in the presence of light, n-Ag2S may be more dynamic and prone to transformation than previously thought.
In our previous investigations, we found that the bacterial stress response is greatly modified when n-Ag is part of a mixture with other photoactive nanomaterials (NMs), a scenario likely to occur in anthropogenically impacted areas due to the continued use and environmental release of engineered NMs. We have studied the interactions occuring between n-Ag, a soluble NM, and n-TiO2, a stable NM that acts as an adsorptive surface. Under dark conditions, we have found that n-TiO2 can mitigate the toxic effects of low n-Ag concentrations by adsorption of Ag+.24 However, under simulated solar irradiation, the mixture of NMs causes synergistic stress as measured by diminished bacterial ATP levels and disrupted cell membrane integrity.25 We proposed that a Ag/TiO2 nanocomposite forms under irradiation releasing greater amounts of ROS to enhance the phototoxicity of the mixture. Those studies, along with other previous work, demonstrate that the interactions between nanomaterials in complex environmental mixtures create emergent systems with complex chemistry and surprising effects to exposed organisms.22,26
One potential source of n-Ag2S is in WWTP effluent, a major input to many urban waterways.27 While the majority of NMs are removed to the biosolids in the treatment process, a small amount remains in solution and is continuously discharged with the effluent to receiving waters.28 Vriens, et al. detected Ag in the effluent of 59 out of the 64 Swiss WWTPs they sampled.29 The median concentration was 25 ng L−1, but values extended up to 185 ng L−1 total dissolved Ag. Their work did not detail the chemical speciation of Ag, that is, whether it was associated with sulfide, but many studies suggest that after processing in a WWTP, Ag will be released as Ag2S.12,16,30 These effluents will likely also discharge other highly used NMs, including n-TiO2.31–33
Since both nAg2S and n-TiO2 are photoactive semiconductors,34,35 we investigate their interactions under irradiation. We hypothesize that oxidizing conditions created by photocatalytic activation of n-TiO2 may instigate the cycling of Ag released from n-Ag2S. n-Ag2S formed in aquatic systems may be fully or partially sulfidized and may be dissolved to different extents by ROS. Under irradiation, dissolved Ag released from n-Ag2S may form a composite nanoparticle with enhanced phototoxicity as we found occurs in mixtures of n-Ag and n-TiO2.25 To investigate this hypothesis, we study the toxic effects to E. coli caused by exposure to a mixture of n-TiO2 with either partially or fully sulfidized n-Ag under irradiation and compared these effects to n-Ag with n-TiO2. We also probe the chemistry of these systems by measuring the release of dissolved Ag and production of ROS.
Experimental
Nanomaterial preparation
Citrate-stabilized n-Ag was synthesized as previously reported.22,24,25 To obtain Ag2S and Ag/Ag2S nanomaterials (NMs), this n-Ag was sulfidized with the addition of 10 mM sodium sulfilde solution (pH ∼ 12) while bubbling air to provide oxygen and facilitate n-Ag dissolution.17 The Na2S solution was prepared with sodium sulfide powder (Fisher). Because sodium sulfide powder can be partially oxidized, the Na2S concentration in the stock solution was verified by colorimetric titration (USGS method I-3480).36 After sulfidation, nanoparticle solutions were purged with nitrogen to remove the remaining oxygen and prevent the oxysulfidation reaction from continuing.17 Partially sulfidized n-Ag was formed by reacting 5 mL of 219 mg L−1 citrate-capped n-Ag with 0.46 mL of 10 mM Na2S and purging with nitrogen immediately. Fully sulfidized n-Ag (n-Ag2S) was formed by reacting 5 mL of 219 mg L−1 citrate-capped n-Ag with 1.5 mL of 10 mM Na2S for 1 hour. After purging with nitrogen, the solutions of nanomaterials were immediately moved to an anaerobic chamber (Coy labs) for purification and storage. Inside the anaerobic chamber, excess reagents were removed from nanomaterial solutions by dialysis (Spectrum Labs Float-a-lyzer G2, 0.5–1 kDa MWCO) with 3 buffer changes over 24 hours. All stock solutions of Ag-containing NMs were stored in the anaerobic chamber in Al foil wrapped tubes to avoid exposure to light.
Degussa P25 n-TiO2 was donated by Evonik Industries. P25 is a composite containing 84% anatase and 16% rutile phases.26 The nominal size of P25 is approximately 20 nm and particles are spherical. Detailed characterization of P25 is described in previous reports.37,38 Prior to use, a ∼1 g L−1 P25 stock suspension was sonicated for 20 min in an ultrasonic bath (Health-Sonics, 110 W, 42 kHz).
n-Ag and silver sulfides characterization
The extent of sulfidation was determined using X-ray absorption near edge structure (XANES) spectroscopy. Modulation of the energy was achieved with a Si(111) monochromator, giving a nominal energy resolution of 3.4 eV at the Ag K-edge. XANES data were collected in fluorescence mode with a four element silicon-drift detector (Vortex-ME4). Typically 3 to 4 scans were collected per sample and standards, with no apparent signal variation or deterioration between scans. XANES spectra of samples and references were normalized by fixing the absorption threshold to the nominal energy of the Ag K-edge (i.e., 25.51 keV), to perform phase identification via linear combination fitting (LCF) with known standards. A fit window of −30 to 90 eV above the threshold energy was used for the LCF procedure, which was implemented via the least-squares minimization routines available within Larch.39 LCFs were forced to sum one except when only one reference was used for the fit. The chi-square metric was used as the parameter for goodness of fit. Ag foil and Ag2S powder (Sigma Aldrich) were used as reference materials. These two references were sufficient for fitting the data from all samples as no other phases were detected. Screening of the nanomaterials prior to XAS was also done by X-ray diffraction (XRD, Stoe STADI-MP) and analysis was performed using JADE 9.5 software (Materials Data Inc.).40
Silver quantification of each nanomaterial was performed using ICP-OES (Thermo iCap 7600) of solutions containing nanomaterials digested in a mixture consisting of 35% trace metals nitric acid and 7.5% hydrogen peroxide. During digestion, the mixtures were heated overnight in a hot water bath at 65 °C. The residual dissolved silver in an aliquot of each NM stock solution after purification was measured by ICP-MS (Thermo iCapQ) after ultrafiltration (Pall Nanosep, 3 kDa MWCO). Concentrations of nanomaterials used are reported on an Ag mass basis.
Transmission electron microscopy (Hitachi HD-2300) was used to observe nanomaterials and a histogram of particle diameters was generated by measuring at least 100 particles for each sample using FIJI for image analysis.41 Dynamic light scattering experiments were performed to determine hydrodynamic diameters and the zeta potentials of the three Ag-containing nanomaterials (Malvern Zetasizer Nano). UV-vis absorbance (Shimadzu UV-2450) was also measured to monitor the evolution of n-Ag's surface plasmon resonance peak after sulfidation.
Bacteria preparation
Bacteria preparation was performed as described previously.24 Briefly, E. coli ATCC 25922 (American Type Culture Collection) was cultured in low-salt lysogeny broth (LB, Alfa Aesar) until the optical density reached a value of ∼0.4. The cells were washed twice with the exposure medium, Lake Michigan water (LMW), before dilution to an optical density of ∼0.08, corresponding to 107 cells per mL as measured by plate counting. LMW is a medium representing oligotrophic surface waters and was used to allow comparison between this study and our previous work.24,25 LMW was collected from the inlet of the Evanston Drinking Water Treatment plant. LMW was stored at 4 °C and was filtered prior to use through a 0.2 μm pore size filter (Nalgene, PES membrane) to remove small particles and bacteria. Chemical characterization of LMW is presented in Table S1 in the ESI.†
Bacterial ATP response
The response of bacterial ATP levels to NM exposure was measured using the BacTiter-Glo assay (Promega) as in our previous work.22,24,25 NM mixtures were prepared immediately prior to exposure tests. 10 μL of NM mixtures and 90 μL of bacteria suspension were added to a 96-well microtiter plate. Under dark conditions, bacteria were exposed to nanomaterials for 1 hour. During the exposure, the plate was agitated on an orbital shaker at 300 rpm (VWR). Three replicates for each exposure condition (n = 3) were included per microtiter plate, and at least 3 independent microtiter plates were analyzed to assess reproducibility.
For exposure tests under light, simulated solar irradiation (SSI) was provided by a Xe arc lamp operating at 900 W (Newport Oriel). The lamp output was attenuated by a water-filled chamber to reduce the heating associated with infrared light. A UV transmitting fused quartz diffuser (Thor labs) was placed in the lamp beam to broaden the area on the plate that was irradiated. The output was characterized by a spectroradiometer and the spectrum is provided in Fig. S1 of the ESI.† Under SSI, the exposure time was 15 min with plates agitated on the orbital shaker (300 rpm).
After either dark or light exposure, the BacTiter-Glo reagent (Promega) was mixed with samples in the microplate. The microplate was covered with foil and incubated for 5 min on an orbital shaker. Luminescence resulting from the assay reaction with bacterial ATP was measured using a BioTek Synergy MX microplate reader. At least 6 tests from multiple plates were averaged to generate means and their associated error bars (standard deviation). p-values were calculated using the Student's t-Test. All data processing and the calculation of p-values were performed in Microsoft Excel. The potential interference of the assay with ENMs was tested and no interference was found (Fig. S2 in the ESI†).
Reactive oxygen species (ROS) detection
ROS were quantified using methods similar to those we have previously described.22,25 Hydrogen peroxide was measured using phenol red as a colorimetric probe.42 20 μL of nanoparticle mixtures were added to 180 μL of Millipore™-filtered Milli-Q (MQ) H2O in each well of a microtiter plate and irradiated for 30 min. After SSI exposure, 10 μL of 1 g L−1 phenol red (Sigma-Aldrich), 10 μL 0.5 mg mL−1 horseradish peroxidase (type II, salt-free powder, Sigma-Aldrich), and 10 μL of 0.77 M NaOH were added to each well. After mixing, the absorbance at 610 was measured to detect the purple color of the reaction product of hydrogen peroxide and phenol red. Superoxide anion was measured using 2,3-bis(2-methoxy-4-nitro-5-sulfophenyl)-2H-tetrazolium-5-carboxanilide sodium salt (XTT sodium salt, Sigma-Aldrich).43 20 μL of 1 mM XTT, 20 μL of nanoparticle mixtures, and 160 μL MQ H2O were added to wells in the microtiter plate and exposed for 30 min to SSI. The absorbance at 470 nm, which corresponds to the formation of XTT formazan by reaction of XTT with O2˙−, was measured after exposure. Of all the ROS potentially formed, we chose to measure H2O2 because it is relatively stable and formed by reaction of other ROS and serves as a proxy for total ROS formation. We additionally measure O2˙− because it can be produced by photoactivation of n-Ag2S.34
Dissolved Ag measurements
Dissolved silver concentrations resulting from n-Ag and sulfidized n-Ag particles were measured under several different conditions. In all experiments, Ag-containing NMs suspended in MQ H2O were added to a petri dish (Falcon, polystyrene) immediately prior to the exposure test. An ultraclear film (Axygen) was used to seal the dish to prevent evaporation of solution. For all test conditions, solutions were agitated on an orbital shaker (300 rpm). After each test, aliquots were collected from the solution and nanoparticles were removed using ultrafilters (Pall Nanosep, 3 kDa MWCO) with centrifugation at 12000 rpm. Filtrate from aliquots were diluted to achieve required sample volume, acidified, and Ag was quantified by HR-ICP-MS (Thermo Element2). Tests were performed under dark and light conditions and included n-TiO2 or H2O2 to provide oxidizing conditions.
Results
Properties of n-Ag and sulfidized n-Ag
Nanomaterials used for the exposure testing and chemical evaluations were thoroughly characterized by several methods: TEM for size and morphology, XRD and XAS for phase analysis, ICP-OES for total silver content, UV-vis for optical properties, and DLS for hydrodynamic size and particle stability. In order to explore the effect of sulfidation extent on bacterial stress, we compared a partially and fully sulfidized n-Ag to 100% metallic n-Ag. Representative TEM images of all three Ag-containing nanomaterials and corresponding particle size histograms are presented in Fig. 1. The citrate stabilized n-Ag particles are spherical with an average diameter of 13 ± 7 nm. Partially sulfidized n-Ag are 38 ±18 nm, and fully sulfidized n-Ag are 17 ± 11 nm. The images in Fig. 1 show that upon sulfidation, the morphology changes and bridges form to connect particles, as others have observed.3,10
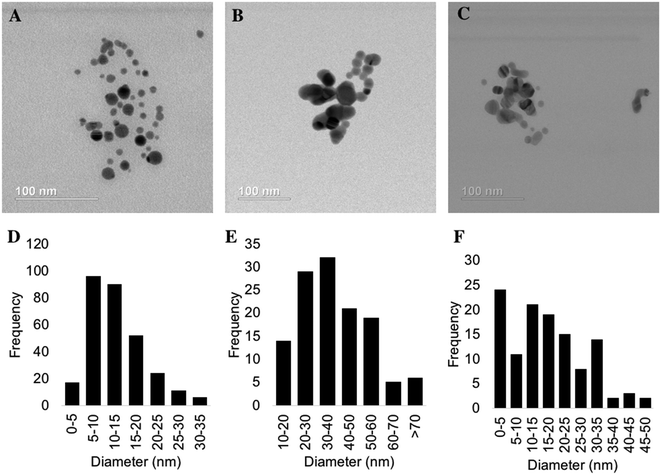 |
| Fig. 1 Characterization of NMs by TEM. Micrographs of (A) citrate capped n-Ag (B) partially sulfidized n-Ag and (C) fully sulfidized n-Ag. Histogram of particle sizes found by analyzing TEM images for (D) citrate capped n-Ag (E) partially sulfidized n-Ag and (F) fully sulfidized n-Ag. | |
Phase composition of nanomaterials was screened using XRD. Diffractograms are presented in Fig. S3 of the ESI.† The diffractogram for n-Ag shows the characteristsic reflections for metallic n-Ag (111 and 200) for this range of 2θ. For partially sulfidized n-Ag, the XRD pattern contains reflections from both metallic n-Ag (111 and 200) as well as those from n-Ag2S. The fully sulfidized n-Ag shows peaks characteristic of Ag2S and does not show those for metallic Ag. The XRD peaks of monoclinic Ag2S are low in intensity relative to metallic Ag, making phase quantification from these data difficult. Therefore, we relied on data collected from XAS for phase quantification. XANES spectra and fit to references are presented in Fig. 2. The signal of citrate stabilized n-Ag was found to correspond to 100% metallic Ag by linear combination fitting. Partially sulfidized n-Ag was found to be 37% Ag2S with the balance being metallic Ag. The signal from fully sulfidized n-Ag corresponded entirely with that of Ag2S. Throughout the paper, partially sulfidized n-Ag will be referred to as “37% Ag2S” and fully sulfidized n-Ag will be referred to as be “100% Ag2S.”
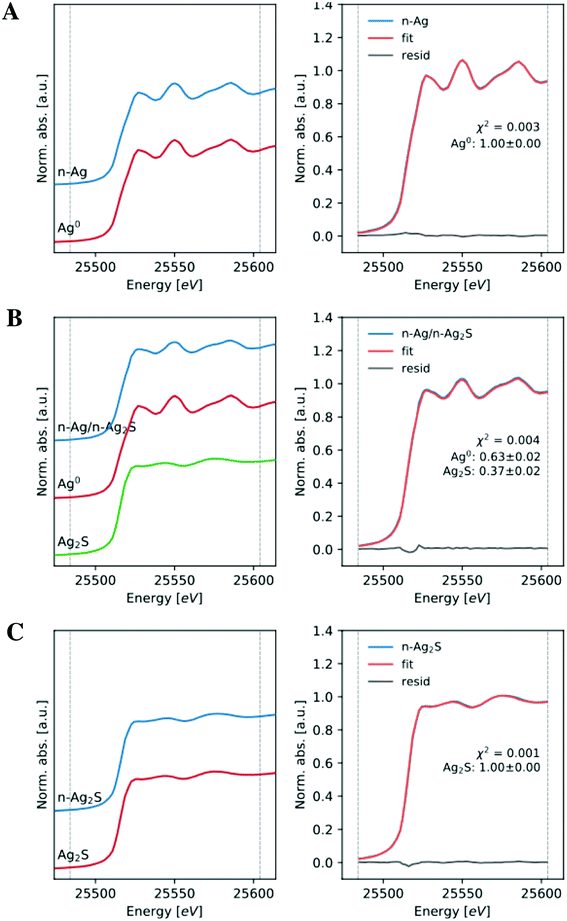 |
| Fig. 2 X-ray absorption near edge spectra and their corresponding linear combination fitting to determine the speciation of Ag in the nanoparticles used in the exposure experiments: (A) n-Ag, (B) partially sulfidized n-Ag (37% n-Ag2S and 63% n-Ag), and (C) fully sulfidized n-Ag (100% n-Ag2S). | |
UV-vis extinction spectra of n-Ag, 37% Ag2S and 100% Ag2S are presented in Fig. S4 of the ESI.† The spectrum for n-Ag shows a sharp peak at 388 nm, which is characteristic of the surface plasmon resonance (SPR) of metallic silver nanoclusters.44 A peak is present in the 37% Ag2S spectrum and is slightly shifted to 400 nm. The broadening of this peak relative to n-Ag is explained by the greater aggregation of partially suflidized n-Ag.45 100% Ag2S does not show a peak in the region of the n-Ag SPR.
Results of silver quantification by ICP methods and hydrodynamic diameter and zeta potential by DLS are presented in Table S2 of the ESI.† Zeta potentials for all three Ag-containing NMs were less than −25 mV, due to the presence of the citrate ligand. Consequently, these particles are considered moderately stable (±20–30 mV).46 Particles were stable over the four months that they were used for exposure testing and photochemical evaluation. The concentration of residual dissolved silver after purification and dilution to concentrations used for toxicity tests and chemical evaluations was below the target level (∼3 μg L−1) where Ag+ alone causes decreases in bacterial ATP levels.24
Bacterial exposure testing
The microbial stress response due to Ag-containing NMs was first investigated under dark conditions to understand their comparative stress and as a baseline prior to working with the NMs under light and in mixtures with TiO2. These results are presented in Fig. 3 and all NM concentrations are reported on an Ag mass basis. Here, bacterial ATP is proportional to the luminescence signal reported. Bacteria exposed to n-Ag experience a decrease in ATP levels at very low n-Ag concentration, starting between 20 and 40 μg L−1. In contrast, no stress was observed for the 100% Ag2S in the concentration range tested (1 mg L−1 and lower). E. coli exposed to 37% Ag2S showed a response that was an intermediate between the two extremes and the trends in the dose–response curve were shifted by an order of magnitude as compared to n-Ag. For tests using 37% Ag2S, a decrease in ATP levels was observed beginning at ∼750 μg L−1.
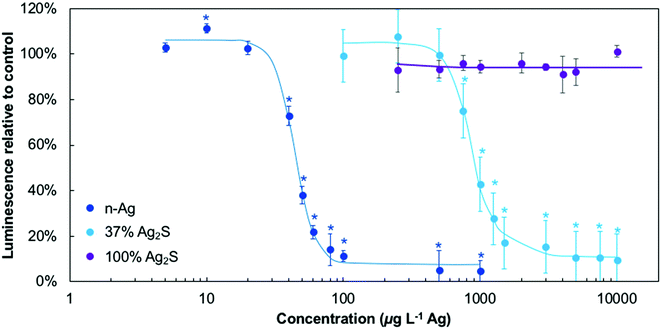 |
| Fig. 3 Effect of nanomaterials on bacterial ATP under dark conditions (1 h exposure time in LMW, n = 3). Trendlines depict four parameter logistic equation fit to data. Statistical significance (p < 0.05) for values compared with control is shown by asterisks in same color as dataset. | |
Fig. 4 depicts the effects of n-Ag, 37% Ag2S, and 100% Ag2S on bacterial ATP levels under SSI exposure, for Ag-containing NMs alone (Fig. 4A) and in a mixture with 1 mg L−1 P25 n-TiO2 (Fig. 4B). Exposure to n-Ag causes bacterial stress at concentrations lower than 100 μg L−1, while both 37% Ag2S and 100% Ag2S do not trigger toxic effects over the range tested. In Fig. 4B, the dashed line represents the minor effect of 1 mg L−1 n-TiO2 alone, a decrease of ATP to 88% relative to the control. When bacteria were exposed to n-TiO2 with n-Ag, 37% Ag2S, or 100% Ag2S, a synergistic stress effect on bacterial ATP was observed, that is, lower ATP levels were observed as compared to either the effects of the NMs by themselves or a simple summation of combined effects. For n-Ag with n-TiO2, a stress effect is observed at ∼10 μg L−1 n-Ag and confirms what we previously reported.25 The decrease in ATP levels to ∼40% caused by the mixture of 10 μg L−1 n-Ag with 1 mg L−1 n-TiO2 is similar to that caused by n-Ag alone at 100 μg L−1. Bacterial stress responses for 37% Ag2S or 100% Ag2S occur starting at ∼100 μg L−1, whereas no ATP depression was observed in the absence of TiO2 (Fig. 4A), and the responses of E. coli to NM dosage are very similar over the concentration range tested. The statistical significance for these data relative to the control and to n-TiO2 alone is given in Fig. S5 of the ESI.†
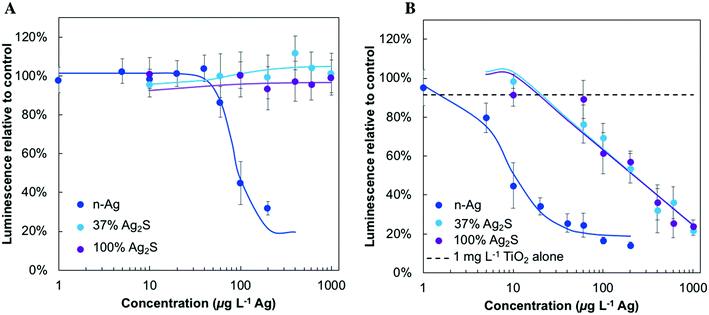 |
| Fig. 4 Effect of n-Ag, or partially or fully sulfidized n-Ag on bacterial ATP under simulated solar irradiation (15 min exposure time in LMW, n = 6). (A) without n-TiO2 (B) with 1 mg L−1 n-TiO2 (dashed line shows n-TiO2 alone for comparison). Results are normalized to a control with light exposure only (no NMs, 100% luminescence). Trendlines depict four parameter logistic equation fit to data. | |
Reactive oxygen species
To investigate whether the enhanced stress produced by mixtures under SSI occurred due to amplified photochemical ROS production, we measured the abiotic production of H2O2 and O2˙− using spectroscopic probes. Fig. 5A illustrates the production of H2O2 under SSI. 1 mg L−1 n-TiO2 causes a significant increase in the absorbance at 610 nm due to H2O2 production relative to the control while a significant increase is not observed for any of the Ag-containing NMs alone. When 20 μg L−1 n-Ag or 200 μg L−1 of 37% Ag2S or 100% Ag2S are exposed to SSI with 1 mg L−1 n-TiO2, the absorbance at 610 nm is increased over that of n-TiO2 alone, indicating that greater H2O2 production occurs with the mixture. Fig. 5B depicts the production of O2˙− measured. Here, a dramatic increase, between two and three-fold, in absorbance associated with the production of O2˙− results when any of the three Ag-containing NMs are present with TiO2 over n-TiO2 alone.
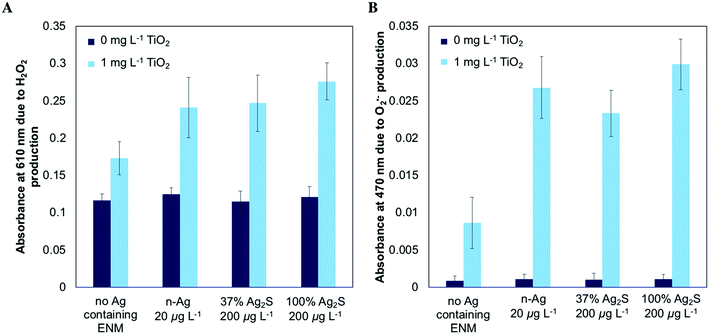 |
| Fig. 5 Production of (A) hydrogen peroxide and (B) superoxide anion as quantified by absorbance of probe molecules after exposure to SSI (n ≥ 6) from mixtures of n-Ag, 37% Ag2S, or 100% Ag2S with 1 mg L−1 TiO2. | |
Dissolved silver measurement
In order to probe the chemical transformations of these NM mixtures, we measured dissolved silver under several scenarios. These conditions included Ag-containing NMs alone or with 1 mg L−1 n-TiO2 as in our exposure test conditions, with H2O2 to provide oxidizing conditions without the adsorptive surface of n-TiO2, and under dark conditions. Fig. 6A shows the dissolved Ag resulting from 100 μg L−1 n-Ag. Low levels of dissolved Ag (<5 μg L−1) are observed for n-Ag alone in the dark and under SSI and for n-Ag with n-TiO2. Because n-TiO2 adsorbs Ag+,24 the presence of n-TiO2 can obscure whether dissolved Ag has been released from an Ag-containing NM. As shown in Fig. 5A, n-TiO2 produces H2O2 under our experimental conditions. Therefore, to simulate the presence of oxidizing species produced by n-TiO2, we measured the dissolved Ag for Ag containing NMs with H2O2. When n-Ag is combined with 1 mM H2O2, the dissolved Ag measured increases significantly. n-Ag is oxidized by H2O2,47 and here, the concentration after light exposure is slightly greater than that detected under dark conditions.
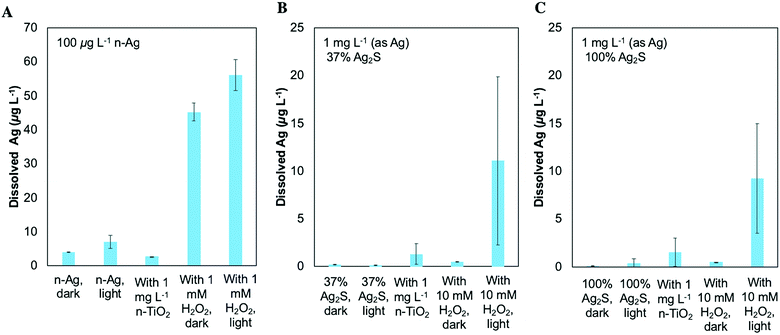 |
| Fig. 6 Dissolved Ag concentrations as detected by ICP-MS for (A) 100 μg L−1 n-Ag, (B) 1 ppm 37% Ag2S and (C) 1 ppm 100% Ag2S during 30 min exposure (n = 6 for SSI exposures, n = 3 for dark experiments). | |
Fig. 6B and C show the dissolved Ag resulting from 1 mg L−1 37% Ag2S and 1 mg L−1 100% Ag2S, respectively. Here, low concentrations of dissolved Ag (<5 μg L−1) were measured from these NMs alone under dark and light conditions, under light with n-TiO2, and under dark with 10 mM H2O2. Higher concentrations of dissolved silver were detected for both of these NMs after exposure under SSI with H2O2. Although the error bars for this condition are large, these data show qualitatively that an increase in dissolved Ag can occur under the scenario where highly oxidizing ROS are produced. For all three Ag containing NMs, the measurement of dissolved Ag under light with H2O2 indicates that they are likely prone to attack by the ROS also produced by n-TiO2 although this is not easily detected in the presence of n-TiO2 due to adsorption at the TiO2 surface.
Discussion and implications
Our results agree with those of previous studies reporting that sulfidation decreases the bacterial stress caused by n-Ag under dark conditions.3,4,6 In our experiments testing the acute toxic effects of Ag-containing NMs, partial sulfidation shifted the threshold concentration where E. coli exhibits stress as expressed by diminished ATP production an order of magnitude as compared to n-Ag. Complete sulfidation eliminated stress effects over the concentration range tested. These results are consistent with those presented by Reinsch, et al. where a partially sulfidized n-Ag with similar metallic/sulfidized silver composition had reduced toxic effects while the fully sulfidized n-Ag did not inhibit E. coli growth.3
We have previously shown that light drives important chemical reactions when n-Ag is present with n-TiO2 and amplifies the bacterial stress of the NM mixture22,25 beyond additive effects. This work extends our earlier finding to n-Ag2S and shows that it has similar interactions with n-TiO2 under light. While neither 37% nor 100% Ag2S impact bacterial ATP alone under SSI, they both cause decreases in ATP levels starting at a concentration of approximately 100 μg L−1 in the presence of n-TiO2. Under the oxidizing conditions created by n-TiO2, sulfidation of n-Ag fails to neutralize its toxic effects.
To determine whether this synergistic bacterial stress resulted from an enhancement in photoactivity with n-TiO2 and n-Ag2S, we investigated the production of ROS from these mixtures. Based on its bulk band gap (0.92 eV), Ag2S can produce O2˙− ,34 but we did not detect the production of ROS from any of the Ag-containing NMs alone under SSI. However, we did observe increased levels of H2O2 and O2˙− when n-TiO2 was present with any of the Ag-containing NMs. These results indicate that like n-Ag, 37% and 100% n-Ag2S photochemically interact with n-TiO2. In our work with irradiated mixtures of n-Ag and n-TiO2, we found that enhanced photoactivity resulted from the formation of a hybrid nanomaterial that self-assembled from the photreduction of Ag+ on the surface of n-TiO2.
To test whether this same interaction with n-TiO2 is also likely for partially sulfidized n-Ag or n-Ag2S, we measured dissolved Ag under oxidizing environments created by n-TiO2 and H2O2. We failed to detect an increase in dissolved Ag for Ag-containing NMs in the presence of n-TiO2 under SSI, presumably due to the fact that Ag+ adsorbs at the TiO2 surface. To simulate the ROS produced by n-TiO2 without the interference of an adsorptive surface, we used H2O2 and measured the Ag released from Ag containing NMs either under SSI or under dark conditions. H2O2 is a mild oxidant that can be photolyzed to produce hydroxyl radical (˙OH), which can react further with H2O2 to produce superoxide (O2˙−) through the following reactions:48
| ˙OH + H2O2/HO2− → HO2˙/O2˙− + H2O | (2) |
and O
2˙
− can also act as a source of H
2O
2 through the addition of another election in a proton-assisted reaction.
49 ROS can promote the dissolution of n-Ag (
ref. 50–52) and more recent studies have pointed to their role in dissolving Ag
2S through oxidation of the sulfur.
18,19 Our experiments with H
2O
2 demonstrate that these same ROS produced by n-TiO
2 can lead to the oxidative dissolution of n-Ag
2S, which liberates Ag
+ that can be reduced on the surface of n-TiO
2 to form a highly photoactive nanocomposite.
In Fig. 7 we sketch a cycle of interactions between n-TiO2 and n-Ag2S that results in the formation of a nAg/n-TiO2 nanocomposite. n-Ag released to the environment may be partially or fully sulfidized to produce n-Ag2S. Under irradiation and with n-TiO2, n-Ag2S releases Ag+ because of oxidation by ROS. Ag+ can then be adsorbed onto the surface of n-TiO2 and photoreduced there, forming a hybrid particle with increased photoactivity and ROS production. As we reported in our study on n-Ag and n-TiO2 mixtures, the Ag clusters on n-TiO2 likely extend the photoresponse of the composite into the visible range due to their plasmonic character.25 This type of composite would explain the greater phototoxicity and photoactivity observed here for mixtures of sulfidized n-Ag with n-TiO2. Although the concentrations released from n-Ag2S under similar environmental conditions would be quite low, the potential exists for n-Ag2S to interact and produce enhanced phototoxicity with n-TiO2, especially considering the release of both materials from WWTPs.
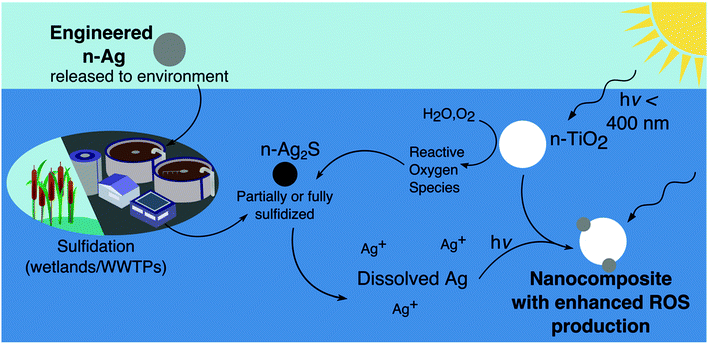 |
| Fig. 7 Schematic depicting interactions between n-Ag2S and n-TiO2 under irradiation in an oxygenated surface water. Partially or fully sulfidized n-Ag may be released after processing in a WWTP or wetland into surface water also containing n-TiO2. UV irradiation causes photocatalytic activation of n-TiO2 to produce ROS that oxidizes n-Ag2S causing release of Ag+. Dissolved Ag can be adsorbed onto surfaces. Adsorption of dissolved Ag and photoreduction on surface of n-TiO2 can lead to the formation of a nanocomposite with enhanced phototoxic effects to microorgansims. | |
Based on their commercial uses and applications in personal care products, both n-TiO2 and n-Ag will enter municipal waste streams.16,32 While the majority of n-TiO2 and sulfidized n-Ag may be removed during treatment, some Ag species and n-TiO2 are released in the effluent of WWTPs to surface waters53,54 where they can interact to produce amplified stress to exposed microoganisms. However, with advanced disinfection technologies such as UV treatment, these composite n-Ag/n-TiO2 NMs may form in the WWTP itself. UV disinfection has become common for treatment of municipal wastewater because, unlike chlorination, UV treatment does not cause the formation of halogenated dinsinfection byproducts.55 UV treatment would cause the photoactivation of n-TiO2 to produce ROS, which can dissolve sulfidized n-Ag and initiate the creation of nanocomposites as described earlier. These materials would then be discharged to effluent receiving waters and interact with microbial communities there.
Our results demonstrating the release of dissolved Ag from Ag2S under environmental conditions show that Ag2S is not a permanent sink for silver released from nanoenabled consumer goods. Instead, it is another stage in the environmental cycling of silver. n-Ag2S is much less labile than n-Ag. However, if it is processed in a WWTP under UV irradation or exposed to sunlight with photoactive ENMs like n-TiO2, n-Ag2S is not inert and has the potential to release dissolved silver. This silver released to the environment behaves in the same manner as that released from n-Ag and can cycle between the dissolved and nanoparticle forms, as has been demonstrated in sunlit environments.56
A conventional wisdom has evolved that the environmental transformations of ENMs, particularly aggregation and sulfidation, render them benign. Sulfidation of silver, in particular, has generally been described as an environmental process that detoxifies n-Ag. Yet, as we report here, sulfidation does not entirely eliminate the environmental toxicity of n-Ag, especially under light. In fact, due to their photochemical interactions, mixtures of n-Ag2S and n-TiO2 produce an enhanced microbial stress response under irradiation. Overall, this work provides additional evidence that the processing of multiple metallic nanomaterials together in complex environmental systems with light can lead to surprising changes in their chemical speciation and toxic effects.
Conflicts of interest
There are no conflicts to declare.
Acknowledgements
Metal analysis was performed at the Northwestern University Quantitative Bio-element Imaging Center and was funded by the Chicago Biomedical Consortium with support from the Searle Funds at The Chicago Community Trust and supported by the Office of The Director, National Institutes of Health of the National Institutes of Health under Award Number S10OD020118. Thanks to Keith MacRenaris and Rebecca Sponenburg for assistance with ICP-MS. This work made use of the EPIC facility of Northwestern University's NUANCE Center, which has received support from the Soft and Hybrid Nanotechnology Experimental (SHyNE) Resource (NSF ECCS-1542205); the MRSEC program (NSF DMR-1720139) at the Materials Research Center; the International Institute for Nanotechnology (IIN); the Keck Foundation; and the State of Illinois, through the IIN. Portions of this work were performed at the DuPont-Northwestern-Dow Collaborative Access Team (DND-CAT) located at Sector 5 of the Advanced Photon Source (APS). DND-CAT is supported by Northwestern University, E. I. DuPont de Nemours & Co., and The Dow Chemical Company. This research used resources of the Advanced Photon Source, a U.S. Department of Energy (DOE) Office of Science User Facility operated for the DOE Office of Science by Argonne National Laboratory under Contract No. DE-AC02-06CH11357. C. M. W. also acknowledges the support of the Northwestern University Presidential Fellowship.
References
- C. Levard, E. M. Hotze, B. P. Colman, A. L. Dale, L. Truong, X. Y. Yang, A. J. Bone, G. E. Brown, R. L. Tanguay, R. T. Di Giulio, E. S. Bernhardt, J. N. Meyer, M. R. Wiesner and G. V. Lowry, Sulfidation of Silver Nanoparticles: Natural Antidote to Their Toxicity, Environ. Sci. Technol., 2013, 47, 13440–13448 CrossRef CAS PubMed.
- H. T. Ratte, Bioaccumulation and toxicity of silver compounds: A review, Environ. Toxicol. Chem., 1999, 18, 89–108 CrossRef CAS.
- B. Reinsch, C. Levard, Z. Li, R. Ma, A. Wise, K. Gregory, G. Brown Jr and G. Lowry, Sulfidation of silver nanoparticles decreases Escherichia coli growth inhibition, Environ. Sci. Technol., 2012, 46, 6992–7000 CrossRef CAS PubMed.
- O. Choi, T. E. Clevenger, B. Deng, R. Y. Surampalli, L. Ross Jr and Z. Hu, Role of sulfide and ligand strength in controlling nanosilver toxicity, Water Res., 2009, 43, 1879–1886 CrossRef CAS PubMed.
- D. L. Starnes, J. M. Unrine, C. P. Starnes, B. E. Collin, E. K. Oostveen, R. Ma, G. V. Lowry, P. M. Bertsch and O. V. Tsyusko, Impact of sulfidation on the bioavailability and toxicity of silver nanoparticles to Caenorhabditis elegans, Environ. Pollut., 2015, 196, 239–246 CrossRef CAS PubMed.
- Z.-M. Xiu, J. Ma and P. J. J. Alvarez, Differential Effect of Common Ligands and Molecular Oxygen on Antimicrobial Activity of Silver Nanoparticles versus Silver Ions, Environ. Sci. Technol., 2011, 45, 9003–9008 CrossRef CAS PubMed.
- B. Kim, C.-S. Park, M. Murayama and M. F. Hochella, Discovery and Characterization of Silver Sulfide Nanoparticles in Final Sewage Sludge Products, Environ. Sci. Technol., 2010, 44, 7509–7514 CrossRef CAS PubMed.
- M. Baalousha, K. P. Arkill, I. Romer, R. E. Palmer and J. R. Lead, Transformations of citrate and Tween coated silver nanoparticles reacted with Na2S, Sci. Total Environ., 2015, 502, 344–353 CrossRef CAS PubMed.
- M. Azodi, Y. Sultan and S. Ghoshal, Dissolution Behavior of Silver Nanoparticles and Formation of Secondary Silver Nanoparticles in Municipal Wastewater by Single-Particle ICP-MS, Environ. Sci. Technol., 2016, 50, 13318–13327 CrossRef CAS PubMed.
- C. Levard, B. C. Reinsch, F. M. Michel, C. Oumahi, G. V. Lowry and G. E. Brown, Sulfidation Processes of PVP-Coated Silver Nanoparticles in Aqueous Solution: Impact on Dissolution Rate, Environ. Sci. Technol., 2011, 45, 5260–5266 CrossRef CAS PubMed.
- R. Ma, C. Levard, J. D. Judy, J. M. Unrine, M. Durenkamp, B. Martin, B. Jefferson and G. V. Lowry, Fate of Zinc Oxide and Silver Nanoparticles in a Pilot Wastewater Treatment Plant and in Processed Biosolids, Environ. Sci. Technol., 2014, 48, 104–112 CrossRef CAS PubMed.
- E. Lombi, E. Donner, S. Taheri, E. Tavakkoli, Å. K. Jämting, S. McClure, R. Naidu, B. W. Miller, K. G. Scheckel and K. Vasilev, Transformation of four silver/silver chloride nanoparticles during anaerobic treatment of wastewater and post-processing of sewage sludge, Environ. Pollut., 2013, 176, 193–197 CrossRef CAS PubMed.
- C. L. Doolette, M. J. McLaughlin, J. K. Kirby, D. J. Batstone, H. H. Harris, H. Ge and G. Cornelis, Transformation of PVP coated silver nanoparticles in a simulated wastewater treatment process and the effect on microbial communities, Chem. Cent. J., 2013, 7, 46 CrossRef PubMed.
- M. L. Nguyen, J. A. Murphy, L. C. Hamlet and B. L. T. Lau, Ligand-dependent Ag2S formation: changes in deposition of silver nanoparticles with sulfidation, Environ. Sci.: Nano, 2018, 5, 1090–1095 RSC.
- A. L. Dale, G. V. Lowry and E. A. Casman, Modeling Nanosilver Transformations in Freshwater Sediments, Environ. Sci. Technol., 2013, 47, 12920–12928 CrossRef CAS PubMed.
- R. Kaegi, A. Voegelin, C. Ort, B. Sinnet, B. Thalmann, J. Krismer, H. Hagendorfer, M. Elumelu and E. Mueller, Fate and transformation of silver nanoparticles in urban wastewater systems, Water Res., 2013, 47, 3866–3877 CrossRef CAS PubMed.
- J. Liu, K. G. Pennell and R. H. Hurt, Kinetics and Mechanisms of Nanosilver Oxysulfidation, Environ. Sci. Technol., 2011, 45, 7345–7353 CrossRef CAS PubMed.
- L. Li, Z. Xu, A. Wimmer, Q. Tian and X. Wang, New Insights into the Stability of Silver Sulfide Nanoparticles in Surface Water: Dissolution through Hypochlorite Oxidation, Environ. Sci. Technol., 2017, 51, 7920–7927 CrossRef CAS PubMed.
- B. Thalmann, A. Voegelin, U. von Gunten, R. Behra, E. Morgenroth and R. Kaegi, Effect of Ozone Treatment on Nano-Sized Silver Sulfide in Wastewater Effluent, Environ. Sci. Technol., 2015, 49, 10911–10919 CrossRef CAS PubMed.
- B. Collin, O. V. Tsyusko, D. L. Starnes and J. M. Unrine, Effect of natural organic matter on dissolution and toxicity of sulfidized silver nanoparticles to Caenorhabditis elegans, Environ. Sci.: Nano, 2016, 3, 728–736 RSC.
- L. Li, Y. Wang, Q. Liu and G. Jiang, Rethinking Stability of Silver Sulfide Nanoparticles (Ag2S-NPs) in the Aquatic Environment: Photoinduced Transformation of Ag2S-NPs in the Presence of Fe(III), Environ. Sci. Technol., 2016, 50, 188–196 CrossRef CAS PubMed.
- C. M. Wilke, J.-F. Gaillard and K. A. Gray, The critical role of light in moderating microbial stress due to mixtures of engineered nanomaterials, Environ. Sci.: Nano, 2018, 5, 96–102 RSC.
- L. Li, Q. Zhou, F. Geng, Y. Wang and G. Jiang, Formation of Nanosilver from Silver Sulfide Nanoparticles in Natural Waters by Photoinduced Fe(II, III) Redox Cycling, Environ. Sci. Technol., 2016, 50, 13342–13350 CrossRef CAS PubMed.
- C. M. Wilke, T. Tong, J.-F. Gaillard and K. A. Gray, Attenuation of Microbial Stress Due to Nano-Ag and Nano-TiO2 Interactions under Dark Conditions, Environ. Sci. Technol., 2016, 50, 11302–11310 CrossRef CAS PubMed.
- C. M. Wilke, B. Wunderlich, J.-F. Gaillard and K. A. Gray, Synergistic Bacterial Stress Results from Exposure to Nano-Ag and Nano-TiO2 Mixtures under Light in Environmental Media, Environ. Sci. Technol., 2018, 52, 3185–3194 CrossRef CAS PubMed.
- T. Tong, C. M. Wilke, J. Wu, C. T. T. Binh, J. J. Kelly, J.-F. Gaillard and K. A. Gray, Combined Toxicity of Nano-ZnO and Nano-TiO2: From Single- to Multinanomaterial Systems, Environ. Sci. Technol., 2015, 49, 8113–8123 CrossRef CAS PubMed.
- A. Pal, Y. He, M. Jekel, M. Reinhard and K. Y.-H. Gin, Emerging contaminants of public health significance as water quality indicator compounds in the urban water cycle, Environ. Int., 2014, 71, 46–62 CrossRef CAS PubMed.
- L. Li, M. Stoiber, A. Wimmer, Z. Xu, C. Lindenblatt, B. Helmreich and M. Schuster, To What Extent Can Full-Scale Wastewater Treatment Plant Effluent Influence the Occurrence of Silver-Based Nanoparticles in Surface Waters?, Environ. Sci. Technol., 2016, 50, 6327–6333 CrossRef CAS PubMed.
- B. Vriens, A. Voegelin, S. J. Hug, R. Kaegi, L. H. E. Winkel, A. M. Buser and M. Berg, Quantification of Element Fluxes in Wastewaters: A Nationwide Survey in Switzerland, Environ. Sci. Technol., 2017, 51, 10943–10953 CrossRef CAS PubMed.
- R. D. Kent, J. G. Oser and P. J. Vikesland, Controlled Evaluation of Silver Nanoparticle Sulfidation in a Full-Scale Wastewater Treatment Plant, Environ. Sci. Technol., 2014, 48, 8564–8572 CrossRef CAS PubMed.
- B. Kim, M. Murayama, B. P. Colman and M. F. Hochella, Characterization and environmental implications of nano- and larger TiO2 particles in sewage sludge, and soils amended with
sewage sludge, J. Environ. Monit., 2012, 14, 1128–1136 CAS.
- M. A. Kiser, P. Westerhoff, T. Benn, Y. Wang, J. Pérez-Rivera and K. Hristovski, Titanium Nanomaterial Removal and Release from Wastewater Treatment Plants, Environ. Sci. Technol., 2009, 43, 6757–6763 CrossRef CAS PubMed.
- P. Westerhoff, G. Song, K. Hristovski and M. A. Kiser, Occurrence and removal of titanium at full scale wastewater treatment plants: implications for TiO2 nanomaterials, J. Environ. Monit., 2011, 13, 1195–1203 RSC.
- H. Jia, W. He, W. G. Wamer, X. Han, B. Zhang, S. Zhang, Z. Zheng, Y. Xiang and J.-J. Yin, Generation of Reactive Oxygen Species, Electrons/Holes, and Photocatalytic Degradation of Rhodamine B by Photoexcited CdS and Ag2S Micro-Nano Structures, J. Phys. Chem. C, 2014, 118, 21447–21456 CrossRef CAS.
- G. Li and K. A. Gray, The solid–solid interface: Explaining the high and unique photocatalytic reactivity of TiO2-based nanocomposite materials, Chem. Phys., 2007, 339, 173–187 CrossRef CAS.
-
American Public Health Association, Standard methods for the examination of water and wastewater, Washington, D.C., 15th edn, 1980 Search PubMed.
- T. Tong, C. T. T. Binh, J. J. Kelly, J.-F. Gaillard and K. A. Gray, Cytotoxicity of commercial nano-TiO2 to Escherichia coli assessed by high-throughput screening: Effects of environmental factors, Water Res., 2013, 47, 2352–2362 CrossRef CAS PubMed.
- T. Tong, K. Fang, S. A. Thomas, J. J. Kelly, K. A. Gray and J.-F. Gaillard, Chemical Interactions between Nano-ZnO and Nano-TiO2 in a Natural Aqueous Medium, Environ. Sci. Technol., 2014, 48, 7924–7932 CrossRef CAS PubMed.
-
M. Newville, Larch: An Analysis Package For XAFS And Related Spectroscopies, Journal of Physics: Conference Series, 2013, vol. 430, p. 012007 Search PubMed.
-
J.-F. Gaillard, Probing Environmental Particles and Colloids with X-Rays, in Environmental Particles and Colloids: Behaviour, Structure, and Characterisation, ed. K. J. Wilkinson and J. R. Lead, IUPAC Series on Analytical and Physical Chemistry of Environmental Systems, Wiley, Chichester, 2007, pp. 613–666 Search PubMed.
- J. Schindelin, I. Arganda-Carreras, E. Frise, V. Kaynig, M. Longair, T. Pietzsch, S. Preibisch, C. Rueden, S. Saalfeld and B. Schmid, Fiji: an open-source platform for biological-image analysis, Nat. Methods, 2012, 9, 676 CrossRef CAS PubMed.
- E. Pick and Y. Keisari, A simple colorimetric method for the measurement of hydrogen peroxide produced by cells in culture, J. Immunol. Methods, 1980, 38, 161–170 CrossRef CAS PubMed.
- W. Zhang, Y. Li, J. Niu and Y. Chen, Photogeneration of Reactive Oxygen Species on Uncoated Silver, Gold, Nickel, and Silicon Nanoparticles and Their Antibacterial Effects, Langmuir, 2013, 29, 4647–4651 CrossRef CAS PubMed.
- S. Link, Z. L. Wang and M. A. El-Sayed, Alloy Formation of Gold−Silver Nanoparticles and the Dependence of the Plasmon Absorption on Their Composition, J. Phys. Chem. B, 1999, 103, 3529–3533 CrossRef CAS.
- S. Kapoor, Preparation, Characterization, and Surface Modification of Silver Particles, Langmuir, 1998, 14, 1021–1025 CrossRef CAS.
- S. Bhattacharjee, DLS and zeta potential – What they are and what they are not?, J. Controlled Release, 2016, 235, 337–351 CrossRef CAS PubMed.
- Y. Ono, T. Matsumura, N. Kitajima and S. Fukuzumi, Formation of superoxide ion during the decomposition of hydrogen peroxide on supported metals, J. Phys. Chem., 1977, 81, 1307–1311 CrossRef CAS.
- C.-H. Liao and M. D. Gurol, Chemical Oxidation by Photolytic Decomposition of Hydrogen Peroxide, Environ. Sci. Technol., 1995, 29, 3007–3014 CrossRef CAS PubMed.
- C. Lu, G. Song and J.-M. Lin, Reactive oxygen species and their chemiluminescence-detection methods, TrAC, Trends Anal. Chem., 2006, 25, 985–995 CrossRef CAS.
- Y. Li, W. Zhang, J. Niu and Y. Chen, Surface-Coating-Dependent Dissolution, Aggregation, and Reactive Oxygen Species (ROS) Generation of
Silver Nanoparticles under Different Irradiation Conditions, Environ. Sci. Technol., 2013, 47, 10293–10301 CAS.
- D. He, S. Garg and T. D. Waite, H2O2-Mediated Oxidation of Zero-Valent Silver and Resultant Interactions among Silver Nanoparticles, Silver Ions, and Reactive Oxygen Species, Langmuir, 2012, 28, 10266–10275 CrossRef CAS PubMed.
- W. He, Y.-T. Zhou, W. G. Wamer, M. D. Boudreau and J.-J. Yin, Mechanisms of the pH dependent generation of hydroxyl radicals and oxygen induced by Ag nanoparticles, Biomaterials, 2012, 33, 7547–7555 CrossRef CAS PubMed.
- M. M. Shafer, J. T. Overdier and D. E. Armstong, Removal, partitioning, and fate of silver and other metals in wastewater treatment plants and effluent-receiving streams, Environ. Toxicol. Chem., 1998, 17, 630–641 CrossRef CAS.
- T. Tong, A. N. Hill, M. A. Alsina, J. Wu, K. Y. Shang, J. J. Kelly, K. A. Gray and J.-F. Gaillard, Spectroscopic Characterization of TiO2 Polymorphs in Wastewater Treatment and Sediment Samples, Environ. Sci. Technol. Lett., 2015, 2, 12–18 CrossRef CAS.
- A. D. Dotson, C. E. Rodriguez and K. G. Linden, UV disinfection implementation status in US water treatment plants, J. - Am. Water Works Assoc., 2012, 104, E318–E324 CrossRef.
- W.-C. Hou, B. Stuart, R. Howes and R. G. Zepp, Sunlight-Driven Reduction of Silver Ions by Natural Organic Matter: Formation and Transformation of Silver Nanoparticles, Environ. Sci. Technol., 2013, 47, 7713–7721 CrossRef CAS PubMed.
Footnotes |
† Electronic supplementary information (ESI) available. See DOI: 10.1039/c8en01159a |
‡ Current address: Department of Construction Engineering and Management, University of Talca, Camino Los Niches Km.1, Curicó, Región del Maule, Chile. |
|
This journal is © The Royal Society of Chemistry 2019 |
Click here to see how this site uses Cookies. View our privacy policy here.