DOI:
10.1039/C8CS00494C
(Tutorial Review)
Chem. Soc. Rev., 2019,
48, 22-37
Optical nano-agents in the second near-infrared window for biomedical applications
Received
23rd August 2018
First published on 16th November 2018
Abstract
The optical technology presents non-invasive, non-destructive, and non-ionizing features and has the ability to display various chemical components in tissues to provide useful information for various biomedical applications. Regarding selection of light wavelengths, second near-infrared (NIR-II, 900–1700 nm) light is a much better choice compared to both visible (380–780 nm) and traditional near-infrared (780–900 nm) light, because of its advantages including deeper penetration into biological tissues, less tissue scattering or absorption, and decreased interference by fluorescent proteins. Thus, using optical nano-agents that absorb or emit light in the NIR-II window can achieve deeper tissue optical imaging with higher signal-to-background ratios and better spatial resolution for diagnosis. What's more, some of these nano-agents can be further applied for imaging guided surgical removal, real-time monitoring of drug delivery, labeling lymphatic metastasis, biosensing, and imaging guided phototherapy. In this review, we attempt to summarize the recent advances of various NIR-II nano-agents (including single-walled carbon nanotubes, quantum dots, rare-earth doped nanoparticles, other inorganic nanomaterials, small organic molecule-based nanoparticles, and semiconducting polymer nanoparticles) in both bioimaging and therapeutic applications, and discuss the challenges and perspectives of these nano-agents for clinical practice in the near future.
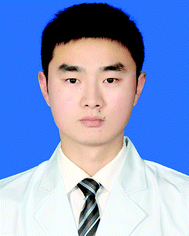
Yu Cai
| Dr Yu Cai received his PhD degree from the Institute of Advanced Materials, Nanjing Tech University, in 2017, supervised by Prof. Xiaochen Dong. Then he moved to the Central Laboratory of Stomatology in Nanjing Stomatological Hospital as an assistant research fellow. This was followed by a period of visiting scholar research at Nanyang Technological University (Singapore). Currently, his work focuses on biomedical phototheranostics. |
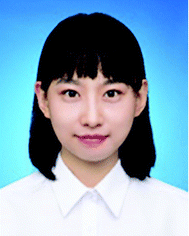
Zheng Wei
| Miss Zheng Wei received her master's degree from the Medical School of Nanjing University in 2018. Then she moved to the Children Department of Nanjing Stomatological Hospital as a dentist. Currently, she is devoted to the study for sensitized oral squamous cell carcinomas with nano-agents. |
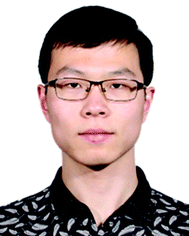
Chuanhui Song
| Mr Chuanhui Song earned his bachelor's degree in 2017. Now he is a PhD candidate in oral and maxillofacial surgery as a graduate student. Currently, his work focuses on head and neck squamous cell carcinoma phototheranostics. |
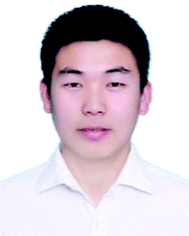
Chuanchao Tang
| Mr Chuanchao Tang obtained his bachelor's degree from Anhui Medical University in 2017. He is pursuing his master's degree at the Medical School of Nanjing University. Currently, his work focuses on NIR-II phototheranostics. |
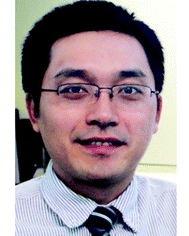
Wei Han
| Prof. Wei Han is currently an Associate Professor in the Medical School of Nanjing University and a deputy director in the Department of Oral and Maxillofacial Surgery at Nanjing Stomatology Hospital. He completed his PhD in Shanghai Jiaotong University in 2011. He has some periods as a visiting scholar in Anderson Cancer Center (USA), Leeds Dental Institute (UK), and Ludwig-Maximilians-Universität (Germany), respectively. His current research focuses on oncotherapy, cytobiology, and bionanotechnology. |
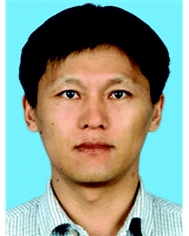
Xiaochen Dong
| Prof. Xiaochen Dong obtained his PhD degrees from Zhejiang University in China in 2007, supervised by Prof. Li Wang. Then he joined the School of Materials Science and Engineering in Nanyang Technological University as a postdoc. In 2012, he joined the Institute of Advanced Materials, NanjingTech University, as a Full Professor. He has published more than 180 papers, including those in Adv. Mater., ACS Nano, etc. The current research involves biophotonics and bioelectronics, and carbon-based nanomaterials. |
Key learning points
(1) The general concept and importance of NIR-II nano-agents for biomedical applications.
(2) Photophysical and photochemical properties of NIR-II nano-agents.
(3) Design and application guidelines of NIR-II nano-agents.
(4) Various NIR-II nano-agents for fluorescence imaging, photoacoustic imaging, biosensing, imaging guided therapy and phototherapy.
(5) Perspectives and considerations of NIR-II nano-agents for future clinical applications.
|
1. Introduction
The optical technology has attracted increasing attention in various fields of biomedical diagnosis and therapy due to its characteristics including high precision, high efficiency, and non-destructiveness. Different from traditional diagnostic and therapeutic methods of diseases, photo-induced imaging and therapy present non-invasive, non-destructive, and non-ionizing features, and have the ability to display various chemical components in tissues to provide more useful information. However, biological tissues can reflect, scatter and absorb light. For instance, when a beam of light is directed to a finger, the finger can be seen because the light is reflected diffusely on the surface. At the same time, light can be observed on the back of the finger, indicating that the light is scattered by tissues with a changed direction. Also, the finger may feel warm as the tissues absorb light. The light interacts with the tissue medium during the irradiation process, demonstrating the ability of biological tissues to reflect, scatter and absorb light, respectively. In particular, most incident visible (VIS, 380–780 nm) light to organisms is scattered and absorbed by surface tissues, and hardly penetrates into deep tissues. With longer wavelengths, near-infrared (NIR, 780–1700 nm) light displays less tissue scattering and absorption, which leads to much higher penetration efficiency into biological tissues. Therefore, NIR light has been applied in a variety of biomedicine fields. For instance, the NIR fluorescence (FL) imaging technique, which can emit FL with the excitation light for biological imaging, provides the advantages of deep tissue penetration and reduced background auto-fluorescence interference from organisms and tissues. Different form FL imaging, photoacoustic (PA) imaging is the other non-invasive NIR optical imaging technology, which transforms NIR excitation into ultrasonic signals to achieve biological tissue images with higher contrast, better spatial resolution, and a decreased optical diffusion limitation in the meantime. More than that, these optical imaging technologies can not only be used for biomedical diagnosis, but also be developed for some further applications such as real-time monitoring of drug delivery, lymphatic metastasis labeling, imaging guided surgical removal or phototherapy. Phototherapy, including photodynamic therapy (PDT) and photothermal therapy (PTT), has attracted extensive research interest due to its minimal harm to normal tissues, non-invasiveness, and efficient anti-tumor capability. In the process of PDT, photosensitizers (PSs) under NIR illumination can generate reactive oxygen species (ROS) with surrounding oxygen to kill pathological cells. On the other hand, PTT converts NIR light into heat through photothermal agents to induce cellular hyperthermia-based apoptosis or necrosis for the treatment of serious diseases such as cancers. On top of the individual treatment, phototherapy is usually employed as an adjuvant therapeutic way to assist other therapeutic strategies (chemotherapy, radiotherapy, surgery, immunotherapy, etc.), which can acquire extraordinary synergistic therapeutic effects for better treatment.1
For photo-induced biomedicine, optical nano-agents are essential factors for light absorption and energy conversion in both diagnostic and therapeutic processes, and the nanostructure can provide the advantages of selective targeting to diseases (especially for tumors) based on the enhanced permeability and retention (EPR) effect, improving the theranostic effect with a much lower dosage, and decreasing the side effects to the normal organs. Moreover, these nano-agents in an aggregation state usually show redshift absorption and emission in the NIR region, which is beneficial to theranostics for deep tissue diseases with increased light penetration. Besides, the aggregating nano-agents also provide the ability to promote photothermal conversion under NIR irradiation with enhanced surface plasma resonance, which makes them promising candidates for high-efficiency PTT. In the past decades, plenty of optical nano-agents in the traditional NIR window (NIR-I, 780–900 nm) have been extensively studied in both animal and clinical applications, but the limited tissue penetration depth and apparent photon scattering of NIR-I may still restrict further development. As is well-known, the degree of scattering for biological tissues follows an inversely proportional wavelength relationship (∼λ−w, the exponent (w) varies from 0.22 to 0.68 for various tissues), indicating that reduced scattering and higher photon penetration depths for biological tissues can be achieved at longer wavelengths. Although the tissue penetration depth of NIR-I light (less to 1 cm) is about an order of magnitude deeper than VIS photons, NIR-I seems not the ultimate choice of light source for various deep tissue diseases. Thus, the deeper tissue penetration is increasingly desired for better optical imaging and therapy. Very recently, it has been demonstrated that FL imaging in the second NIR window (NIR-II, which is a part of the electromagnetic spectrum with the approximate range of 900–1700 nm, and also a section of shortwave-infrared, SWIR)2 provides additional advantages with the innovations to achieve feature contrast at greater imaging depths (up to 3 cm), good temporal and spatial imaging resolutions (20 ms and 25 μm), a better real-time monitoring ability, and a higher signal-to-noise ratio (because of the lower tissue absorption and less light scattering) compared to both the VIS and NIR-I windows.3 Also, PA imaging in the NIR-II region can overcome the intrinsic problems of attenuation arising from NIR-I excitation on account of optical absorption and scattering, and decrease the optical absorption of blood and other biological compounds for higher imaging contrast and sensitivity.4 For phototherapy, the higher maximum permissible exposure and deeper tissue penetration of NIR-II light have been identified to show the advantages of lower energy of photons and reduced tissue scattering compared to the NIR-I phototherapy. Besides, the subsistent NIR-II imaging ability of these therapeutic agents can provide much more accurate diagnostic information about targeted tissues compared to more effective phototherapy or other therapeutic treatment methods. These significant improvements inspire many researchers to develop efficient NIR-II nano-agents and further explore their potential for biomedical applications such as diagnosis imaging, imaging guided surgical removal, real-time monitoring of drug delivery, labeling of lymphatic metastasis, and imaging guided phototherapy. In 2007, Dai and co-workers first reported the use of semiconducting single-walled carbon nanotubes (SWNTs) with small band-gaps on the order of ∼1 eV as NIR-II fluorescent tags for selective probing of cell surface receptors with high specificity and high sensitivity.5 After that, various optical nano-agents with low band gaps and small effective masses have been investigated, such as quantum dots (QDs), rare-earth doped nanoparticles (NPs), some other inorganic nanomaterials, small organic molecule-based fluorophores and semiconducting polymer NPs (SPNs). These nano-agents with maximal depths of signal penetration, enhanced contrast resolution, locally minimized absorption by tissues, and good biocompatibility present an excellent imaging or therapeutic ability, which make them applicable in various biomedical applications in the NIR-II region (Fig. 1). Although there have been a few reviews summarizing fluorophores in the NIR-II window,6,7 they mainly focused on biological imaging only but less on the much broader and comprehensive biomedical applications that have been achieved in very recent years. Herein, we attempt to review the recent advances of these optical NIR-II nano-agents and their diversiform features in the NIR-II region, highlighting their applications in optical imaging, biosensing, drug delivery, imaging guided therapy and phototherapy for biomedicine, and discuss their challenges and perspectives for the near future clinical practice.
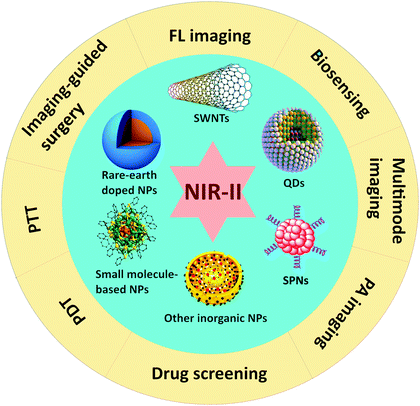 |
| Fig. 1 Optical nano-agents for biomedical applications in the NIR-II window. | |
2. Design and application guidelines of NIR-II nano-agents
Fluorescent markers or therapeutic agents have outstanding biological application potential with relatively deep penetration and low background signals in the NIR-II spectral range. Inorganic nanomaterials with interesting optical properties have already been actively pursued in past years for biological research. With tunable narrow band-gaps to generate photoluminescence (PL) in the NIR-II window, SWNTs, semiconductor QDs, rare-earth doped NPs and some other inorganic nanomaterials (such as mesoporous silica, magnetic iron, and gold NPs) have been used in various medical applications both in vitro and in vivo. On the other hand, organic dyes including small molecules and semiconducting polymers with an alternating electron deficient group (acceptor, A) and electron rich group (donor, D) present strong and sharp absorption and emission in the NIR-II window. Theoretically, to obtain D–A semiconducting structures in the NIR-II region, decreasing the band gap between the highest occupied molecular orbitals (HOMO) and the lowest unoccupied molecular orbitals (LUMO) is usually an efficient strategy. In addition, the design of both inorganic and organic materials should also meet requirements of tunable emission, long lifetimes, and high emission quantum yields (QYs). In particular, water shows an increased absorbance in the region of 1400–1500 nm, which could decrease the light intensity for passing through biological tissues and even cause damage to normal tissues. Therefore, in the design of NIR-II agents, this region of both absorption and emission should be typically avoided to reduce the unnecessary side effects in the subsequent biomedical applications.
Besides the chemical structure demands, these NIR-II nano-agents should also overcome some physical problems such as water solubility and chemical/photo/thermal-stability. For example, poor water solubility will limit the bioavailability of the agents and hamper the development of these agents identified in the early drug screening, and may also affect their stability in the biosystems. Besides, nano-agents with excellent biocompatibility can protect themselves from rapid biodegradation or excretion and will improve their pharmacokinetic profile. Ideally, when the agents are delivered into the body for a special medical purpose, they should perform the desired function and be excreted from the body without any side effects. Thus, it is essential to comprehensively analyze these NIR-II nano-agents from a toxicological perspective. NIR-II nano-agents should be easily distributed and selectively targeted to pathological tissues. Distribution of agents is determined by the physico-chemical properties and is usually limited by drug penetration to diseased regions such as tumor tissues.8 Therefore, nano-agents with both passive and active targeting abilities may improve drug penetration into both tumor cells and stromal compartment cells. Above all, the biggest payoff of these optical nano-agents lies in the realization of phototheranostics that combines the functions of therapy and diagnosis into a single nano-formulation, which allows real-time monitoring during the entire therapeutic action. Thus, the development of NIR-II phototheranostic nano-agents for see–treat–see action will be of significant value to patients in the clinic.
3. SWNTs for FL imaging and sensing
One-dimensional (1D) semiconducting SWNTs that possess a narrow band gap of ∼1 eV and produce possible FL emission in the NIR-II region are attractive candidates for NIR-II biological sensing and imaging. On the other hand, SWNTs are ideal biological fluorescent probes due to their inherently large Stokes shift, which allows NIR excitation in the biological transparency window and further reduces the auto-fluorescence in the NIR-II window and the background effects of scattering. However, the biggest challenge of SWNT NIR-II probes is their rather low brightness and poor biocompatibility. Therefore, the application of SWNTs in vivo requires appropriate functionalization to enhance their photoluminescence quantum yield (PLQY) and reduce their biotoxicity.
After the study of SWNTs for selective probing and imaging of cell surface receptors, Dai and co-workers further studied the whole-animal NIR-II PL imaging of SWNTs using an indium–gallium–arsenide (InGaAs) camera in vivo.9 With SWNTs suspended in phospholipid–polyethylene glycol (PL–PEG), the required dose would be 10 times lower than the original amount. Importantly, almost no autofluorescence background was detected in the SWNTs’ emission range (1100–1700 nm), and high resolution tumor vessel imaging was done through thick skin in vivo (Fig. 2). The biocompatible SWNTs with bright fluorescence were further used for video-rate in vivo NIRII imaging of vascular structures in the mouse hind limb (∼30 μm) with the InGaAs imaging system. Video-rate FL imaging of the injected SWNT solution allowed crisp resolution of anatomical features by application of dynamic contrast imaging based on principal component analysis (PCA). These time-based dynamic contrast enhanced images and raw video were both used to trace the SWNTs through the mouse anatomy 130 s post-injection. These experimental results were then backed up by Monte Carlo simulations, which presented beneficial effects of reduced tissue scattering on deeply fluorophores emitting in the NIR-II region. Using amphiphilic poly(maleic anhydride-alt-1-octadecene)-methoxy poly(ethylene glycol) (C18-PMH-mPEG) coating for SWNTs, only 30% injected dose of original SWNTs was achieved for the same tumor accumulation efficiency, which also presented a high tumor accumulation level for a long circulation time. Under 808 nm excitation, bright FL imaging of SWNTs at the tumor sites could be achieved in the 1000–1400 nm NIR-II window. NIR-II FL imaging also helped the development of peripheral arterial disease (PAD) therapy by providing hemodynamic and anatomic information with pretty high spatial and temporal resolution. The NIR-II imaging window for vascular imaging achieved spatial resolution down to approximately 30 μm and high temporal (<200 ms per frame) resolution at a depth of ∼3 mm in the mouse brain with the intact skull and hind limb, which were beyond the capability of ultrasonography at low blood velocities (∼3 fold improvement). Furthermore, simultaneous single-vessel resolved blood-flow speed mapping for multiple hind limb arterial vessels was achieved by video-rate FL imaging. NIR-II FL imaging with SWNTs as the innovative technology has presented outstanding superiorities in biomedical imaging both in vitro and in vivo, which has been superior to many other imaging techniques and stimulated the search for other nano-agents in the NIR-II region. Heller et al. reported a SWNT-based sensor that can arise from competitive effects between the displacement of both water from the nanotube surface and oligonucleotide charge groups, and lead to a solvatochromism-like response for real-time monitoring of the optical quantification of the hybridization events of microRNA and other oligonucleotides via NIR-II FL imaging in live mice.10 In another work, they developed a kind of single chiral SWNT composed of short single stranded DNA as an optical reporter that responds to lipid accumulation by the modulation of the optical band gap of SWNTs. This optical reporter that emits narrow-bandwidth FL in the NIR-II region can exclusively localize to the lumen of endolysosomal organelles of endolysosomal lipid content in live cells, which conferred the capabilities for the investigation of lipid-linked diseases and drug development processes.11 Strano et al. used non-covalent conjugation of an aptamer-anchor polynucleotide sequence to NIR-II emissive SWNTs to fabricate nanosensor arrays, which can achieve real-time, single-cell analysis of various protein products from different cell types.12 After that, they further developed an optical sensor array using a functionalized SWNTs/chitosan ink that enables a divalent ion-based proximity quenching mechanism for transducing binding between an antibody or a capture protein with the targeted analyte. This NIR-II optically addressable platform has the potential to advance the multiplexed and real-time biomolecular detection of complex mixtures.13 In neural networks, spatial and temporal changes in neurotransmitter concentrations are significant in information processing. Functional SWNTs can achieve selective detection of specific neurotransmitters, such as dopamine for neuroscience in the living brain. For example, corona phase molecular recognition is a new technique that identifies adsorbed polymer phases on SWNTs, which can act as excellent fluorescent neurotransmitter sensors in the NIR-II window. To mitigate the signal attenuation from the absorption and scattering of excitation photons in brain FL imaging, Landry et al. used DNA-wrapped SWNTs with a 1560 nm excitation source (femtosecond pulsed erbium laser) for two-photon (fluorescent molecules excited using a laser that absorb two long wavelength photons at the same time and emit one shorter wavelength photon) through-cranium imaging, which achieved a two-fold increase in the molecular recognition of dopamine compared to traditional SWNT nanosensor FL imaging in the presence of 100 × 10−6 M dopamine. This new two-photon methodology enables neurotransmitter imaging in the living brain and other biologically intact milieux.14 Godin et al. injected SWNTs into rat cerebroventricles and the NIR-II emission of individual nanotubes as they diffuse inside the extracellular space for tens of minutes in acute slices, which showed a direct approach to observe the local extracellular space rheology and structures in the brain using super-resolution NIR-II imaging.15 Nevertheless, the future research and applications of SWNTs may focus on increasing the QY to enhance the FL intensity for much brighter and multicolored NIR-II FL imaging.
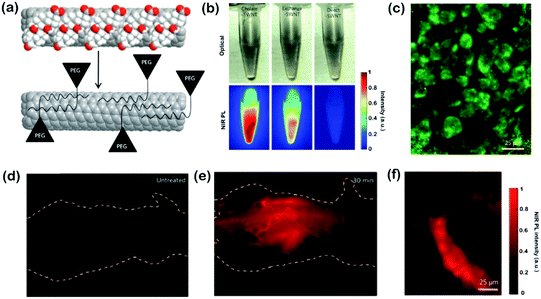 |
| Fig. 2 (a) Schematic of the exchange process on SWNTs. Cholate (red and white balls) was dialyzed and replaced by PL–PEG eventually to form biocompatible nanotubes. (b) NIR-II PL images obtained upon 808 nm excitation of the three solutions at equal concentrations. Exchange-SWNTs presented greater FL yields than direct-SWNTs. (c) NIR-II PL images of human malignant glioma cells (U87 MG) treated with exchange-SWNTs/RGD. (d) NIR-II PL image of an untreated nude mouse showing bare auto-fluorescence. (e) NIR-II PL image of a nude mouse treated with 200 mL of 17 mg L−1 exchange-SWNTs after 30 min. (f) Intravital NIR-II PL image of tumor vessels of injection of exchange-SWNTs (170 mg L−1, 300 mL) within 90 min. Adapted with permission from ref. 9. Copyright 2009 Nature Publishing Group. | |
4. QDs for FL imaging and drug delivery
QDs as a kind of zero-dimensional nanomaterial have been widely studied due to their unique photophysical and photochemical properties such as easy modification of surfaces, size-dependent emission, and high photostability. As a consequence, various QDs have been proposed, with emission ranging from the ultraviolet (UV) to NIR region for bioimaging and biosensing. Recently, QDs with longer wavelength emission have presented promising NIR-II FL imaging potential. For the preparation of NIR-II QDs, one fundamental principle is to obtain a narrower bandgap, which minimizes the energy of an electron jumping from the ground-state valence band to an excited state conduction band. For typical types of QDs, the bandgap energy mainly depends on the particle size within their Bohr diameter, known as the quantum size effect. However, their optical properties are not easily tuned by only this effect. Alternatively, the semiconductor properties can be chemically manipulated to reduce their bandgap. Primordially, II–VI (CdTe, HgTe), III–V (InP, InAs, GaAs), and IV–VI (PbS, PbSe) QDs are the most extensively studied NIR-II QDs. However, the applications of these QDs have been severely limited due to the presence of toxic ions such as Pb2+, Hg2+, Cd2+, and As3−. To overcome this problem, surface coating with biocompatible substances is frequently introduced to obtain core/shell nanostructures, which may improve the biocompatibility and reduce surface defects as well, thus enhancing the bioavailability. However, well controlled synthesis of core/shell nanostructures is a very challenging task, and additional coating will increase the overall size of QDs, which is undesirable for NIR-II luminescence applications. To overcome these drawbacks, many studies focus on manufacturing novel QDs that can simultaneously exhibit good biocompatibility and NIR-II emission. The most promising alternatives are I–VI-based QDs (Ag2S, Ag2Se, Ag2Te, etc.), which exhibit less cytotoxicity, bright NIR-II luminescence, high photostability, and high PLQYs; therefore, research studies on the design and application of I–IV-based QDs are rapidly increasing for biomedical NIR-II FL imaging.
4.1. Ag2S QDs
In 2012, Wang et al. first reported the use of NIR-II Ag2S QDs with a high PLQY (15.5%, approximately 5-fold that of SWNTs) for imaging xenograft tumors with emission at about 1200 nm.16 These Ag2S QDs can be used to detect xenograft tumors through passive targeting based on the EPR effect with a high signal-to-background ratio. Both in vitro and in vivo NIR-II imaging results were achieved using the Ag2S QDs, which demonstrated them to be attractive NIR-II FL probes with decent biocompatibility and high quantum efficiency. In their subsequent work, Ag2S QDs were functionalized with polyethylene glycol (PEG) for the systematic study of the long-term biodistribution and potential toxicity in vivo. PEGylated-Ag2S QDs were accumulated in the reticuloendothelial system (RES) including the spleen and liver after intravenous administration and were cleared slowly by fecal excretion. In the meantime, PEGylated-Ag2S QDs showed no appreciable toxicity by hematological analysis, blood biochemistry and histological examinations at doses of 15 and 30 mg kg−1 for mice. Using these Ag2S QDs, traces of transplanted stem cells in living animals could be achieved with high sensitivity and spatial resolution, real-time imaging, and long-term tracking. Besides, Ag2S QD-based NIR-II imaging was further employed to dynamically visualize the distribution and migration of human mesenchymal stem cells (hMSCs) in response to SDF-1α at the cutaneous wound site with an enhanced healing effect, which elucidated the mechanism of stem cell-based regeneration medicine and would promote potential clinical applications. Moreover, few Ag2S QD-labeled hMSCs were detectable and their FL intensity could maintain for approximately 30 days in vivo, and the dynamic distribution and in situ translocation of transplanted hMSCs in the liver and lung could be monitored even after 14 days with a high temporal resolution (100 ms). Based on the effect of metal enhanced fluorescence (MEF), the FL intensity in the NIR-II region of Ag2S QDs with Au modification can be enhanced over 100 times compared to Ag2S QDs only, which paved the way for high-end imaging and sensing applications of Ag2S QDs. On top of the individual FL imaging function, Ag2S QDs can also act as a novel theranostic nanoplatform for simultaneous diagnosis, therapeutic monitoring and drug delivery of tumors in vivo by loading typical anticancer drugs. Huang et al. used an amphiphilic poly(amino acid) to design nanocapsules for co-encapsulating Ag2S QDs and hydrophobic drugs (paclitaxel and camptothecine) for delivery; the obtained nanosystem can facilitate tumor targeting, pH-responsive release, and real-time NIR-II FL tracking, and was regarded as a safe, efficient, and useful tool for in vivo drug delivery. Another kind of nanodrug (T&D@RGD-Ag2S) also employed Ag2S QDs for in situ NIR-II monitoring, which can effectively inhibit tumor growth by integrating the endothelial inhibitor O-(chloroacetyl-carbamoyl)fumagillol (TNP-470), the specific recognition peptide cyclo(Arg-Gly-Asp-D-Phe-Cys) (cRGD), and the chemotherapeutic drug doxorubicin (DOX) for synergistic targeting tumor therapy. This tumor vasculature-targeted strategy by real time monitoring with Ag2S QDs was a new method for tumor treatment with promise for future clinical applications (Fig. 3).17 Through self-assembly, Ag2S QDs, indocyanine green (ICG), and amphipathic C18/PEG polymer molecules can be fabricated into an ICG@PEG-Ag2S nanoprobe, which can real-time monitor atherosclerosis by combining high contrast enhanced PA imaging of ICG and high signal-to-noise ratio (SNR) NIR-II FL imaging of Ag2S QDs and in vitro histological assessment.
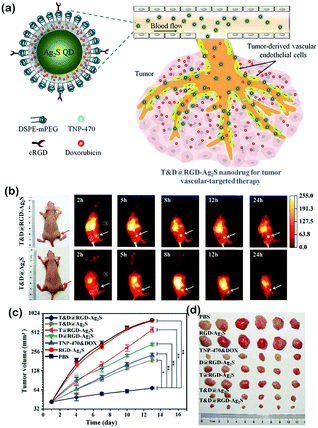 |
| Fig. 3 (a) Schematic of a T&D@RGD-Ag2S nanodrug for tumor vasculature-targeted therapy. (b) Time course of NIR-II FL imaging of U87-MG tumor-bearing mice injected with T&D@RGD-Ag2S (top) or T&D@Ag2S (bottom). (c) Tumor volume growth with various treatments. (d) Representative photographs of tumors collected from mice sacrificed after 16 day different treatments. Adapted with permission from ref. 17. Copyright 2016 Wiley-VCH. | |
4.2. Ag2Se QDs
Ag2Se QDs are another kind of narrow band gap (∼0.15 eV) semiconductor whose emission can also be tuned into the NIR-II region by tailoring their size. Without any heavy metals, Ag2Se QDs are ideal candidates for in vivo NIR-II FL imaging. In 2013, Wang and coworkers first reported Ag2Se QDs with an emission peak at 1300 nm.18 After surface modification, Ag2Se QDs exhibited bright NIR-II emission, good water solubility, high photostability and colloidal stability, and decent biocompatibility, which facilitate deep imaging of organs and blood vessels in vivo with high spatial resolution. Using 1-octanethiol as a ligand can balance the nucleation and growth of Ag2Se QDs, and tune the FL in the emission range from 1080 to 1330 nm in the NIR-II window. The obtained Ag2Se QDs can be transferred to the aqueous phase by ligand exchange, which showed promising potential for in vivo multicolor NIR-II FL imaging. Taking a multidentate polymer (MDP) as a capping agent is another method to form water-dispersible Ag2Se QDs. These MDP-capped Ag2Se QDs were synthesized in aqueous solution with high PL in the NIR-II region and good photostability. Due to the straightforward aqueous synthesis, the resultant QDs can be directly applied to biosystems for in vivo NIR-II FL imaging. Multimodal imaging has the potential for acquiring more information on the lesion area in vivo. Coupling NIR-II Ag2Se QDs with gadopentetate dimeglumine injection (Gd-DTPA), dual-modality T1-weighted magnetic resonance (MR) imaging and FL imaging were achieved. The Ag2Se-Gd QDs present promising potential as multifunctional contrast agents for multimodal imaging in clinical diagnosis and research.
4.3. Ag2Te QDs
Compared to Ag2S and Ag2Se, Ag2Te is a less explored member in the silver chalcogenide family. Ag2Te with lower thermal conductivity and higher electron mobility has been theoretically predicted to be efficient in thermoelectric applications. However, little effort on Ag2Te QDs was made for NIR-II FL imaging, due to their intrinsically less PL, complicated synthesis and low colloidal stability. To overcome these disadvantages, Yang et al. developed a simple one-pot aqueous synthesis to achieve fluorescent Ag2Te QDs using multivalent poly(maleic anhydride) homopolymers as stabilizers. The obtained water-dispersed Ag2Te QDs (3.8–4.7 nm) exhibited tunable PL emission (995–1068 nm) in the NIR-II region, which showed high PLQYs (13.1–15.2%), high photo-/colloidal stability, and ultralow cytotoxicity in vitro.19 With this improvement, Ag2Te QDs may possess promising potential for bioimaging application in the NIR-II window.
4.4. PbS QDs
PbS QDs have a large bulk exciton Bohr radius (∼20 nm), high emission intensity, size-based tunability, and a low bandgap and absorption edge in the NIR region, which have been widely employed for NIR-II biological imaging. However, the toxicity of lead ions can adversely affect organs, especially the nervous system, which is an unavoidable risk for both preparation and biological applications. Therefore, extensive research has been devoted towards decreasing the toxicity of PbS QDs. Chen et al. developed an environment-friendly and economical synthetic route to prepare whey-protein-capped PbS QDs using a recycle setup. Besides, the residual Pb2+ ions reacted with excess S2− ions and generated a nontoxic precipitate to avoid polluting the environment. After surface modification with the protein, PbS QDs demonstrated low toxicity both in vitro and in vivo, and featured bright FL and good stability in the NIR-II window for in vivo imaging. Ribonuclease-A (RNase-A) can rapidly encapsulate PbS QDs under microwave heating to achieve RNase-A@PbS QDs, which also emit FL in the NIR-II window. By controlling the synthesis temperature, PL spectra of the QDs can be tuned across the entire NIR-II window. Water-soluble QDs with a high QY (∼17.3%) were one of the brightest NIR-II emitters for in vivo imaging. Importantly, RNase-A@PbS QDs displayed negligible toxicity both in vitro and in vivo. Besides the methods of biomolecular coating, shell–core structures such as PbS/CdS/ZnS core/shell/shell QDs can also be used as stable and bright NIR-II probes that exhibit FL on matrix metalloprotease activity of the tumor microenvironment.20 This type of NIR-II fluorophore as an activatable modulator could be attached for photoexcited electron transfer quenching. The QDs allowed effective and rapid FL modulations based on the compact surface ligand modulator that included a methylene blue photoexcited electron transfer quencher. Upon enzyme activity, the modulator was optimized to afford a high activation signal surge with full enzyme accessibility. Optical phantom experiments proved the advantage of the PbS/CdS/ZnS QD based NIR-II probe over conventional dyes in the NIR region. The other type of shell–core structure based on PbS QDs is named PbS@CdS@SiO2@F-127 NPs, whose biocompatibility was good and toxicity in vivo was minimal, and PbS@CdS@SiO2@F-127 NPs can be utilized for real-time NIR-II FL microscopic imaging of the mouse brain in vivo and the architecture of blood vessels could be clearly visualized. Using a cation doping strategy to introduce transition metal Pb2+ ions into Ag2S nanocrystals, the obtained Pb-doped Ag2S QDs (Pb:Ag2S QDs) presented a renovated crystal structure and an enhanced optical performance. Besides, by adjusting the levels of Pb doping, Pb:Ag2S QDs (PLQYs up to 30.2%) with bright emission from 975 to 1242 nm were prepared without changing the ultra-small size (∼2.7–2.8 nm) of QDs. These works may set up novel green strategies to obtain various biocompatible QDs with heavy metal for potential biological applications. With a combination of the attractive properties of robust photostability, high brightness, and excellent biocompatibility, these new NIR-II emitting QDs are highly promising for diagnostic applications and accurate disease screening.
4.5. Carbon dots
With the attractive optical properties, low cost, small size, and excellent biocompatibility, carbon dots (CDs) have emerged as potential materials for biomedical applications. Recently, strong PL in the blue and green spectral regions of CDs has mostly been demonstrated with a maximum PLQY of 94%. However, the realization of strong emission at longer wavelengths of CDs remains difficult. Li et al. exploited molecules or polymers rich in sulfoxide/carbonyl groups, which were bound to the edges and the outer layers of the CDs, in order to tune the optical bandgap and promote the electron transitions under NIR excitation. The NIR absorption band and enhanced FL were both realized after the surface engineering of CDs. Upon modifying with poly(vinylpyrrolidone) in aqueous solution, the obtained CDs demonstrated efficient NIR FL imaging of the stomach of a living mouse. Besides, by exciting with a 1400 nm femtosecond laser, the CDs simultaneously generated two-photon-induced NIR emission and three-photon-induced red emission in dimethyl sulfoxide. This study represents the realization of NIR excitation and emission, as well as two-photon- and three-photon-induced FL of CDs excited in the NIR-II window, providing a rational design approach for the construction of CD-based NIR imaging agents for biomedical applications.21
However, several challenges of these QDs still remain such as high QYs without quenching by surface modification and controllable synthesis with defined size and shape. Besides, the current applications of these NIR-II QDs are mostly focused on bioimaging. Therefore, multifunctional applications of these NIR-II fluorophores should be explored, which may present more desirable properties in the practical aspects.
5. Lanthanide NPs for FL imaging and as drug carriers
Lanthanide elements exhibit complex and interesting properties in spectroscopy, which facilitates their use as PL probes for labeling biological application in multispectral windows. Compared to transition metal (3d) spectra, the 4f orbitals of lanthanides are buried beneath the 5p, 5d, and 6s orbitals; therefore, spectra arising from f–f transitions are narrow and insensitive to their environment, which also provides the lanthanide elements with rich energy level structures in the UV, VIS, and NIR spectral ranges. Lanthanide-doped inorganic NPs have been well demonstrated as anti-Stokes shift-based luminescent materials, which could absorb NIR light through the two-photon absorption process or upconversion process to emit VIS light. As lanthanide emissions come only from atomic transitions, they are extremely resistant to photobleaching. Besides, the various absorption and emission wavelengths, the low vibration energy losses and the independence from host materials together make lanthanide ions ideal spectral conversion materials. Lanthanide ions can be doped in a variety of solids in order to give them both upconversion and downconversion optical properties. Lanthanide-doped NPs with low toxicity, narrow band emission, long emission lifetimes, non-photobleaching and non-blinking are promising for biological imaging, such as analytical sensors, PDT and optical imaging. In particular, Nd3+, Yb3+, Ho3+, Er3+, and Tm3+-doped lanthanide NPs with efficient luminescence emission in the NIR-II region have already shown great potential for various biological applications.
Levy et al. first developed a series of lanthanide-doped imaging probes, which harnessed an energy-looping mechanism (in upconversion, excitation at NIR-II wavelengths is resonant with excited-state absorption transitions for populating excited states) to facilitate NIR-II excitation through resonance with excited-state absorption transitions.22 Using combinatorial screening and computational methods, Tm3+-doped NaYF4 NPs were selected as efficient energy-looping systems with continuous-wave excitation at 1064 nm and an emission at 800 nm. The energy-looping NPs (ELNPs) were imaged in cultured mammalian cells, and even can penetrate brain tissue without any auto-fluorescence. Shao et al. introduced a hybrid organic–inorganic system containing epitaxial core/shell/shell (CSS) structured NaYF4:Yb3+/X3+@NaYbF4@NaYF4:Nd3+(X = null, Er, Ho, Tm, or Pr) nanocrystals with ICG on the surface. This system produced narrow band emissions with a large Stokes-shift (>200 nm) in the NIR-II window, and the energy was transferred from light-harvesting ICG on the surface, via lanthanide ions in the shells, finally to the emitter X3+ in the core. In this nanosystem, ICG (no NIR-II emission itself) functioned as an excellent light-harvesting molecule on the surface that transferred light energy to the CSS structure inside for higher NIR-II FL intensity. The in vivo imaging further suggested that these ICG-sensitized CSS nanocrystals were suitable for deep tissue optical imaging in the NIR-II region. Polydopamine (PDA) as an endogenous substance was also applied to coat the lanthanide NPs. Using a reverse microemulsion method, the PDA shell endows the obtained NaYF4:Nd3+@NaLuF4@PDA with excellent biocompatibility and colloidal stability. NaYF4:Nd3+@NaLuF4@PDA exhibited remarkable performance for NIR-II PL imaging and X-ray computer tomography (CT) dual imaging of xenograft tumors in mice. Besides, because of the good photothermal conversion efficiency of PDA, the in vivo tumors could be completely eradicated by NIR irradiation. In another work, Tao et al. developed an optical imaging construct composed of rare earth lanthanide NPs coated with diblock copolymers, and doped with luciferase (LUC) and red fluorescent protein (RFP), which could generate NIR-I and NIR-II emissions together upon optical excitation.23 By injecting these multiple spectrally-unique NPs into mice bearing tumors of intraperitoneal dissemination of LUC+/RFP+ OVCAR-8 ovarian cancer cells, direct comparisons of imaging with NIR-II and NIR-I signals were, respectively, performed within the same animal (Fig. 4). In vivo NIR-II optical imaging presented more accurate detection of earlier and smaller tumor deposits, offering improved spatial contrast, enhanced sensitivity, and increased penetration depths compared to VIS and NIR-I fluorescent agents. Changing the host lattice of the NPs to some other cations can enhance their photophysical properties. For instance, monodisperse NaCeF4:Er3+/Yb3+ nanocrystals (NCs) exhibited intense NIR-II emission upon excitation at 980 nm, which enhanced the luminescence of Er3+ with a maximum NIR-II QY of 32.8% at 1530 nm, revealing the promising potential of these NaCeF4:Er3+/Yb3+ NPs in deep-tissue diagnosis. Sc-based probes (KSc2F7:Yb3+/Er3+) presented a strong NIR-II emission centered at 1525 nm. 10–15 nm-sized NIR-II luminescent NPs (CaF2:Nd3+ NPs) co-doped with Y3+ can enhance the luminescence of the NPs via breaking the aggregation of Nd3+. The incorporated Y3+ could dissolve in CaF2via occupying the Ca2+ sites to obtain a CaF2-YF3 solid solution; therefore, the Y3+ greatly enhanced the NIR-II PL of CaF2:Nd3+ NPs, which could be regarded as a superior imaging probe for biomedical imaging in vivo. Liu et al. designed a new class of Er3+ sensitized upconversion (NaErF4:Ln3+@NaYF4 UCNPs, Ln3+ = Ho3+ or Nd3+) NPs, which showed both excitation (1530 nm) and emission (1180 nm) in the NIR-II window for biosensing in vivo.24 A microneedle patch sensor was developed for dynamic detection of inflammation in vivo; it was based on ratiometric FL with effective NIR-II upconversion luminescence and H2O2 sensing organic probes based on the Fenton catalysis of Fe2+. Due to the large anti-Stokes shifting, low tissue scattering and auto-fluorescence of the NIR-II upconversion emission, inflammation was dynamically evaluated at very high resolution (200 μm × 200 μm) in vivo. Using Mn2+-doped NaNdF4 ultrasmall nanocrystals (NCs), T1- and T2-weighted magnetic resonance imaging (MRI), NIR-II FL imaging and CT imaging were combined to enable high-performance multimodal MRI/NIR-II/CT imaging with single NaNdF4:Mn NCs, which were demonstrated as a bright nanoprobe for multimodal imaging of human mesenchymal stem cells both in vitro and in vivo. Lanthanide-based (NaGdF4:5%Nd@NaGdF4) NPs could also be used as nanocarriers to retain drugs and withstand the strongly acidic condition of the gastrointestinal tract. Moreover, these nanocarriers can be tracked semi-quantitatively in real time. Meanwhile, the drug release can be monitored in vivo by measuring the NIR-II FL signals. The NIR-II FL imaging results indicated that the nanocarriers showed a prolonged residence time in the gastrointestinal tract (up to 72 h), releasing up to 62% of the drugs. Significantly, minimal deposition of the nanocarrier was found in non-target main organs, such as the kidney, spleen and liver.
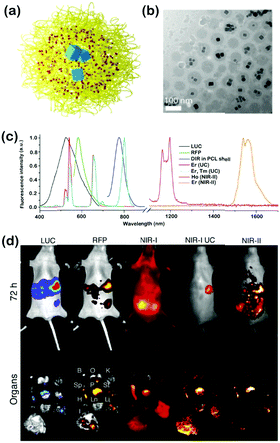 |
| Fig. 4 (a) Illustration depicting core–shell NPs composed of PEO-b-PCL diblock copolymers (yellow), the organic NIR-I fluorophore DiR (red) and LNPs (blue). (b) Cryo-TEM image of core–shell NPs (DiR-Er/PEO-PCL) in aqueous suspension. (c) Normalized FL spectra of intrinsic reports (LUC and RFP) as well as emissive components of various core–shell formulations. (d) Images taken of a mouse after implantation of LUC+/RFP+ OVCAR-8 ovarian cancer cells and 72 h after IP injection of untargeted core–shell NPs (DiR-Er, Tm/PEO-PCL). Top row: in vivo images of intrinsic reporters (LUC and RFP) as well as various emissive components of core–shell NPs (DiR and LNPs). Bottom row: ex vivo images were obtained after mouse sacrifice and corresponded to the detection of the same reporters in excised organs, including the bladder (B), ovaries (O), kidneys (K), spleen (Sp), pancreas (P), stomach (St), heart (H), lung (Ln), liver (Li) and intestines (I). Adapted with permission from ref. 23. Copyright 2017 Elsevier group. | |
These findings together provide novel insights into the applications of bioimaging and therapeutic strategies based on lanthanide nanomaterials. These NPs working in the NIR-II range as potential imaging agents have yielded high intensity signals. However, to improve the utility of the lanthanide NPs, more advances are needed to overcome the intrinsically low photon absorption and PLQY of lanthanide elements.
6. Other inorganic NPs for imaging and phototherapy
Although the long term toxicity and biocompatibility of many inorganic materials have not been determined in the clinic, they have the advantages of high drug-loading rates and controlled drug release, easy surface modification, wide light absorption, and high phototherapy efficiency, which make them suitable for use in various biomedicine fields. Common inorganic NPs such as mesoporous silica, magnetic iron NPs, and gold nanomaterials usually have the ability to combine multiple functions (drug delivery, imaging diagnosis, PDT, PTT etc.) into one platform; therefore much more attention has been paid towards developing their multiple functions in the NIR-II window. In particular, with excellent photophysical and easy chemical modification properties, various inorganic NPs have been developed for NIR-II optical imaging and phototherapy.
6.1. Gold nanomaterials
Highly tunable and localized surface plasmon resonance (LSPR) is a key factor in the plasmonics of a nanostructured material (Au, Ag, or Cu metal) to red shift its spectrum into the NIR-II region. In the meantime, the self-assembly process is able to generate uniform and solution-processable materials with interesting plasmonic properties. Fang et al. used a wet Ag ion soldering and dry thermal sintering technique to produce dimer-derived water soluble nanostructures with accurately engineered charge-transfer plasmons.25 A solid state sintering process was used to build a conductive junction between two Au NPs with a silica shell which can isolate the Au NPs from thermal treatment. The sintered dimers were water soluble, and gradually shifted charge-transfer plasmons to the NIR-II region. Upon silica removal, the nanostructures could be grafted by DNA for more surface functionalities. This process can also be adaptable to DNA-linked Au dimer-derived NPs via DNA-guided sintering and soldering. In another work based on Au NPs, Zhou et al. developed a series of blackbody materials named hyperbranched Au plasmonic blackbodies (AuPBs) with sizes of <50 nm. The AuPBs were obtained in a surfactant-free and seedless approach with the use of mussel-inspired dopamine. Strong intraparticle plasmonic coupling on branches in close proximity resulted in uniform and intense broadband absorption in the wavelength range of 400–1350 nm. The blackbody absorption made the compact AuPBs exhibit a photothermal efficiency of >80% in both the NIR-I and NIR-II windows, making them rare broadband theranostic probes for integrated PA imaging and PTT.26 Gold nanostars (Au NSs) with metal-enhanced FL were also explored for the application in both the NIR-I and NIR-II windows. NIR-I/NIR-II fluorophores were incorporated into monolayers of Au NSs with tunable plasmonic responses, and the enhancement factors were up to 50-fold in the NIR-II region, suggesting that this system showed promise as bright probes for future NIR-II biosensing and bioimaging in the clinic.
6.2. Bismuth-based NPs
Bismuth ferrite harmonic NPs (BFO HNPs) can expand human skeletal muscle-derived stem cells (hMuStem cells) in vitro, which is an attractive therapeutic approach for Duchenne muscular dystrophy (DMD). BFO HNPs proved to have the full advantage of deep imaging penetration by multiphoton microscopy in the NIR-II window, and the individual HNP-labeled hMuStem cells can be detected in skeletal muscle tissue after intramuscular injection. Copper bismuth sulfide nanorods (Cu3BiS3 NRs) presented broad and strong absorption ranging from UV to NIR wavelengths, which was used as a photothermal agent driven using a 1064 nm laser with a high photothermal conversion efficiency of 40.7% in the NIR-II window. The prepared PEGylated Cu3BiS3 NRs could also be used as PA imaging and CT imaging agents with their strong NIR absorption and X-ray attenuation coefficients. Thus, the PEGylated Cu3BiS3 NRs were synergistic thermoradiotherapeutic agents to enhance the efficacy of radiotherapy guided by multimodal imaging. Another multifunctional theranostic agent with peptide (LyP-1)-labeled semimetal NPs of bismuth (Bi-LyP-1 NPs) was facilely synthesized using oleylamine as the reducing agent, and the NPs exhibited high tumor accumulation in conjugation with the tumor-targeting peptide LyP-1. Bi-LyP-1 NPs with the ability to absorb both ionizing radiation and NIR-II laser radiation allowed dual-modal CT/PA imaging and efficient NIR-II PTT/radiotherapy of tumors. With low toxicity and fast clearance, such multifunctional NPs are efficient cancer theranostic agents that can facilitate the future use of semimetal NPs for biomedical applications.
6.3. Mesoporous NPs
Using a magnesiothermic reduction process, Yu et al. synthesized a class of non-stoichiometric hollow silicon oxide NPs (H-SiOx NPs).27 These black NPs generated a desired LSPR in the NIR-II window with a photothermal conversion efficiency of 48.6% at 1064 nm, which was an outstanding performance among the semiconductor and noble metal-based NPs for NIR-II PTT. In addition, H-SiOx NPs exhibited excellent PA imaging properties in vivo, which could be used for efficient cancer treatment by irradiation with a 1064 nm laser. The findings demonstrated the existence of LSPR in H-SiOx NPs with a low-toxicity degradation pathway, and provided new insights into understanding the role of new semiconductor NPs in nanomedicine. The mSiO2@Gd3Ga5O12:Cr33+,Nd3+ (mSiO2@GGO) NPs as another multifunctional nanoplatform showed excellent NIR-II luminescence (1067 nm) under 808 nm excitation, which were designed for multimodal cancer FL imaging, MRI, drug delivery and tumor therapy. Mesoporous carbon nanospheres with broadband and intense absorption in the UV-VIS-NIR region (300–1400 nm) were explored as a multifunctional platform for NIR-II PA imaging, and the porous structure can also serve as a drug carrier which can release the drug by pH and external light.
6.4. MXene and TiO2 nanocrystals
Lin et al. explored a new kind of two-dimensional (2D) metal carbide (MXene), niobium carbide (Nb2C) nanosheets (NSs), which presented high efficiency for in vivo PTT of mouse tumors in both the NIR-I and NIR-II regions with high photothermal conversion efficiencies (36.4% and 45.65% in NIR-I and NIR-II, respectively) and high photothermal stability (Fig. 5). Besides, the Nb2C NSs presented high biocompatibility, low phototoxicity, and enzyme-responsive biodegradability to human myeloperoxidase, which broadened the application prospects of 2D MXenes in the phototherapy of cancer.28 Han et al. created an oxygen-deficient TiO2−x layer on the surfaces of TiO2 nanocrystals and formed a crystalline-disordered core/shell structure (TiO2@TiO2−x) semiconductor for synergistic sono-/photoinduced tumor therapy. The oxygen-deficient TiO2−x layer on the surface with abundant oxygen defects can facilitate the separation of holes (h+) and electrons (e−) from the energy-band structure upon external ultrasound irradiation, which significantly improved the efficacy of tumor sonodynamic therapy. TiO2@TiO2−x also showed high photothermal conversion efficiency (39.8%) under NIR-II (1064 nm) irradiation for enhanced PTT.29
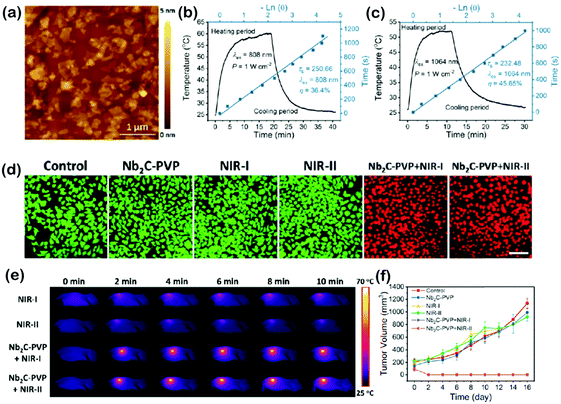 |
| Fig. 5 (a) Typical AFM image of Nb2C NSs. (b and c) Calculation of the photothermal conversion efficiency at 808 nm (NIR-I) and 1064 nm (NIR-II) of Nb2C NSs. (d) Confocal fluorescence imaging of Nb2C-PVP-induced photothermal ablation (1.0 W cm−2, 40 μg mL−1) after various treatments. Images shared the same scale bar (50 μm). (e) The corresponding IR thermal images at the tumor site of 4T1-tumor-bearing mice in different groups during laser irradiation at different time intervals. (f) Time-dependent tumor growth curves after different treatments. Adapted with permission from ref. 28. Copyright 2017 American Chemical Society. | |
Inorganic NPs are powerful tools for diagnosis of diseases, monitoring drug delivery, and phototherapy. However, the challenges of these inorganic NPs remain in terms of their biocompatibility, long-term toxicity, targeting efficiency, and stability for clinical translation, and issues of the functionalized inorganic NPs need to be addressed. There is a great requirement for effective surface functionalization chemistry, to achieve bioaffinity, ligand diversity, and stable bioactive interfaces.
7. Organic dyes for FL imaging and imaging-guided surgery
Donor–acceptor–donor (D–A–D) structured small molecule fluorophores with the advantages of good biocompatibility, minimal cellular toxicity, easy chemical modification, and favorable excretion pharmacokinetics in the biological system are more desirable than inorganic agents for potential clinical NIR-II diagnostic imaging and therapeutic applications. In general, highly tunable organic dye architecture is built based on a strong electron acceptor like benzo[1,2-c:4,5-c′]bis([1,2,5]thiadiazole) (BBTD), and strong electron donors can be wired to the acceptor through π-spacers to lower the entire energy gap HOMO and LUMO levels, which may further allow wavelength tuning of absorption and emission peaks in the NIR-II region. However, free organic molecules usually lack tumor-targeting and water solubility properties. To solve these problems, the strategies of PEGylation or fabrication of nanometric forms may preclude the organic dyes from being rapidly cleared through the kidney or liver, which can extend the circulation time in blood. In addition, these nano-sized particles also easily accumulate in tumor tissues based on the EPR effect.
7.1. BBTD-based molecules
BBTD as a kind of strong electron acceptor was mostly used for the design of D–A–D structured organic molecules, and many NIR-II dyes based on BBTD have been successfully synthesized in the past two years (Fig. 6). Dai et al. first reported a synthetic 970 Da NIR-II organic fluorophore (CH1055) based on the acceptor BBTD and triphenylamine (TPA) as the donor, which outperformed the clinically approved ICG in resolving mouse sentinel lymphatic mapping near a tumor and lymphatic vasculature, and can be rapidly excreted through the kidneys (90% excreted within 24 h).30 Besides, high uptake of the CH1055-PEG dye could also be observed in brain tumors in vivo, indicating the advantage of deep penetration. After conjugation with anti-EGFR antibody, the CH1055 dye demonstrated targeted molecular imaging of tumors in mice. Encouragingly, the superior tumor-to-background signal ratio facilitated precise imaging-guided tumor surgery. However, CH1055-PEG holds a rather low PLQY (0.3%); therefore, the authors further designed a NIR-II molecular fluorophore (IR-E1) with thiophene-based units as the donor to afford a lower bandgap, which exhibited excellent photostability, water solubility, fast renal excretion, high biocompatibility and a higher QY (∼0.7%). This NIR-II dye was used to perform noninvasive NIR-II imaging in the mouse brain via the tail vein, which assisted in investigating the traumatic brain injury (TBI) in mice. The NIR-II imaging with high-spatial and temporal resolution could directly help in visualizing the fluorophore leakage and cerebral hypoperfusion and trapping the injured brain tissue, which would further help in realizing the dynamics of neurovascular changes following TBI (Fig. 7).31 By changing the carboxylic groups of CH1055 to sulfonic acid, a completely water-soluble organic NIR-II dye (CH-4T) can be obtained, which could further form supramolecular assemblies with plasma proteins in serum and produce a great increase in fluorescence brightness (QY, ∼5%). The bright molecular complex presented fast video-rate imaging in the NIR-II window at a pace of 50 frames-per-second for resolving mouse cardiac cycles. To reduce the conjugated backbone interactions and improve FL characteristics in both aqueous solutions and organic solvents, a molecular fluorophore (IR-FE) was constructed with a structure named a shielding unit–donor–acceptor–donor–shielding unit (S–D–A–D–S), whose BBTD was the acceptor, 3,4-ethylenedioxy thiophene (EDOT) was the donor, and dialkyl fluorene was the shielding unit. Theoretical calculations revealed that EDOT allowed a less delocalized LUMO and larger backbone distortion, and tuned the electrostatic potential distribution. Dialkyl fluorene in the conjugated backbone can shield the backbone from aggregation. Therefore, IR-FE presented an enhanced PLQY of 31% in toluene solution, which was reported to be the brightest NIR-II dye in organic solution so far. By conjugating a novel peptide (named CP) with the NIR-II fluorescent probe (IRT), the obtained peptide-dye (CP-IRT dye) could target to the tumor stem cell biomarker CD133 for molecular tumor imaging. This CP-IRT probe was renally excreted, and the urethra of the mice was imaged with NIR-II FL imaging for the first time. Linking two hormones to nonoverlapping NIR-II fluorophores can facilitate two-color imaging of different receptors, which demonstrated an unprecedented multicolor of live molecular imaging in the NIR-II window. Besides, using a home-built NIR-II set-up, 900 μm-deep 3D organ imaging was performed without any tissue clearing techniques. The deep tissue imaging of different specific receptors in vivo may allow the development of noninvasive molecular imaging to distinguish normal and neoplastic organs, and the developed NIR-II FL microscopy may become a novel imaging technique for deep tissue 3D imaging without any tissue clearing treatment or physical sectioning. Feng et al. reported the conjugation of CH1055 to a follicle stimulating hormone (FSH) to form a targeted FSH-CH for FL imaging ovaries and tested in mice.32 This work provides active targeting imaging in vivo using a ligand-conjugated NIR-II fluorophore, which may open up the development of non-invasive NIR-II molecular imaging of diverse target cells in vivo. Sun et al. designed a series of NIR-II dyes with D–A–D structures, which incorporates a thiophene spacer, to design and synthesize 4 different fluorescent compounds (Q1, Q2, Q3, and Q4), and studied the relationships of their absorption FL properties with structures. The most promising Q4 was further conjugated with a small bombesin peptide to prepare a SCH1100 probe for gastrin-releasing peptide receptors (GRPR), which can be used for in vivo NIR-II imaging of prostate cancer.33 Through different chemical strategies, a series of biocompatible and water-soluble NIR-II probes: SDH, SXH, and H1 NPs were prepared, which demonstrated active and passive tumor targeting abilities together. With the high resolution imaging of the whole body of living mice and blood vessels of tumors, NIR-II imaging-guided precise surgery on sentinel lymph nodes (SLNs) was performed for the first time. Encapsulating a NIR-II dye into phospholipid vesicles (DSPE-mPEG5000) to prepare a nanoprobe called CQS1000 can dynamically visualize and monitor many physiological and pathological conditions in circulatory systems, such as angiogenesis of tumors, lymphatic drainage and routing, vascular deformities like arterial thrombus formation, and ischemia with high temporal and spatial resolution. Using nanoprecipitation, D–A chromophore based NPs (DAPs) were formed as a dual-modal imaging contrast agent with strong absorption in the NIR-I window and a strong FL emission peak in NIR-II. These multifunctional DAPs’ surfaces were available for bioconjugation of an EGFR antibody (Ac-Cys-ZEGFR:1907) to actively target EGFR-positive cancers. Using base-catalyzed double addition of thiols into a propiolamide scaffold, Sun et al. developed a novel dual-modal imaging platform (68Ga-SCH2), which allowed for selective and efficient assembly of thiol units. The small molecule based on avb3-targeted NIR-II FL imaging and positron emission computed tomography (PET) imaging signals was concisely generated and evaluated in U87MG xenograft bearing mice; it delineated the tumor, and dual-modal imaging-guided surgery was achieved. By integrating NIR-II imaging and proteomics to gain quantitative insight into molecular targets for HCC treatment, a minichromosome maintenance 2 (MCM2)-targeted NIR-II probe (CH1055-MCM2) was obtained to determine the novel targets of HCC for both imaging and therapy.
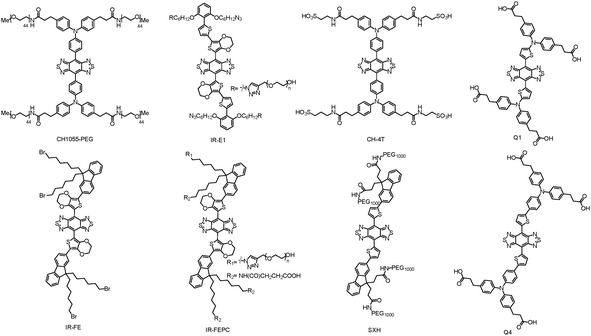 |
| Fig. 6 Representative BBTD-based organic dyes. | |
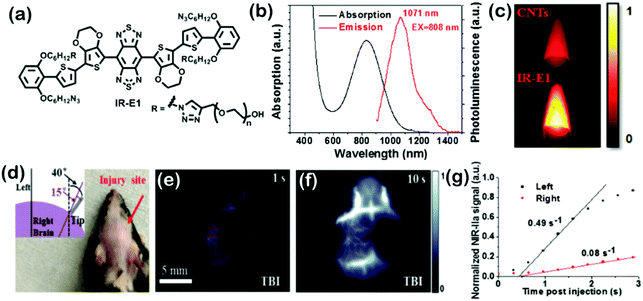 |
| Fig. 7 (a) Molecular structure of IR-E1. (b) UV-vis absorption and FL emission spectra of an aqueous solution of IR-E1. (c) FL imaging of a typical carbon nanotube suspension and an IR-E1 solution upon the same excitation with an 808 nm laser. (d) Photograph of a mouse with the scalp shaved following TBI on the right side of the head. Inset: Schematic of angles of the employed metal tip that impact to the mouse cranium at a speed of 5.3 mm s−1. (e and f) Dynamic NIR-II image frames recorded at indicated time points post injection of IR-E1 (10 mg kg−1) showing reduced blood flow in the injured right hemisphere. (g) NIR-II signal in the left (contralateral) and right (ipsilateral) cerebral hemispheres (normalized by the signal in the left side at 10 s p.i. when the signal began to plateau) of the TBI mouse versus time. Adapted with permission from ref. 31. Copyright 2016 Wiley-VCH. | |
7.2. Other organic dyes
Besides BBTD-based molecules, some other organic dyes with D–A–D structure also present excellent NIR-II optical properties, which have been studied for biomedical imaging. Very recently, Xu et al. devised an activatable NIR-II probe for visualizing colorectal cancers, which was rationally designed with a boron-dipyrromethene dye to generate NIR-II emission in the presence of H2S. The probe displayed H2S-activated ratiometric FL and exhibited NIR-II emission in the ∼900–1300 nm region. Using the activating and targeting specific probe could deep-tissue image H2S-rich colon cancer cells to accurately identify colorectal tumors in animal models. Such a design was used to construct FL probes with multi-wavelength ratiometric imaging to acquire high precision measurements.34 In another work, Tang et al. developed dual-pathological-parameter cooperatively activatable IR-1061 NIR-II FL nanoprobes using disulfide bonds and hyaluronic acid chains as the “double locks” to maintain the FL quenched aggregation state for performing specific in vivo tumor imaging. FL emission was exhibited when the “double locks” were opened by simultaneously reacting with the overexpressed thiols and hyaluronidase in tumors (dual keys).35 Li et al. synthesized a small molecule fluorophore (FD-1080) with both excitation and emission in the NIR-II region for in vivo imaging. A heptamethine structure was introduced into FD-1080 for bathochromically-shifting the absorption and emission into the NIR-II region. The FL QY of FD-1080 was 0.31%, which increased to 5.94% in fetal bovine serum (FBS). FD-1080 was capable of realizing high-resolution brain vessel bioimaging and deep-tissue hindlimb vasculature, and quantifying the respiratory rate by dynamic imaging in the respiratory craniocaudal motion of the liver in vivo.36
8. SPNs for PA imaging and phototherapy
As the photophysical properties of the polymers are determined by the molecular structures, molecular engineering makes the possibility of photophysical processes for SPNs to obtain different optical absorption and emission properties in the NIR region. In particular, increasing the π-conjugation length by designing quinoid stabilized D–A–D polymers has been verified as an effective strategy to reduce the bandgap, which is beneficial for the bathochromic shift of SPNs.37 Meanwhile, the nanoformulation can alternate the intramolecular and intermolecular structures of SPNs for various biological applications such as activatable PA imaging,38 phototherapy,39 chemiluminescence imaging,40 and photoactivation.41 Besides, with the advantages of high photostability, large absorption coefficients, and good biocompatibility, SPNs present promising potential as NIR-II materials. For instance, NIR-II SPNs have achieved ultrasensitive molecular imaging in deep-tissues with strong charge-transfer backbones to convert photoenergy into mechanical acoustic waves for PA imaging. So far, SPNs as molecular probes have been developed to image physiological indexes (pH and temperature), biomarkers (biothiols and ROS), and tumors in vivo.42 Moreover, SPNs also permit light-modulated therapeutic interventions with high photothermal conversion efficiencies, which have been well applied to photothermal sensors to regulate biological analyses such as gene expression, enzymatic activity, and protein ion channels.43 For further functions with tumor-homing and biodistribution abilities, SPNs can also be doped with other organic/inorganic agents for amplified PTT or PDT.44 Li et al. used PEDOT NPs with broad absorption from 700 to 1250 nm, a suitable nanosize of 17.2 nm, and a photothermal conversion efficiency of 71.1% to conjugate ICG as an effective bactericidal agent mediating dual-modal photothermal and photodynamic sterilization in both the NIR-I and NIR-II windows.45 To further obtain bacteria targeting properties, PEDOT:ICG was conjugated with PEG and glutaraldehyde (GTA). PEDOT:ICG@PEG-GTA presented excellent synergistic bactericidal efficiency of PDT and PTT after irradiation with NIR light (808 nm and 1064 nm). Cao et al. developed a narrow bandgapped D–A–D polymer with 2,2′-bithiophene (DT) as the donor part and thiophene-fused benzodifurandione-based oligo(p-phenylenevinylene) as the acceptor part, and they further exploited it as a PTT agent with robust photostability and high extinction efficiency in the NIR-II region.46 Pu et al. reported a broadband absorbing photoacoustic (PA) contrast agent based on SPNs (SPN-II) and applied it for PA imaging in the NIR-II region. Because of the weaker background PA signals of biological tissues in the NIR-II window, the SNR of SPN-II resulted PA imaging at 1064 nm was 1.4-fold stronger than that at 750 nm at a depth of 3 cm. This study for the first time introduced the organic contrast agent applicable for PA imaging in both the NIR-II and NIR-I windows (Fig. 8).47 After this work, they further designed an organic semiconducting copolymer nano-agent (SPNI–II) with two distinct segments in the NIR-I and NIR-II windows, and utilized it for tumor PTT. The nano-agent with dual peaks can, respectively, absorb NIR light at 808 and 1064 nm. SPNI–II enabled superior deep-tissue PTT with a photothermal conversion efficiency of 43.4% at 1064 nm than that at 808 nm. Doping with a NIR dye of SPNI–II, it can achieve FL imaging-guided NIR-II PTT in vivo.48 Cao et al. synthesized a thieno-isoindigo derivative-based semiconducting polymer and formulated it into PEGylated NPs, which exhibited a strong absorption ability in the NIR-II window, high photothermal conversion efficacy and good photostability.49 Moreover, with the enhanced PA imaging of tumors in living mice, the NPs showed great probability for in vivo PA imaging-guided NIR-II PTT. Guo et al. formed a poly(benzodithiophene-alt-benzobisthiadiazole) semiconducting polymer with good biocompatibility, high imaging contrast and excellent photostability, which has been successfully used to generate NIR-II PA images of orthotopic brain tumors.50
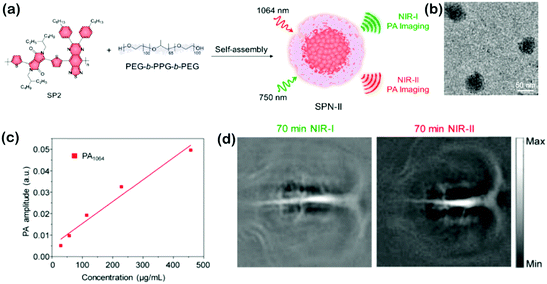 |
| Fig. 8 (a) Schematic of preparation of SPN-II via the nanoprecipitation method. (b) TEM image of SPN-II. (c) PA amplitude of SPN-II as a function of concentration at 1064 nm. R2 = 0.96131. (d) Representative PA images of the rat cortex at 70 min post injection of SPN-II at 750 and 1064 nm. Adapted with permission from ref. 47. Copyright 2017 American Chemical Society. | |
Relative to previous uses of these organics in NIR-II imaging and phototherapy, studies further show the breadth and depth of their applications and powerful vitality in biomedical and biological applications. Although encouraging progress has been achieved, limitations that prevent these semiconducting polymers from further development and clinical applications still exist, including undesirable interactions and self-aggregation with hydrophobic substrates, low QYs of most NIR-II polymers, varying QYs and uncertain MWs between batches, low killing selectivity to cancer cells, potential toxicity in vivo, and impotent function in biological fluids.
9. Conclusions
Here, we have reviewed recent optical nano-agents for various biomedical applications in the NIR-II window. Nano-agents such as SWNTs, QDs, rare-earth doped NPs, small organic molecule-based fluorophores, and semiconducting polymer NPs that absorb or emit light in the NIR-II window provide advantages of higher feature contrast at greater imaging depths, better spatial resolution, higher signal-to-background ratios, reduced light scattering, and real-time imaging compared to the VIS and NIR-I windows. As a result, these NIR-II agents have been developed to achieve maximal depths of signal penetration, enhanced contrast resolution, and locally minimized absorption by water for in vivo optical imaging, phototherapy, and various related biomedical applications. Although these NIR-II agents have demonstrated promising potential in basic biomedical research, the actual implementation for clinical applications still requires extra efforts from interdisciplinary subjects. Above all, through advanced chemistry and nano-design, more delicate NIR-II NPs may achieve non-biotoxicity, high PLQYs, good photostability, favourable bioavailability and biodegradability, and multifunctionality for high-efficiency phototheranostics. Besides, using various chemical and biological designs to link targeting function groups to NIR-II nano-agents is an effective and promising method to realize accurate biomedical treatments. In addition, multimodal imaging (FL imaging, PA imaging, MRI, PET, etc.) and therapy (PDT, PTT, chemotherapy, surgery, radiotherapy, etc.) may be combined into one nano-platform to provide more diagnostic information and higher treatment efficiency, which may generate an outstanding synergistic effect. More importantly, comprehensive animal studies on long-term bio-safety, immune reactions and pharmacokinetics are essential to realize real clinical translation. It is also needed to consider the possible risks associated with NIR-II nano-agents and their complicate interactions with biological systems of some other animals such as rabbits and monkeys. Overall, these optical nano-agents studied in the past decade for various biomedical applications in the NIR-II region have pointed out a meaningful development direction of optical medicine, which not only influences basic biomedical research studies, but also presents a great possibility for clinical phototheranostics in the near future.
Conflicts of interest
The authors declare no conflict of interest.
Acknowledgements
This work was supported by the NNSF of China (61525402, 61775095), the Jiangsu Provincial Key Research and Development Plan (BE2017741), the Jiangsu Provincial Natural Science Foundation (BK20180136, BK20160051), the Fundamental Research Funds for the Central Universities (021414380350), and the Nanjing Foundation for Development of Science and Technology (2017sc512031, 201605042).
References
- H. S. Peng and D. T. Chiu, Chem. Soc. Rev., 2015, 44, 4699–4722 RSC.
- H. Zhang, D. Salo, D. M. Kim, S. Komarov, Y.-C. Tai and M. Y. Berezin, J. Biomed. Opt., 2016, 21, 126006 CrossRef PubMed.
- G. Chen, I. Roy, C. Yang and P. N. Prasad, Chem. Rev., 2016, 116, 2826–2885 CrossRef CAS PubMed.
- C. Xie, X. Zhen, Q. Lei, R. Ni and K. Pu, Adv. Funct. Mater., 2017, 27, 1605397 CrossRef.
- Z. L. Kevin Welsher, Dan Daranciang and Hongjie Dai, Nano Lett., 2008, 8, 586–590 CrossRef PubMed.
- E. Thimsen, B. Sadtler and M. Y. Berezin, Nanophotonics, 2017, 6, 1043–1054 CAS.
- Y. Jiang and K. Pu, Acc. Chem. Res., 2018, 51, 1840–1849 CrossRef CAS PubMed.
- J. Li, J. Rao and K. Pu, Biomaterials, 2018, 155, 217–235 CrossRef CAS PubMed.
- K. Welsher, Z. Liu, S. P. Sherlock, J. T. Robinson, Z. Chen, D. Daranciang and H. Dai, Nat. Nanotechnol., 2009, 4, 773–780 CrossRef CAS PubMed.
- J. D. Harvey, P. V. Jena, H. A. Baker, G. H. Zerze, R. M. Williams, T. V. Galassi, D. Roxbury, J. Mittal and D. A. Heller, Nat. Biomed. Eng., 2017, 1, 0041 CrossRef PubMed.
- P. V. Jena, D. Roxbury, T. V. Galassi, L. Akkari, C. P. Horoszko, D. B. Iaea, J. Budhathoki-Uprety, N. Pipalia, A. S. Haka, J. D. Harvey, J. Mittal, F. R. Maxfield, J. A. Joyce and D. A. Heller, ACS Nano, 2017, 11, 10689–10703 CrossRef CAS PubMed.
- M. P. Landry, H. Ando, A. Y. Chen, J. Cao, V. I. Kottadiel, L. Chio, D. Yang, J. Dong, T. K. Lu and M. S. Strano, Nat. Nanotechnol., 2017, 12, 368–377 CrossRef CAS PubMed.
- J. Dong, D. P. Salem, J. H. Sun and M. S. Strano, ACS Nano, 2018, 12, 3769–3779 CrossRef CAS PubMed.
- J. T. D. Bonis-O'Donnell, R. H. Page, A. G. Beyene, E. G. Tindall, I. R. McFarlane and M. P. Landry, Adv. Funct. Mater., 2017, 27, 1702112 CrossRef.
- A. G. Godin, J. A. Varela, Z. Gao, N. Danne, J. P. Dupuis, B. Lounis, L. Groc and L. Cognet, Nat. Nanotechnol., 2017, 12, 238–243 CrossRef CAS PubMed.
- G. Hong, J. T. Robinson, Y. Zhang, S. Diao, A. L. Antaris, Q. Wang and H. Dai, Angew. Chem., Int. Ed., 2012, 51, 9818–9821 CrossRef CAS PubMed.
- C. Song, Y. Zhang, C. Li, G. Chen, X. Kang and Q. Wang, Adv. Funct. Mater., 2016, 26, 4192–4200 CrossRef CAS.
- B. Dong, C. Li, G. Chen, Y. Zhang, Y. Zhang, M. Deng and Q. Wang, Chem. Mater., 2013, 25, 2503–2509 CrossRef CAS.
- M. Yang, R. Gui, H. Jin, Z. Wang, F. Zhang, J. Xia, S. Bi and Y. Xia, Colloids Surf., B, 2015, 126, 115–120 CrossRef CAS PubMed.
- S. Jeong, J. Song, W. Lee, Y. M. Ryu, Y. Jung, S. Y. Kim, K. Kim, S. C. Hong, S. J. Myung and S. Kim, Nano Lett., 2017, 17, 1378–1386 CrossRef CAS PubMed.
- D. Li, P. Jing, L. Sun, Y. An, X. Shan, X. Lu, D. Zhou, D. Han, D. Shen, Y. Zhai, S. Qu, R. Zboril and A. L. Rogach, Adv. Mater., 2018, 30, 1705913 CrossRef PubMed.
- E. S. Levy, C. A. Tajon, T. S. Bischof, J. Iafrati, A. Fernandez-Bravo, D. J. Garfield, M. Chamanzar, M. M. Maharbiz, V. S. Sohal, P. J. Schuck, B. E. Cohen and E. M. Chan, ACS Nano, 2016, 10, 8423–8433 CrossRef CAS PubMed.
- Z. Tao, X. Dang, X. Huang, M. D. Muzumdar, E. S. Xu, N. M. Bardhan, H. Song, R. Qi, Y. Yu, T. Li, W. Wei, J. Wyckoff, M. J. Birrer, A. M. Belcher and P. P. Ghoroghchian, Biomaterials, 2017, 134, 202–215 CrossRef CAS PubMed.
- L. Liu, S. Wang, B. Zhao, P. Pei, Y. Fan, X. Li and F. Zhang, Angew. Chem., Int. Ed., 2018, 57, 7518–7522 CrossRef CAS PubMed.
- L. Fang, Y. Wang, M. Liu, M. Gong, A. Xu and Z. Deng, Angew. Chem., Int. Ed., 2016, 55, 14296–14300 CrossRef CAS PubMed.
- J. Zhou, Y. Jiang, S. Hou, P. K. Upputuri, D. Wu, J. Li, P. Wang, X. Zhen, M. Pramanik, K. Pu and H. Duan, ACS Nano, 2018, 12, 2643–2651 CrossRef CAS PubMed.
- X. Yu, K. Yang, X. Chen and W. Li, Biomaterials, 2017, 143, 120–129 CrossRef CAS PubMed.
- H. Lin, S. Gao, C. Dai, Y. Chen and J. Shi, J. Am. Chem. Soc., 2017, 139, 16235–16247 CrossRef CAS PubMed.
- X. Han, J. Huang, X. Jing, D. Yang, H. Lin, Z. Wang, P. Li and Y. Chen, ACS Nano, 2018, 12, 4545–4555 CrossRef CAS PubMed.
- A. L. Antaris, H. Chen, K. Cheng, Y. Sun, G. Hong, C. Qu, S. Diao, Z. Deng, X. Hu, B. Zhang, X. Zhang, O. K. Yaghi, Z. R. Alamparambil, X. Hong, Z. Cheng and H. Dai, Nat. Mater., 2016, 15, 235–242 CrossRef CAS PubMed.
- X. D. Zhang, H. Wang, A. L. Antaris, L. Li, S. Diao, R. Ma, A. Nguyen, G. Hong, Z. Ma, J. Wang, S. Zhu, J. M. Castellano, T. Wyss-Coray, Y. Liang, J. Luo and H. Dai, Adv. Mater., 2016, 28, 6872–6879 CrossRef CAS PubMed.
- Y. Feng, S. Zhu, A. L. Antaris, H. Chen, Y. Xiao, X. Lu, L. Jiang, S. Diao, K. Yu, Y. Wang, S. Herraiz, J. Yue, X. Hong, G. Hong, Z. Cheng, H. Dai and A. J. Hsueh, Chem. Sci., 2017, 8, 3703–3711 RSC.
- Y. Sun, C. Qu, H. Chen, M. He, C. Tang, K. Shou, S. Hong, M. Yang, Y. Jiang, B. Ding, Y. Xiao, L. Xing, X. Hong and Z. Cheng, Chem. Sci., 2016, 7, 6203–6207 RSC.
- G. Xu, Q. Yan, X. Lv, Y. Zhu, K. Xin, B. Shi, R. Wang, J. Chen, W. Gao, P. Shi, C. Fan, C. Zhao and H. Tian, Angew. Chem., Int. Ed., 2018, 57, 3626–3630 CrossRef CAS PubMed.
- Y. Tang, Y. Li, X. Hu, H. Zhao, Y. Ji, L. Chen, W. Hu, W. Zhang, X. Li, X. Lu, W. Huang and Q. Fan, Adv. Mater., 2018, 30, 1801140 CrossRef PubMed.
- B. Li, L. Lu, M. Zhao, Z. Lei and F. Zhang, Angew. Chem., Int. Ed., 2018, 57, 7483–7487 CrossRef CAS PubMed.
- Q. Miao and K. Pu, Adv. Mater., 2018, 30, 1801778 CrossRef PubMed.
- J. Li, X. Zhen, Y. Lyu, Y. Jiang, J. Huang and K. Pu, ACS Nano, 2018, 12, 8520–8530 CrossRef CAS PubMed.
- Y. Lyu, J. Zeng, Y. Jiang, X. Zhen, T. Wang, S. Qiu, X. Lou, M. Gao and K. Pu, ACS Nano, 2018, 12, 1801–1810 CrossRef CAS PubMed.
- X. Zhen, C. Zhang, C. Xie, Q. Miao, K. L. Lim and K. Pu, ACS Nano, 2016, 10, 6400–6409 CrossRef CAS PubMed.
- J. Li, C. Xie, J. Huang, Y. Jiang, Q. Miao and K. Pu, Angew. Chem., Int. Ed., 2018, 57, 3995–3998 CrossRef CAS PubMed.
- Y. Jiang and K. Pu, Adv. Biosyst., 2018, 2, 1700262 CrossRef.
- H. Zhu, Y. Fang, Q. Miao, X. Qi, D. Ding, P. Chen and K. Pu, ACS Nano, 2017, 11, 8998–9009 CrossRef CAS PubMed.
- Q. Miao, C. Xie, X. Zhen, Y. Lyu, H. Duan, X. Liu, J. V. Jokerst and K. Pu, Nat. Biotechnol., 2017, 35, 1102–1110 CAS.
- L. Li, Y. Liu, P. Hao, Z. Wang, L. Fu, Z. Ma and J. Zhou, Biomaterials, 2015, 41, 132–140 CrossRef CAS PubMed.
- Y. Cao, J.-H. Dou, N.-j. Zhao, S. Zhang, Y.-Q. Zheng, J.-P. Zhang, J.-Y. Wang, J. Pei and Y. Wang, Chem. Mater., 2017, 29, 718–725 CrossRef CAS.
- Y. Jiang, P. K. Upputuri, C. Xie, Y. Lyu, L. Zhang, Q. Xiong, M. Pramanik and K. Pu, Nano Lett., 2017, 17, 4964–4969 CrossRef CAS PubMed.
- Y. Jiang, J. Li, X. Zhen, C. Xie and K. Pu, Adv. Mater., 2018, 30, 1705980 CrossRef PubMed.
- Z. Cao, L. Feng, G. Zhang, J. Wang, S. Shen, D. Li and X. Yang, Biomaterials, 2018, 155, 103–111 CrossRef CAS PubMed.
- B. Guo, Z. Sheng, K. Kenry, D. Hu, X. Lin, S. Xu, C. Liu, H. Zheng and B. Liu, Mater. Horiz., 2017, 4, 1151–1156 RSC.
|
This journal is © The Royal Society of Chemistry 2019 |