Cu-exchanged RTH-type zeolites for NH3-selective catalytic reduction of NOx: Cu distribution and hydrothermal stability†
Received
17th September 2018
, Accepted 13th November 2018
First published on 13th November 2018
Abstract
The Cu-exchanged RTH-type zeolites (Cu-RTH) were applied in ammonia-selective catalytic reduction (NH3-SCR) of NOx. The effects of Cu loading and distribution on the NOx reduction efficiency were investigated. The hydrothermal stabilities of a series of Cu-RTH catalysts were also presented. Excellent NOx conversion activity was observed for Cu-RTH catalysts with high Cu loadings (2.5–4.4 wt%) in the temperature range from 150 °C to 550 °C. Two possible sites (α and β species) for Cu2+ species were proposed based on the analysis of H2-TPR and DRIFTS results. The α species next to the 8-membered rings showed significantly higher TOF (turnover frequency) of NO conversion than the β species present next to the rth cage. The Cu-RTH catalysts showed lower activation energies (30–40 kJ mol−1) compared with that of the Cu-SSZ-13 catalyst (∼54 kJ mol−1) due to the different zeolite framework structures. After undergoing hydrothermal aging, although the zeolite framework structure stayed stable, the active Cu2+ species migrated to inactive sites, which resulted in the loss of NOx conversion for the NH3-SCR reaction. The saturated Cu2+-exchanged Cu4.4-RTH catalyst showed the best hydrothermal stability due to the limited Cu2+ mobility, which stabilized the active Cu2+ species.
1. Introduction
Currently, the removal of NOx from diesel engine exhaust, an extremely important environmental issue, faces enormous challenges. Zeolite-based selective catalytic reduction of NOx with NH3 (NH3-SCR) is an efficient and widely implemented technique for the abatement of NOx due to the high NOx conversion and hydrothermal stability.1–7 Since Cu-SSZ-13, a Cu ion-exchanged zeolite with a small-pore chabazite (CHA) framework, was found to have outstanding activity and hydrothermal stability for the reduction of NOx in NH3-SCR, researchers have focused considerably on small-pore metal-exchanged zeolites.8–15
During their actual application, the presence of H2O in the exhaust from diesel particulate filter (DPF) regeneration always damaged the CHA zeolite framework structure by causing the dealumination process. The high hydrothermal stability of small-pore zeolite catalysts is attributed to their specific structures since the constricting dimensions of the special small pores limits the detachment of aluminum hydroxide.16 Based on this advantage, researchers have focused on many other types of small-pore zeolites, such as KFI,10 LTA,9 AEI,12 RTH,17 AFX,18 and ERI,18 which have also shown NOx reduction conversion or hydrothermal stability comparable to Cu-SSZ-13 catalysts in the NH3-SCR reaction.
Xu et al.19 recently reported an efficient and rapid method of synthesizing small-pore aluminosilicate RTH zeolite, which cansignificantly shorten the crystallization time (50 min at 240 °C), which is beneficial to its practical application as an NH3-SCR catalyst. Being different from other zeolites (KFI, LTA, AEI, AFX, and ERI) with three-dimensional channels, the RTH-type zeolite has two-dimensional channels with aperture sizes of 0.41 nm × 0.38 nm (8MRs) and 0.56 nm × 0.25 nm (distorted 8MRs), as shown in Scheme 1. The structure of RTH was constructed with a small cage (rth) and a large cage (ite). Therefore, the Cu-exchanged RTH zeolite (Cu-RTH) was expected to have high catalytic properties in the NH3-SCR reaction due to the 8MR pore channel and its cages. To our knowledge, the studies on Cu-RTH zeolite catalysts used in NH3-SCR applications have been few. Albarracin-Caballero et al.17 and Jo et al.20 used Cu-RTH zeolites for comparison with SSZ-13 zeolite and other catalysts. However, each literature compared a catalyst with only one Cu-loading value (0.7 wt% and 3.3 wt%, respectively), and limited characterizations were performed on these catalysts. The zeolite framework structure, Cu2+ species distribution, catalytic properties, and hydrothermal stability of Cu-RTH catalysts should be further studied for their dependence on different Cu loadings.
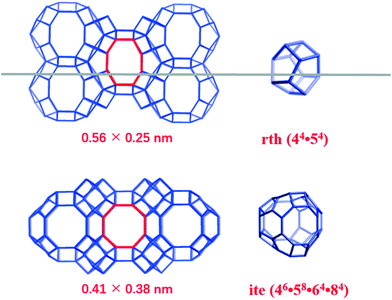 |
| Scheme 1 Two types of 8MR pores and cage structures in the RTH-type zeolite. rth cage consists of 4 four-membered rings and 4 five-membered rings; ite cage consists of 6 four-membered rings, 8 five-membered rings, 4 six-membered rings, and 4 eight-membered rings. | |
Herein, we systematically investigated Cu-RTH catalysts with different Cu loadings before and after hydrothermal aging. The Cu2+ species location and distribution as well as their catalytic properties were studied using H2-TPR, in situ NH3 absorption DRIFTS measurements, and kinetic studies. Additionally, changes in the Cu2+ species and the framework structure after hydrothermal aging were investigated for the Cu-RTH catalysts with different Cu loadings through XRD, 27Al-NMR, and XAFS. We expect to have a better understanding of the structure–activity relationship and optimize the steps for rationally designing Cu-exchanged RTH zeolite catalysts with high activity and hydrothermal stability.
2. Experimental section
2.1. Catalyst preparation
The parent RTH zeolite employed in this study was synthesized following the method reported by Xu et al.19 The obtained Na-RTH zeolite was ion-exchanged with 1 M NH4NO3 solution at 80 °C for 8 h to produce NH4-RTH zeolite. Varying Cu loadings of the Cu-form RTH zeolite were achieved by ion-exchange between NH4-RTH zeolite and various concentrations of Cu(CHCOO)2 solution (0.05–1.0 M). After the ion-exchange, the catalysts were filtered, washed, and dried in air overnight prior to calcination at 600 °C for 6 h.
For comparison, the Cu-SSZ-13 catalyst was synthesized using a one-pot method.21 The initial Cu-SSZ-13 catalyst was ion-exchanged with HNO3 solution (pH = 1). After the ion-exchange, the catalyst was filtered, washed and dried in air overnight prior to calcination at 600 °C for 6 h. The Cu loading and Cu/Al and Si/Al ratios of Cu-RTH catalysts are listed in Table 1.
Table 1 Elemental analysis and micropore areas and volumes of Cu-RTH catalysts
Samples |
Cu contents (wt%) |
Na contents (wt%) |
Cu/Ala |
Si/Ala |
Micropore area (m2 g−1)b |
Micropore volume (cm3 g−1)b |
Micropore area (m2 g−1)c |
Micropore area (m2 g−1)c |
Elements were determined by ICP-AES.
For fresh catalysts, calculated by the t-plot method.
For HTA catalysts, calculated by the t-plot method.
|
Cu1.0-RTH |
0.99 |
0.09 |
0.10 |
8.2 |
519 |
0.20 |
— |
— |
Cu1.4-RTH |
1.37 |
0.09 |
0.15 |
8.5 |
528 |
0.21 |
— |
— |
Cu2.5-RTH |
2.52 |
0.06 |
0.27 |
8.0 |
547 |
0.21 |
510 |
0.21 |
Cu3.5-RTH |
3.46 |
0.06 |
0.40 |
8.2 |
542 |
0.21 |
518 |
0.21 |
Cu4.4-RTH |
4.41 |
0.05 |
0.52 |
8.2 |
319 |
0.16 |
320 |
0.15 |
2.2. Characterization
Powder X-ray diffraction (PXRD) patterns were collected on a computerized Bruker D8 Advance diffractometer with Cu Kα (λ = 0.15406 nm) radiation. The 2θ data from 5° to 40° were collected with a step size of 0.02°. All measurements were performed at ambient temperature. Elemental analyses of the samples (Cu contents, Cu/Al, and Si/Al ratio) were obtained using an inductively coupled plasma instrument (OPTMIA 2000DV) with a radial view of the plasma. The samples were dissolved in strong acid prior to analysis. N2 adsorption/desorption isotherms of the Cu-RTH samples were measured at 77 K using a Micromeritics ASAP 2020 system. Micropore surface areas and micropore volumes were obtained by the t-plot method. Before the measurements, the samples were treated at 300 °C for 5 h. Solid state 27Al and 29Si MAS NMR spectra were collected on a Bruker AVANCE III 400 WB spectrometer equipped with a 4 mm standard bore CP MAS probe head whose X channel was tuned to 156 MHz and 119 MHz for 27Al and 29Si, respectively. The dried and finely powdered samples were packed in the ZrO2 rotor, closed with a Kel-F cap and spun at 12 kHz. A total of 1000 scans were recorded with 5 s recycle delay for each sample. All 27Al and 29Si MAS NMR chemical shifts were referenced to the resonances of aluminum oxide (Al2O3) and TMA standard, respectively.
Temperature-programmed reduction with hydrogen (H2-TPR) experiments were performed on a Micromeritics AutoChem 2920 chemisorption analyzer. The samples (∼50 mg) were loaded into a quartz reactor and exposed to a 10 vol% H2/Ar gas flow at 50 mL min−1 after being treated in air at 500 °C for 60 min. Hydrogen consumption was detected by a thermal conductivity detector (TCD), with temperature ramping linearly from ambient temperature to 1000 °C at a rate of 10 °C min−1. The in situ diffuse reflectance infrared Fourier transform spectroscopy experiments using molecular NH3 as the probe (in situ NH3-DRIFTS) were conducted using a Nicolet Is10 instrument equipped with a Smart Collector and an MCT/A detector. Before collecting the spectra, the samples were heated to 500 °C for 1 h in 20% O2/N2,and then cooled to 35 °C. Subsequently, the background was collected in pure N2 and automatically subtracted from the sample spectrum. Then, the sample was exposed to the probe molecule NH3 until the spectra were unchanged, and then swept out with N2. All spectra were recorded from 4000 cm−1 to 650 cm−1 by accumulating 100 scans with a resolution of 4 cm−1.
X-ray absorption fine structure (XAFS) experiments were performed on the BL14W1 beamline of the Shanghai Synchrotron Radiation Facility (SSRF). Using a Si (111) monochromator, the absorption data from −200 eV to 800 eV of the Cu K-edge (8979 eV) was collected at ambient temperature in transmission mode. CuO and Cu2O were used as reference compounds. X-ray absorption near-edge structure (XANES) data were background-corrected and normalized using the Athena module implemented in the IFFEFIT software package.22 The extended X-ray absorption fine structure (EXAFS) data and FT of the k2-weighed data were analyzed and fit using Athena and Artemis (k range of 3–12 Å−1). The amplitude reduction factor (S02) of 0.9 was used for all fitted data sets.
2.3. Catalytic activity test
Standard selective catalytic reduction (SCR) was performed in a fixed-bed quartz flow reactor at atmospheric pressure. A resistance furnace was used to simulate different reaction temperatures. The reactive gas contained 500 ppm NO, 500 ppm NH3, 5% O2, 5% H2O, balance N2, and the total flow rate was 500 mL min−1. The catalysts were pelletized and sieved to 40–60 mesh size and ∼180 mg of catalyst was used, which resulted in a gas hourly space velocity (GHSV) of ∼100
000 h−1. An online Nicolet Is10 spectrometer equipped with a heated low volume (0.2 L) multiple-path gas cell (2 m) was used to continuously analyze the effluent gases, including NO, NH3, NO2, and N2O. When the test reaction was in a steady state, FTIR spectra were recorded throughout. NOx conversion was calculated according to the following equation:
Kinetic studies were also conducted on this measurement system. The reaction rates (r), activation energies (Ea), and turnover frequencies (TOF) were calculated based on the following equation:
where
W is the catalyst weight (g),
F is the NO flow rate (mol s
−1),
x is the NO conversion, [NO]
0 is the initial concentration of NO, and
M is the weight percent of Cu in the sample. For collecting the hydrothermal stability data, the tested samples were hydrothermally aged (HTA) at 750 °C for 16 h in a 10 vol% H
2O/air feed.
3. Results and discussion
3.1. Standard NH3-SCR performance and hydrothermal stability
The standard NH3-SCR activities of Cu-RTH catalysts with varying Cu loading are shown in Fig. 1. It can be seen that Cu1.0-RTH and Cu1.4-RTH catalysts with low Cu loading exhibited low NOx conversion at low temperatures (<400 °C) On increasing the Cu loading to 2.5 wt%, a significant increase in NOx conversion was realized at low temperatures. Further increasing the Cu loading had limited effect on the low temperature activity, but a decrease in NOx conversion at high temperatures (>450 °C) was observed for these catalysts, probably due to the facile non-selective NH3 oxidation.23 Simultaneously, nearly 100% N2 selectivity was achieved due to the low N2O production (less than 15 ppm) at all the tested temperatures (150–550 °C) for all the catalysts (Fig. S1†).
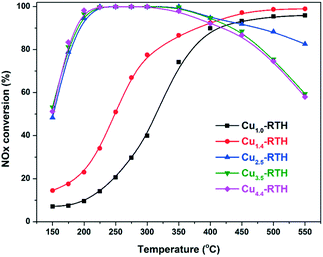 |
| Fig. 1 NOx conversion of Cu-RTH catalysts with different Cu loadings in the NH3-SCR reaction. Conditions: 500 ppm of NO, 500 ppm of NH3, 5% O2, 5% H2O, and balanced with N2. GHSV = 100 000 h−1. | |
Kinetic studies of the NH3-SCR reaction were performed over the Cu-RTH catalysts with varying Cu loading. Fig. S2† presents the NOx conversion in a standard NH3-SCR reaction under rigorous gas hourly space velocity (GHSV) conditions to obtain relatively low NOx conversion (<20%), which was used to calculate the reaction rates and activation energy (Ea) in the standard SCR reaction. Fig. 2 shows the Arrhenius plots of Cu-RTH with different Cu loadings. It was found that the Cu-RTH catalysts with Cu loadings from 1.0 wt% to 4.4 wt% showed lower activation energies (35–39 kJ mol−1) compared with that of our as-prepared Cu-SSZ-13 catalyst (54 kJ mol−1, Fig. S3†) and other reported Cu-SSZ-13 catalysts.24 This decrease in activation energy was attributed to the different molecular sieve framework structures of Cu-RTHs, in which the dynamic [Cu(NH3)2]+ ions serve as active sites.25–27
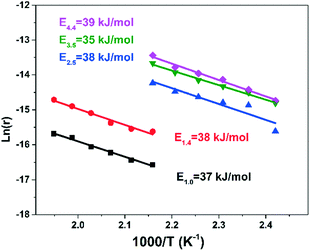 |
| Fig. 2 Arrhenius plots of NOx conversion over Cu-RTH catalysts with different Cu loadings. Normalized reaction rates were calculated using NOx conversion data (<20%) in high GHSVs, shown in Fig. S2.† | |
Generally, the hydrothermal stability of NH3-SCR catalysts should be improved to meet the requirements for high durability toward moisture at high temperatures for future diesel after-treatment systems.28,29 In this work, Cu-RTH catalysts with relatively high Cu loadings (2.5 wt%, 3.5 wt%, and 4.4 wt%) were tested after hydrothermal aging because of their high NOx conversion activities. As shown in Fig. 3, the hydrothermally aged samples showed a sharp decrease in NOx conversion, indicating severe damage after hydrothermal aging at 750 °C for the Cu-RTH zeolite catalysts. Among the three catalysts, Cu4.4-RTH-HTA catalyst exhibited higher NOx conversion than the other two catalysts. In addition, the NOx conversion after hydrothermal aging increased with an increase in Cu loading, although fresh catalysts had similar NOx conversion, particularly at low temperatures. Fig. S4† shows that N2O formation was still less than 15 ppm for the hydrothermally aged catalysts, indicating excellent N2 selectivity.
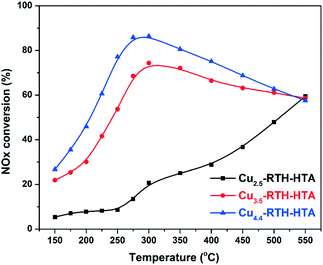 |
| Fig. 3 NOx conversion of hydrothermally aged Cu-RTH catalysts with different Cu loadings during the NH3-SCR reaction. Conditions: 500 ppm NO, 500 ppm NH3, 5% O2, 5% H2O, and balanced with N2. GHSV = 100 000 h−1. Hydrothermal aging conditions: 750 °C, 10% H2O, and for 16 h. | |
3.2. Characterization of Cu2+ species of Cu-RTH catalysts
X-ray absorption spectroscopy (XAS) is an effective technique to determine the local coordination and valence state of Cu species in Cu-based zeolites. Fig. 4 depicts the Cu K-edge XANES spectra and the associated Fourier transforms of EXAFS spectra of the fresh and hydrothermally aged Cu-RTH catalysts measured at ambient temperatures. In addition to ordinary reference samples such as Cu2O and CuO, Cu-SSZ-13 (Cu: 4.8 wt%, Cu/Al = 0.41) was also used as a reference to compare the Cu coordination and valence states in the two types of zeolite catalysts, as shown in Fig. S5.† For the fresh samples, a pre-edge feature at 8977.5 eV, which was attributed to the 1s → 3d transition of Cu ions, was characteristic of the presence of Cu(II) (Fig. 4a, magnified profiles).30 For a series of Cu-RTH catalysts with different Cu loading, this pre-edge feature was unchanged, suggesting that the coordination environment of Cu(II) ions was similar in different samples with varying Cu loadings. Additionally, another weak pre-edge peak was observed at 8983 eV for the Cu-RTH samples, which was assigned to the 2-coordinate isolated Cu(I).31 It can be seen that the Cu-SSZ-13 catalysts also exhibited a weak peak at 8983 eV (Fig. S4a†). The presence of this feature meant that “self-reduction” of Cu2+ species occurred during the process of cooling after calcination. In addition, higher intensities of the pre-feature for Cu-RTH catalysts indicated that more Cu2+ ions were converted to Cu+ than in Cu-SSZ-13 at ambient conditions, suggesting the instability of Cu2+ ions in Cu-RTH catalysts. A slight feature at 8986 eV, which was ascribed to the 1s → 4pz electronic transition on 4-coordinate Cu2+ (CuO),31–33 was only observed for the Cu4.4-RTH catalyst with highest Cu loading, indicating that high level of Cu2+ ion exchange resulted in the formation of a small amount of CuO. The R-space plot of EXAFS data (Fig. 4b) also showed a Cu–Cu bond in the Cu4.4-RTH catalyst, while no such Cu–Cu bond was observed for the other fresh catalysts. The broad feature on the absorption edge at 8989 eV was assigned to dehydrated Cu2+ species coordinated with the zeolite framework and the hydrated Cu(II)-aqua complex.13,32,34 The parameters resulting from the experimental data and the simulated fittings of EXAFS spectra are listed in Table S1.† There was almost no difference in the CN (∼3.6) and Cu–O bond distance (∼1.95 Å) between Cu-RTH and Cu-SSZ-13 catalysts, which indicated that the coordination environments of most Cu species in Cu-RTH are similar to those in Cu-SSZ-13 catalysts.
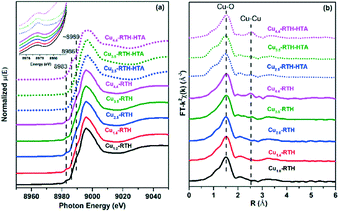 |
| Fig. 4 (a) Cu K-edge XANES spectra and (b) associated Fourier transforms of EXAFS spectra of fresh and hydrothermally aged Cu-RTH catalysts with varying Cu loadings. | |
Among the hydrothermally aged Cu-RTH catalysts, Cu4.4-RTH-HTA showed an increase in the feature peak at 8986 eV, indicating CuOx accumulation during the hydrothermal aging process. However, the feature peak belonging to CuOx cannot be seen in the XANES spectra for Cu2.5 and Cu3.5-RTH-HTA catalysts, suggesting no accumulation of CuOx for Cu2.5 and Cu3.5-RTH catalysts after hydrothermal aging. The R-space plot of EXAFS data also showed an increased Cu–Cu bond peak intensity in the hydrothermally aged Cu4.4-RTH catalyst, while there was no Cu–Cu bond for the other two hydrothermally aged catalysts (Fig. 4b). There was also no significant difference between the fresh and hydrothermally aged Cu-RTH catalysts in terms of the CN (coordination number) and Cu–O distances (Table S1†), indicating that Cu2+ in the hydrothermally aged Cu-RTH catalysts was still the dominant form of Cu. Interestingly, the Cu4.4-RTH catalyst showed the best hydrothermal stability even though CuO was formed during hydrothermal aging, whereas severe deactivation was observed for the hydrothermally aged Cu2.5 and Cu3.5-RTH catalysts without CuOx formation. This phenomenon demonstrated that CuOx formation was not the main reason for the deactivation of hydrothermally aged Cu2.5 and Cu3.5-RTH catalysts. Therefore, another reason responsible for the deactivation of these hydrothermal aged Cu-RTH catalysts will be discussed in the next section.
H2-TPR measurement is an efficient technique to investigate the reducibility and distribution of Cu2+ species in Cu-based zeolite catalysts. In H2-TPR profiles (Fig. 5), the peaks at temperatures lower than 500 °C are attributed to the reduction of Cu2+ to Cu+, and the peaks at temperatures higher than 500 °C are attributed to the reduction of Cu+ to Cu0 based on previously published studies.35–37 As shown in Fig. 5a, the H2-TPR reduction peak of the fresh Cu-RTH catalysts shows a similar change trend to that of Cu-SSZ-13 with the increase in Cu loading.36,38 A single reduction peak at around 360 °C was observed for the Cu-RTH catalysts with low Cu loadings (<1.4 wt%), while with increase in Cu loading, another reduction peak appeared at 200 °C. Notably, the intensity of this reduction peak at 200 °C increased when the Cu loading increased from 2.5 wt% to 4.4 wt%, while the intensity of the reduction peak at 360 °C remained almost constant, demonstrating that Cu2+ ions preferentially occupied stable ion-exchange sites until saturation; also, another relatively unstable ion-exchange site (peak at 200 °C) was occupied by additional Cu2+ ions. The calculation of H2 consumption is depicted in Fig. S6.† A positive linear relationship between H2 consumption and Cu loading was observed, which indicated the uniformity of Cu species. It should be noted that a slight amount of CuO was observed in Cu4.4-RTH, according to the XAFS results (Fig. 4). The reduction of CuO should exhibit a consumption peak at around 200–300 °C.3,38,39 It seemed that either there was no distinct CuO reduction peak or the reduction peak might be merged in the peak at 200 °C. Regardless, the amount of CuO for the Cu4.4-RTH catalyst was low, as can be seen from the lack of deviation of Cu4.4-RTH from the linear relation between H2 consumption and Cu loading (Fig. S5†) for the Cu-RTH catalysts. Moreover, a wide peak appeared at ∼825 °C, attributed to the presence of a variety of Cu+ species, which would cause the H2 consumption peak to broaden.
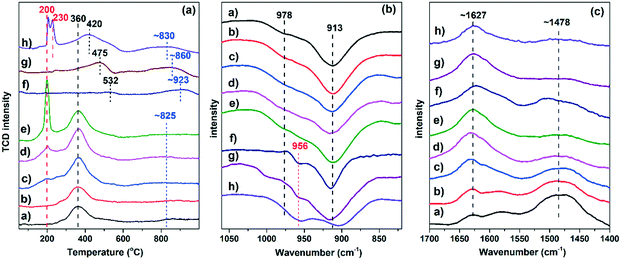 |
| Fig. 5 (a) H2-TPR profiles, (b) in situ DRIFTS spectra of T–O–T vibration regions and (c) acid vibration regions after NH3 adsorption at 35 °C on fresh and hydrothermally aged Cu-RTH catalysts. a) Cu1.0-RTH, b) Cu1.4-RTH, c) Cu2.5-RTH, d) Cu3.5-RTH, e) Cu4.4-RTH, f) Cu2.5-RTH-HTA, g) Cu3.5-RTH-HTA, and h) Cu4.4-RTH-HTA. | |
After hydrothermal aging at 750 °C, a significant change in the reduction peaks was observed for the Cu-RTH catalysts. The facile reduction peak at 200 °C disappeared for Cu2.5-RTH and Cu3.5-RTH catalysts after hydrothermal aging. However, the high-temperature H2 reduction peaks at 532 °C and 475 °C, which were higher than the H2 reduction peak for fresh samples, were observed for hydrothermally aged Cu2.5-RTH and Cu3.5-RTH catalysts, respectively. Moreover, for the Cu4.4-RTH-HTA catalyst, it was observed that the peak at 200 °C splited into two peaks, which were attributed to the original Cu2+ species and CuOx clusters. This CuOx formation was identified in the XANES and EXAFS results. Furthermore, the peak at 360 °C of the Cu4.4-RTH catalyst also shifted to higher temperature (∼420 °C) after hydrothermal aging, similar to the trend observed for Cu2.5-RTH and Cu3.5-RTH catalysts. The peak for the H2 reduction of Cu+ to Cu0 also shifted to higher temperatures for all Cu-RTH catalysts, indicating that the Cu2+ species migrated to more stable sites, where Cu+ ions were less reducible by H2. Furthermore, the lower H2 consumption of hydrothermally aged samples, particularly the Cu2.5-RTH-HTA catalyst, indicated that Cu species could not be completely reduced. These unreduced Cu2+ species, which were probably the stable Cu–aluminum–oxygen complexes,3,17 lost their catalytic ability of deNOx.
In situ DRIFTS using NH3 as the adsorbed probe molecule was also conducted to identify the Cu2+ locations. Fig. 5b shows the IR spectra in the zeolite T–O–T bond vibration region after NH3 was adsorbed onto the Cu-RTH catalysts. Two negative peaks at 978 and 913 cm−1 represent the zeolite T–O–T vibration perturbed by Cu2+ at different locations because NH3-solvated Cu2+ ions weaken the interaction between Cu2+ ions and the zeolite framework.40,41 From the IR spectra after NH3 adsorption, it can be seen that the peak intensity at 978 cm−1 enhanced, while the peak intensity at 913 cm−1 remained stable after saturation of Cu loading of 2.5 wt%. These two resolved peaks in the IR spectra coincide well with the two peaks observed in the H2-TPR results. Therefore, the DRIFTS experiment using NH3 as a probe molecule indicated two Cu exchange sites, and the peaks at 978 and 913 cm−1 can be possibly attributed to the T–O–T vibration perturbed by the Cu2+ species that were reduced at 200 °C and 360 °C, respectively, during the H2-TPR measurement. For the hydrothermally aged Cu-RTH catalysts, the peak at 978 cm−1, related to Cu2+ reduced by H2 at 200 °C in the H2-TPR results, disappeared and was accompanied by a new peak appearing at 956 cm−1, which was ascribed to a new stable Cu2+ site, probably attributed to the Cu–aluminum–oxygen complexes.17 After hydrothermal aging, the peak at 913 cm−1 changed only slightly, except for the decay observed for Cu4.4-RTH due to the formation of CuOx.
As shown in Fig. 5c, in the N–H bending region, the bands at 1627 cm−1 and 1478 cm−1 were assigned to molecular NH3 and NH4+ ion adsorbed on the Lewis acid sites and Brønsted acid sites, respectively.40,42,43 With additional Cu loading, it was observed that the Lewis acid sites, which stem from Cu2+ ions, increased accompanied by the decrease in Brønsted acid sites, indicating that Brønsted acid sites were replaced by Cu2+ during the ion exchange process. After hydrothermal aging, the Lewis acid sites of the Cu2.5-RTH and Cu3.5-RTH catalysts remained almost unchanged, indicating that the Cu2+ species still exist even after hydrothermal aging. This result agrees well with the XANES results, where CuOx was not observed for the hydrothermally aged Cu2.5-RTH and Cu3.5-RTH catalysts. The decrease in the number of Brønsted acid sites after hydrothermal aging resulted from the dealumination process during hydrothermal aging, which was also observed in the 27Al-NMR measurements (Fig. 7). For the Cu4.4-RTH catalyst, the formation of CuOx identified in XANES (Fig. 4) caused a decrease in the intensity of the peak at 1627 cm−1 after hydrothermal aging.
3.3. Characterization of the zeolite structure
The micropore areas and volumes of Cu-RTH zeolite catalysts with different Cu loadings are listed in Table 1. Except for Cu4.4-RTH, the samples showed micropore areas of 519–547 m2 g−1, with micropore volumes of 0.20–0.21 cm3 g−1. The lower micropore area and volume of Cu4.4-RTH was attributed to pore blocking due to the large amount of copper loading. All Cu-RTH catalysts showed the typical isotherms for microporous materials, as shown in Fig. S7.† After hydrothermal aging, there was no distinct change in the micropore areas or volumes. The typical isotherms of microporous materials were still maintained for hydrothermally aged Cu-RTH catalysts. SEM images (Fig. S8†) of Cu-RTH catalysts also showed no distinct change in morphological features after hydrothermal aging.
XRD patterns of the Cu-RTH samples are exhibited in Fig. 6. Typical diffraction peaks of the RTH zeolite structure were observed for the Cu-RTH catalysts of different Cu loadings with no distinct differences between the samples, indicating the presence of a stable RTH structure after ion exchange. Moreover, the XRD patterns of Cu-RTH catalysts showed no CuO peaks except for that of Cu4.4-RTH, with a minor CuOx peak (magnified XRD profiles in Fig. 6), which indicated well-dispersed Cu species. The formation of CuO for Cu4.4-RTH was due to supersaturation during ion exchange (Cu/Al = 0.52, 104% ion exchange level), probably resulting in more than one Cu2+ ions exchanged to one Al site. The hydrothermally aged Cu-RTH catalysts were also compared. After undergoing hydrothermal aging, although there was a slight decrease in the XRD peak intensity, the XRD patterns of Cu-RTH catalysts showed typical RTH diffraction peaks, indicating that the long-range structural order was maintained. Except for the presence of CuOx in the Cu4.4-RTH-HTA catalyst, there were no typical CuOx peaks observed for the hydrothermally aged Cu2.5-RTH and Cu3.5-RTH catalysts.
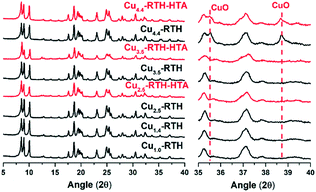 |
| Fig. 6 XRD patterns (left: 2θ = 5–40°) and magnified patterns (right: 2θ = 35–40°) of the Cu-RTH catalysts with different Cu loadings before and after hydrothermal aging. | |
Next, the local Al and Si coordination was probed by solid state 27Al and 29Si-NMR measurements (Fig. 7). As shown in Fig. 7a, all Cu-RTH catalysts, irrespective of hydrothermally aging, showed only one peak at chemical shift of ∼58 ppm, which was attributed to tetrahedral Al (framework Al). After hydrothermal aging, all Cu-RTH catalysts showed a reduced peak at ∼58 ppm, while the tetrahedral Al peak still remained for most catalysts, indicating that the integrity of the zeolite structure was maintained. Octahedral Al (at ∼0 ppm chemical shift) was not observed after dealumination for the hydrothermally aged Cu-RTH catalysts in the 27Al-NMR profiles, while the peak at ∼0 ppm was observed for the hydrothermally aged H-RTH zeolite (Fig. S9†). Therefore, the absence of octahedral Al in Cu-RTH catalysts was probably due to the strong interaction between paramagnetic Cu ions and the as-formed octahedral Al.3 In addition, the 29Si-NMR spectra are displayed in Fig. 7b. The peaks at −112 ppm, −105 ppm and −99 ppm are ascribed to Si (0Al), Si (1Al), and Si (2Al), respectively.44 After hydrothermal aging, the peaks at −105 ppm and −99 ppm decreased, which was consistent with the dealumination shown in 27Al-NMR spectra. The framework Si/Al ratio was also calculated based on the deconvoluted 29Si-NMR profiles (Fig. S10†). Cu-RTH catalysts showed an increase in framework Si/Al ratio after hydrothermal aging (5.6 to 7.9, 5.6 to 7.4 and 5.7 to 6.8 for Cu2.5-RTH, Cu3.5-RTH and Cu4.4-RTH catalysts, respectively.), also indicating the dealumination of zeolite structure. Via analysis of the zeolite structure, it was found that although the dealumination process occurred during hydrothermal aging, the long-range structures and morphological features of Cu-RTH catalysts remained intact, and framework Al was still dominant, indicating a high hydrothermal stability of the zeolite structure.
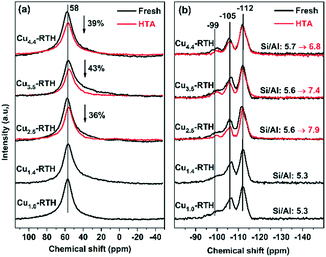 |
| Fig. 7 (a) 27Al and (b) 29Si-NMR profiles of fresh and hydrothermally aged Cu-RTH catalysts with different Cu loadings. | |
4. Discussion
4.1. Copper distribution and its relationship with activity
It has been reported that there were two sites for Cu2+ location next to a 6-membered ring (6MR) and an 8-membered ring (8MR) in Cu-SSZ-13 catalysts.36,45 The two Cu2+ species could be identified via H2-TPR and DRIFTS measurements, in which the H2 consumption peak at ∼350 °C and the IR peak at ∼900 cm−1 are ascribed to the Cu2+ species next to 6MR, while the H2 consumption peak at ∼230 °C and the IR peak at 950 cm−1 are ascribed to the Cu2+ next to 8MR.36,38,40,41 In the case of Cu-RTH catalysts studied in this work, two different H2 consumption peaks (200 °C and 360 °C) and two zeolite T–O–T stretching vibrations (978 cm−1 and 913 cm−1) perturbed by Cu2+ species were also observed. Therefore, analogous to SSZ-13, two sites for Cu2+ location in Cu-RTH catalysts are suggested. The H2 consumption peak at 200 °C and T–O–T vibration at 978 cm−1 were attributed to Cu2+ next to 8MR (α species), while the H2 consumption peak at 360 °C and T–O–T vibration at 913 cm−1 were attributed to Cu2+ next to rth cage (coordinated to 5MR or 6MR, β species). More definitive evidence should be further obtained to clarify the Cu2+ location precisely. Nevertheless, the two sites for Cu2+ location in Cu-RTH catalysts were confirmed, which we labeled as α and β species.
To investigate the two types of Cu2+ species, the TOFs (200–250 °C) of NO conversion in NH3-SCR reaction were calculated based on the data in Fig. S2† and shown in Fig. 8. The TOFs increased slightly as Cu loading varied from 1.0 wt% to 1.4 wt%. However, when the Cu loading reached 2.5 wt%, there was a significant increase in TOF, which was about quadruple of that of the Cu1.4-RTH catalysts. This indicated that another higher active species (α species) appeared rather than an increasing amount of active sites. Therefore, the α species has higher TOF of NO conversion than the β species, resulting in a significant increase in NOx conversion in the NH3-SCR reaction for Cu2.5-RTH compared with that for Cu1.0- and Cu1.4-RTH catalysts with low Cu loadings.
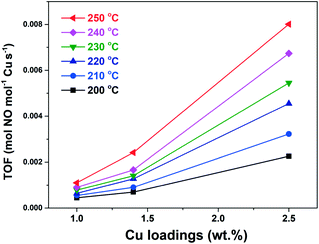 |
| Fig. 8 TOFs of Cu2+ of Cu-RTH catalysts as a function of Cu loading. Conditions: 500 ppm NO, 500 ppm NH3, 5% O2, 5% H2O, N2 balance, and GHSV as shown in Fig. S2.† | |
4.2. Inactivation mechanism of hydrothermal aging
As shown in Fig. 3, the hydrothermal stability of Cu-RTH catalysts increased with the increase in Cu loading. This result was unexpected because Cu-SSZ-13 catalysts have always showed lower hydrothermal stability with the increase in Cu loading because Cu2+ species easily accumulate to form CuOx, and these CuOx clusters damaged the zeolite structure.38,46,47 The effect of Cu loading on hydrothermal aging differs between RTH and CHA zeolites despite the similar nature of the sites of Cu2+ species in the two zeolites. Simultaneously, it is worth noting that the Cu4.4-RTH catalyst was saturated with Cu ions, with an ion-exchange degree of 104% (Cu/Al = 0.52), and showed the best hydrothermal stability among the tested samples. This phenomenon is consistent with studies on the Cu-LTA catalyst, which showed that fully copper-exchanged samples had the highest hydrothermal stability.47,48 As shown in the zeolite structure characterization results, it was found that the RTH zeolite structure was hydrothermally stable, though a weak degree of dealumination occurred (Fig. 7). Therefore, the change in active Cu2+ species should be primarily responsible for the significant decrease in NOx conversion after hydrothermal aging. Comparing the H2-TPR results (Fig. 5) of fresh and hydrothermally aged Cu-RTH catalysts, we found that only the saturated Cu2+ ion exchanged RTH zeolite (Cu4.4-RTH) maintained its highly active Cu sites (α species) for NOx conversion, and exhibited a H2 reduction temperature of 200 °C. Therefore, the Cu4.4-RTH catalyst showed the best NH3-SCR performance after hydrothermal aging (Fig. 3), and the loss of NOx conversion at high temperatures was attributed to the formation of CuOx, which caused non-selective NH3 oxidation. For the Cu2.5-RTH and Cu3.5-RTH catalysts, however, it was found that the Cu2+ species were very stable (high H2 reduction temperature) after hydrothermal aging. Despite the presence of Cu2+, which was identified in XANES and NH3-adsorption DRIFTS results, the hydrothermally aged Cu2.5-RTH and Cu3.5-RTH catalysts lost their NOx conversion activity due to the presence of more stable and inactive Cu2+ species (Cu–aluminum–oxygen complexes). The saturated Cu-exchanged RTH zeolite (Cu4.4-RTH) limited the mobility of Cu2+ during hydrothermal aging due to the high level of Cu2+ ions in the zeolite pores, therefore resulting in some CuOx accumulation and preservation of highly active Cu2+ ions (α species). For the Cu2.5-RTH and Cu3.5-RTH catalysts, in contrast, the Cu2+ species easily migrated to more stable sites, in which Cu2+ showed low activity for NOx reduction after hydrothermal aging. Based on these observations, it may be a good strategy to use other substituting metal ions to partially occupy the exchanged sites and limit Cu mobility in order to increase the hydrothermal stability, which should be explored in future.
5. Conclusions
In this work, Cu-RTH catalysts with varied Cu loadings showed low activation energies (30–40 kJ mol−1) in the NH3-SCR reaction for NOx elimination. Two possible sites for Cu2+ in the Cu-RTH catalysts were suggested to be located next to 8MR (α species) and rth cage (β species). The α species has a significantly higher TOF of NO conversion than the β species. After hydrothermal aging, the zeolite framework structure changed only slightly, but a significant decrease in NOx conversion was observed, which was attributed to the change in Cu2+ species. The Cu2+ species, particularly the α species, were easily movable and transferred to more stable sites during hydrothermal aging, therefore resulting in inactive Cu2+ species for NOx reduction. The fully Cu-exchanged Cu4.4-RTH catalyst (saturation, Cu/Al = 0.52) showed the best hydrothermal stability, which was attributed to the preservation of highly active Cu2+ species (α species) due to the limited Cu2+ ions mobility during hydrothermal aging.
Conflicts of interest
There are no conflicts to declare.
Acknowledgements
This study was financially supported by the National Natural Science Foundation of China (21637005, 51578536).
Notes and references
- J. Park, H. Park, J. Baik, I. Nam, C. Shin, J. Lee, B. Cho and S. Oh, Hydrothermal stability of CuZSM5 catalyst in reducing NO by NH3 for the urea selective catalytic reduction process, J. Catal., 2006, 240, 47–57 CrossRef CAS
.
- J. H. Kwak, R. G. Tonkyn, D. H. Kim, J. Szanyi and C. H. F. Peden, Excellent activity and selectivity of Cu-SSZ-13 in the selective catalytic reduction of NOx with NH3, J. Catal., 2010, 275, 187–190 CrossRef CAS
.
- J. H. Kwak, D. Tran, S. D. Burton, J. Szanyi, J. H. Lee and C. H. F. Peden, Effects of hydrothermal aging on NH3-SCR reaction over Cu/zeolites, J. Catal., 2012, 287, 203–209 CrossRef CAS
.
- X. Shi, F. Liu, L. Xie, W. Shan and H. He, NH3-SCR performance of fresh and hydrothermally aged Fe-ZSM-5 in standard and fast selective catalytic reduction reactions, Environ. Sci. Technol., 2013, 47, 3293–3298 CrossRef CAS PubMed
.
- L. Xie, F. Liu, L. Ren, X. Shi, F. S. Xiao and H. He, Excellent performance of one-pot synthesized Cu-SSZ-13 catalyst for the selective catalytic reduction of NOx with NH3, Environ. Sci. Technol., 2014, 48, 566–572 CrossRef CAS PubMed
.
- C. Yin, P. Cheng, X. Li and R. T. Yang, Selective catalytic reduction of nitric oxide with ammonia over high-activity Fe/SSZ-13 and Fe/one-pot-synthesized Cu-SSZ-13 catalysts, Catal. Sci. Technol., 2016, 6, 7561–7568 RSC
.
- S. Han, Q. Ye, S. Cheng, T. Kang and H. Dai, Effect of the hydrothermal aging temperature and Cu/Al ratio on the hydrothermal stability of CuSSZ-13 catalysts for NH3-SCR, Catal. Sci. Technol., 2017, 7, 703–717 RSC
.
- F. Gao, Y. Zheng, R. K. Kukkadapu, Y. Wang, E. D. Walter, B. Schwenzer, J. Szanyi and C. H. F. Peden, Iron Loading Effects in Fe/SSZ-13 NH3-SCR Catalysts: Nature of the Fe Ions and Structure–Function Relationships, ACS Catal., 2016, 6, 2939–2954 CrossRef CAS
.
- D. Jo, T. Ryu, G. T. Park, P. S. Kim, C. H. Kim, I.-S. Nam and S. B. Hong, Synthesis of High-Silica LTA and UFI Zeolites and NH3–SCR Performance of Their Copper-Exchanged Form, ACS Catal., 2016, 6, 2443–2447 CrossRef CAS
.
- J. Kim, S. J. Cho and D. H. Kim, Facile Synthesis of KFI-type Zeolite and Its Application to Selective Catalytic Reduction of NOx with NH3, ACS Catal., 2017, 7, 6070–6081 CrossRef CAS
.
- J. H. Lee, Y. J. Kim, T. Ryu, P. S. Kim, C. H. Kim and S. B. Hong, Synthesis of zeolite UZM-35 and catalytic properties of copper-exchanged UZM-35 for ammonia selective catalytic reduction, Appl. Catal., A, 2017, 200, 428–438 CrossRef CAS
.
- M. Moliner, C. Franch, E. Palomares, M. Grill and A. Corma, Cu-SSZ-39, an active and hydrothermally stable catalyst for the selective catalytic reduction of NOx, Chem. Commun., 2012, 48, 8264–8266 RSC
.
- R. Q. Zhang, J. Szanyi, F. Gao and J. S. McEwen, The interaction of reactants, intermediates and products with Cu ions in Cu-SSZ-13 NH3 SCR catalysts: an energetic and ab initio X-ray absorption modeling study, Catal. Sci. Technol., 2016, 6, 5812–5829 RSC
.
- A. Shishkin, H. Kannisto, P.-A. Carlsson, H. Harelind and M. Skoglundh, Synthesis and functionalization of SSZ-13 as an NH3-SCR catalyst, Catal. Sci. Technol., 2014, 4, 3917–3926 RSC
.
- R. Oord, I. C. ten Have, J. M. Arends, F. C. Hendriks, J. Schmidt, I. Lezcano-Gonzalez and B. M. Weckhuysen, Enhanced activity of desilicated Cu-SSZ-13 for the selective catalytic reduction of NOx and its comparison with steamed Cu-SSZ-13, Catal. Sci. Technol., 2017, 7, 3851–3862 RSC
.
- D. W. Fickel, E. D'Addio, J. A. Lauterbach and R. F. Lobo, The ammonia selective catalytic reduction activity of copper-exchanged small-pore zeolites, Appl. Catal., A, 2011, 102, 441–448 CrossRef CAS
.
- J. D. Albarracin-Caballero, I. Khurana, J. R. Di Iorio, A. J. Shih, J. E. Schmidt, M. Dusselier, M. E. Davis, A. Yezerets, J. T. Miller, F. H. Ribeiro and R. Gounder, Structural and kinetic changes to small-pore Cu-zeolites after hydrothermal aging treatments and selective catalytic reduction of NOx with ammonia, React. Chem. Eng., 2017, 2, 168–179 RSC
.
- N. Martín, C. Paris, P. N. R. Vennestrøm, J. R. Thøgersen, M. Moliner and A. Corma, Cage-based small-pore catalysts for NH3 -SCR prepared by combining bulky organic structure directing agents with modified zeolites as reagents, Appl. Catal., A, 2017, 217, 125–136 CrossRef
.
- F. Xiao, H. Xu, Q. Wu, Y. Chu, J.-g. Jiang, L. Zhang, S. Pan, C. Zhang, L. Zhu, F. Deng, X. Meng, S. Maurer, R. McGuire, A.-N. Parvulescu and U. Mueller, Efficient synthesis of aluminosilicate RTH zeolite with good catalytic performances in NH3-SCR and MTO reactions, J. Mater. Chem. A, 2018, 6, 8705–8711 RSC
.
- D. Jo, J. B. Lim, T. Ryu, I.-S. Nam, M. A. Camblor and S. B. Hong, Unseeded hydroxide-mediated synthesis and CO2 adsorption properties of an aluminosilicate zeolite with the RTH topology, J. Mater. Chem. A, 2015, 3, 19322–19329 RSC
.
- L. Ren, L. Zhu, C. Yang, Y. Chen, Q. Sun, H. Zhang, C. Li, F. Nawaz, X. Meng and F. S. Xiao, Designed copper-amine complex as an efficient template for one-pot synthesis of Cu-SSZ-13 zeolite with excellent activity for selective catalytic reduction of NOx by NH3, Chem. Commun., 2011, 47, 9789–9791 RSC
.
- M. Newville, Interactive XAFS Analysis and FEFF Fitting, J. Synchrotron Radiat., 2001, 322–324 CrossRef CAS
.
- J. H. Kwak, D. Tran, J. Szanyi, C. H. F. Peden and J. H. Lee, The Effect of Copper Loading on the Selective Catalytic Reduction of Nitric Oxide by Ammonia Over Cu-SSZ-13, Catal. Lett., 2012, 142, 295–301 CrossRef CAS
.
- F. Gao, E. D. Walter, M. Kollar, Y. Wang, J. Szanyi and C. H. F. Peden, Understanding ammonia selective catalytic reduction kinetics over Cu/SSZ-13 from motion of the Cu ions, J. Catal., 2014, 319, 1–14 CrossRef CAS
.
- C. Paolucci, I. Khurana, A. A. Parekh, S. Li, A. J. Shih, H. Li, J. R. D. Iorio, J. D. Albarracin-Caballero, A. Yezerets, J. T. Miller, W. N. Delgass, F. H. Ribeiro, W. F. Schneider and R. Gounder, Dynamic multinuclear sites formed by mobilized copper ions in NOx selective catalytic reduction, Science, 2017, 357, 898–903 CrossRef CAS PubMed
.
- F. Gao, D. Mei, Y. Wang, J. Szanyi and C. H. Peden, Selective Catalytic Reduction over Cu/SSZ-13: Linking Homo- and Heterogeneous Catalysis, J. Am. Chem. Soc., 2017, 139, 4935–4942 CrossRef CAS PubMed
.
- C. Paolucci, A. A. Parekh, I. Khurana, J. R. Di Iorio, H. Li, J. D. Albarracin Caballero, A. J. Shih, T. Anggara, W. N. Delgass, J. T. Miller, F. H. Ribeiro, R. Gounder and W. F. Schneider, Catalysis in a Cage: Condition-Dependent Speciation and Dynamics of Exchanged Cu Cations in SSZ-13 Zeolites, J. Am. Chem. Soc., 2016, 138, 6028–6048 CrossRef CAS PubMed
.
- A. M. Beale, F. Gao, I. Lezcano-Gonzalez, C. H. Peden and J. Szanyi, Recent advances in automotive catalysis for NOx emission control by small-pore microporous materials, Chem. Soc. Rev., 2015, 44, 7371–7405 RSC
.
- J. Wang, H. Zhao, G. Haller and Y. Li, Recent advances in the selective catalytic reduction of NOx with NH3 on Cu-Chabazite catalysts, Appl. Catal., A, 2017, 202, 346–354 CrossRef CAS
.
- E. Borfecchia, K. A. Lomachenko, F. Giordanino, H. Falsig, P. Beato, A. V. Soldatov, S. Bordiga and C. Lamberti, Revisiting the nature of Cu sites in the activated Cu-SSZ-13 catalyst for SCR reaction, Chem. Sci., 2015, 6, 548–563 RSC
.
- S. A. Bates, A. A. Verma, C. Paolucci, A. A. Parekh, T. Anggara, A. Yezerets, W. F. Schneider, J. T. Miller, W. N. Delgass and F. H. Ribeiro, Identification of the active Cu site in standard selective catalytic reduction with ammonia on Cu-SSZ-13, J. Catal., 2014, 312, 87–97 CrossRef CAS
.
- I. Lezcano-Gonzalez, U. Deka, H. E. van der Bij, P. Paalanen, B. Arstad, B. M. Weckhuysen and A. M. Beale, Chemical deactivation of Cu-SSZ-13 ammonia selective catalytic reduction (NH3-SCR) systems, Appl. Catal., A, 2014, 154–155, 339–349 CrossRef CAS
.
- I. Lezcano-Gonzalez, D. S. Wragg, W. A. Slawinski, K. Hemelsoet, A. Van Yperen-De Deyne, M. Waroquier, V. Van Speybroeck and A. M. Beale, Determination of the Nature of the Cu Coordination Complexes Formed in the Presence of NO and NH3 within SSZ-13, J. Phys. Chem. C, 2015, 119, 24393–24403 CrossRef CAS
.
- J. H. Kwak, T. Varga, C. H. F. Peden, F. Gao, J. C. Hanson and J. Szanyi, Following the movement of Cu ions in a SSZ-13 zeolite during dehydration, reduction and adsorption: A combined in situ TP-XRD, XANES/DRIFTS study, J. Catal., 2014, 314, 83–93 CrossRef CAS
.
- L. Xie, F. Liu, X. Shi, F.-S. Xiao and H. He, Effects of post-treatment method and Na co-cation on the hydrothermal stability of Cu–SSZ-13 catalyst for the selective catalytic reduction of NOx with NH3, Appl. Catal., A, 2015, 179, 206–212 CrossRef CAS
.
- J. Hun Kwak, H. Zhu, J. H. Lee, C. H. Peden and J. Szanyi, Two different cationic positions in Cu-SSZ-13?, Chem. Commun., 2012, 48, 4758–4760 RSC
.
- F. Gao, E. D. Walter, E. M. Karp, J. Luo, R. G. Tonkyn, J. H. Kwak, J. Szanyi and C. H. F. Peden, Structure–activity relationships in NH3-SCR over Cu-SSZ-13 as probed by reaction kinetics and EPR studies, J. Catal., 2013, 300, 20–29 CrossRef CAS
.
- Y. J. Kim, J. K. Lee, K. M. Min, S. B. Hong, I.-S. Nam and B. K. Cho, Hydrothermal stability of CuSSZ13 for reducing NOx by NH3, J. Catal., 2014, 311, 447–457 CrossRef CAS
.
- T. Zhang, F. Qiu and J. Li, Design and synthesis of core-shell structured meso-Cu-SSZ-13@mesoporous aluminosilicate catalyst for SCR of NOx with NH3: Enhancement of activity, hydrothermal stability and propene poisoning resistance, Appl. Catal., A, 2016, 195, 48–58 CrossRef CAS
.
- J. Luo, D. Wang, A. Kumar, J. Li, K. Kamasamudram, N. Currier and A. Yezerets, Identification of two types of Cu sites in Cu/SSZ-13 and their unique responses to hydrothermal aging and sulfur poisoning, Catal. Today, 2016, 267, 3–9 CrossRef CAS
.
- J. Luo, F. Gao, K. Kamasamudram, N. Currier, C. H. F. Peden and A. Yezerets, New insights into Cu/SSZ-13 SCR catalyst acidity. Part I: Nature of acidic sites probed by NH3 titration, J. Catal., 2017, 348, 291–299 CrossRef CAS
.
- D. Wang, L. Zhang, K. Kamasamudram and W. S. Epling, In Situ-DRIFTS Study of Selective Catalytic Reduction of NOx by NH3 over Cu-Exchanged SAPO-34, ACS Catal., 2013, 3, 871–881 CrossRef CAS
.
- L. Zhang, D. Wang, Y. Liu, K. Kamasamudram, J. Li and W. Epling, SO2 poisoning impact on the NH3-SCR reaction over a commercial Cu-SAPO-34 SCR catalyst, Appl. Catal., A, 2014, 156-157, 371–377 CrossRef CAS
.
- J. Klinowski, Solid-State NMR studies of molecular sieve catalysts, Chem. Rev., 1991, 91, 1459–1479 CrossRef CAS
.
- C. W. Andersen, M. Bremholm, P. N. Vennestrom, A. B. Blichfeld, L. F. Lundegaard and B. B. Iversen, Location of Cu(2+) in CHA zeolite investigated by X-ray diffraction using the Rietveld/maximum entropy method, IUCrJ, 2014, 1, 382–386 CrossRef CAS PubMed
.
- J. Song, Y. Wang, E. D. Walter, N. M. Washton, D. Mei, L. Kovarik, M. H. Engelhard, S. Prodinger, Y. Wang, C. H. F. Peden and F. Gao, Toward Rational Design of Cu/SSZ-13 Selective Catalytic Reduction Catalysts: Implications from Atomic-Level Understanding of Hydrothermal Stability, ACS Catal., 2017, 7, 8214–8227 CrossRef CAS
.
- T. Ryu, N. H. Ahn, S. Seo, J. Cho, H. Kim, D. Jo, G. T. Park, P. S. Kim, C. H. Kim, E. L. Bruce, P. A. Wright, I. S. Nam and S. B. Hong, Fully Copper-Exchanged High-Silica LTA Zeolites as Unrivaled Hydrothermally Stable NH3-SCR Catalysts, Angew. Chem., Int. Ed., 2017, 56, 3256–3260 CrossRef CAS PubMed
.
- N. H. Ahn, T. Ryu, Y. Kang, H. Kim, J. Shin, I.-S. Nam and S. B. Hong, The Origin of an Unexpected Increase in NH3–SCR Activity of Aged Cu-LTA Catalysts, ACS Catal., 2017, 7, 6781–6785 CrossRef CAS
.
Footnote |
† Electronic supplementary information (ESI) available: N2O production, kinetic studies, XANES and EXAFS of referenced materials, H2 consumption of H2-TPR, N2 adsorption–desorption isotherms and SEM images. See DOI: 10.1039/c8cy01933a |
|
This journal is © The Royal Society of Chemistry 2019 |