DOI:
10.1039/C8BM01412D
(Paper)
Biomater. Sci., 2019,
7, 409-418
Paramagnetic CuS hollow nanoflowers for T2-FLAIR magnetic resonance imaging-guided thermochemotherapy of cancer†
Received
2nd November 2018
, Accepted 15th November 2018
First published on 16th November 2018
Abstract
The development of nanoplatforms with integrated therapeutic and imaging functions is necessary for highly efficient cancer therapy. Herein, 3D CuS hollow nanoflowers (HNs) consisting of 2D nanoplates are successfully fabricated through the technique of laser ablation in liquids followed by ion-exchange reactions and applied for the first time as a theranostic nanoagent for magnetic resonance imaging (MRI), photothermal therapy (PTT), and chemotherapy simultaneously. Due to the sufficient and immediate contact between the exposed cupric centers of nanoplates and protons from water molecules, CuS HNs are demonstrated to be capable of being a T1 positive contrast agent for efficient MRI of tumors on the T2-weighted fluid-attenuated inversion recovery imaging (T2-FLAIR) sequence. Besides, the hollow structure makes CuS HNs an efficient nanoplatform for drug loading with a laser-triggered drug release. Moreover, CuS HNs exhibit high photothermal conversion efficiency (30%) and good biocompatibility. The combination of PTT and chemotherapy with CuS HNs provides a significant synergistic therapeutic effect, resulting in a higher tumor inhibition ratio than PTT or chemotherapy alone. This study demonstrates a single-component multifunctional theranostic nanoagent for T2-FLAIR MRI guided thermochemotherapy, which has great potential application in theranostics of cancer.
Introduction
The development of imaging-guided therapy of cancer has attracted increasing attention in the field of visualization therapy due to its huge clinical significance.1–6 As a promising therapeutic modality, photothermal therapy (PTT) has been famous for its minimal invasiveness, remote control capability, high selectivity, and universality,7,8 so it has always been desired to integrate it with effective imaging functions. Researchers have developed multifunctional nanoplatforms by combining different imaging materials with PTT agents, such as Fe3O4 for magnetic resonance imaging (MRI), Au for X-ray computed tomography (CT) imaging, Y2O3:Yb,Er for photoluminescence imaging, and Ag for surface enhanced Raman scattering (SERS) imaging.9–12 Beyond that, some binary or ternary semiconductor nanomaterials also show intrinsic imaging and photothermally therapeutic capabilities simultaneously.13,14 They are regarded as multifunctional single nanoagents (MSNs), which have gained increasing interest due to their exquisite and time-saving synthesis and the avoidance of potential in vivo side effects caused by complicated compositions.1,2,15–18
MRI with contrast agents can rapidly provide 3D topographical data and simultaneous feedback on diseased tissues in vivo.19 Due to the abundant unpaired electrons of metal ions, Gd(III)- or Mn(II)-based T1 MRI contrast agents and magnetic iron oxide-based T2 MRI contrast agents are paramagnetic or superparamagnetic so as to shorten the longitudinal or transverse relaxation time of protons.20–23 Similar to them, Cu(II) also possesses an unpaired 3d electron. Furthermore, some Cu(II)-based compounds have already been proven to have the potential for use in MRI. For example, Cu(II)-doped polyaniline nanoshuttles can shorten the T1, leading to a bright contrast in T1-weighted magnetic resonance images.24,25 Perlman et al.26 reported that the longitudinal relaxivity r1 (0.38 mM−1 s−1) of CuO NPs is comparable to that of MnO NPs (0.37 mM−1 s−1). What's more, Okamoto reported that CuS is paramagnetic due to its positive magnetic susceptibility,27 which has already been proven to be a promising PTT candidate with strong absorption in the near-infrared (NIR) region.28–30 These results indicate that CuS exhibits the potential to be a MSN for MRI and PTT simultaneously. However, the exploration of CuS as an MRI contrast agent has not been reported yet due to the unsatisfied contrast ability of copper ion which has only one unpaired electron.
According to classic Solomon–Bloembergen–Morgan (SBM) theory,31 the enhancement of longitudinal relaxivity r1 requires more immediate contact between paramagnetic metal ions and water molecules. It has been proved that a 2D nanostructure with a large surface to volume ratio can expose more metal centers to enhance the immediate contact between metal ions and water molecules to effectively expedite spin-lattice relaxation.32 Fortunately, CuS easily forms 2D lamellar-like nanoarchitectures which can further assemble as 3D hierarchical nanostructures, such as flower-like hollow nanospheres.33 For hollow nanostructures, the mesoporous pores are considered as a perfect candidate drug carrier with a high loading capacity.16,34–36 It means that CuS with 3D hierarchical hollow nanostructures also has the potential for the synergistic therapeutic effect of PTT combined with chemotherapy. In the present work, we firstly confirmed the realistic feasibility of MRI-guided synergistic therapy that combines PTT with chemotherapy by single-component CuS hollow nanoflower (HN) loading with doxorubicin hydrochloride (DOX) (Scheme 1). Such 3D hollow nanoflowers consisting of 2D CuS nanoplates were prepared through a green technique of laser ablation in liquids followed by ion-exchange reactions. CuS HNs exhibited strong NIR absorption with 30% photothermal conversion efficiency and perceptible drug release after laser-triggering. Notably, CuS HNs significantly lightened the in vitro target area in MR images on the T1-weighted imaging (T1WI) and T2-weighted fluid-attenuated inversion recovery imaging (T2-FLAIR) sequences and the in vivo tumor tissues in MR images on the T2-FLAIR sequence. Furthermore, the obvious synergistic therapeutic efficiency of PTT and chemotherapy of the single-component theranostic nanoagents was confirmed by in vitro and in vivo experiments.
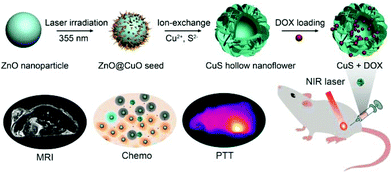 |
| Scheme 1 Schematic illustration of the synthetic processes of CuS HNs and MRI-guided synergistic therapy by combining PTT and chemotherapy. | |
Materials and methods
Materials
Zn (99.99%) metal plates were purchased from Beijing Goodwill Metal Technology Co., Ltd (Beijing, China). Copper nitrate trihydrate (Cu(NO3)2·3H2O, 99%) and ammonium sulphide solution ((NH4)2S, 20% in H2O) were obtained from Aladdin Industrial Corporation (Shanghai, China). Polyethylene glycol 400 (PEG-400) was supplied by Shanghai Lingfeng Chemical Reagent Co., Ltd. Ultrapure water was prepared by using a Milli-Q-Plus system.
Synthesis of ZnO nanoparticles
ZnO nanoparticles (NPs) were prepared according to our previous work.37 Briefly, Zn colloidal solution was obtained by ablating a piece of Zn plate for 15 min in ultrapure water (15 mL) by using a focused Nd/YAG pulsed laser with 1064 nm wavelength, 10 Hz frequency, 7 ns pulse duration and 100 mJ per pulse energy. The colloidal solution was then irradiated for 40 min under continuous stirring to acquire ZnO NPs by using an unfocused laser with 355 nm wavelength, 10 Hz frequency, 7 ns pulse duration and 40 mJ per pulse energy.
Synthesis of CuO hollow nanoflowers
To form a layer of CuO seed on the surface of ZnO NPs, firstly, a colloidal solution of ZnO NPs (40 mL) was mixed with copper nitrate trihydrate aqueous solution (1.6 mL, 0.1 M) under stirring for 10 min. Secondly, the mixture was irradiated using an unfocused laser with 355 nm wavelength, 10 Hz frequency, 7 ns pulse duration and 10 mJ per pulse energy for 1 h. Finally, the solution was then placed in a dry oven at 60 °C for 2 h to complete the reaction. The formed CuO hollow nanoflowers (HNs) were purified by rinsing with pure water three times and redispersed in 40 mL of water.
Synthesis of CuS HNs
Ammonium sulphide solution (0.16 mL) was rapidly added to the well-dispersed CuO HN suspension under vigorous stirring. The reaction system was continually stirred for 2 h at room temperature. The 3D CuS HNs were obtained and purified with water three times through centrifugation. A total of 50 mL of the CuS HN suspension was mixed with 2.5 mL of PEG-400 under stirring overnight to improve biocompatibility. The product was separated by centrifugation, washed with deionized water three times, and dried using a vacuum freeze dryer for further use.
Characterization of CuS HNs
The morphology and structure of the products were investigated using field-emission scanning electron microscopy (SEM, SU8020) and transmission electron microscopy (TEM, JEOL JEM-2010, Japan). The X-ray diffraction (XRD) pattern of the products was recorded using a Rigaku X-ray diffractometer (G2234) with Cu-Kα radiation (λ = 0.15419 nm). Optical absorption spectra were collected using a UV-vis-NIR spectrophotometer (Cary-5E). Fourier transform infrared (FTIR) spectra were recorded with a FTIR apparatus (Nicolet 8700). Concentrations of the samples were determined by inductively coupled plasma-atomic emission spectroscopy (ICP-AES, Optima 7300 DV). The pH of the PBS solution was varied by dropwise addition of HCl aqueous solution and monitored with an S220 Seven Compact pH meter (Mettler Toledo).
Photothermal performance of CuS HNs in ultrapure water
A total of 0.5 mL of CuS HNs dispersed in ultrapure water with various concentrations (0–100 μg mL−1) was added to a quartz cuvette (1 cm × 1 cm × 4.5 cm). The dispersion was irradiated for 10 min with a NIR (1064 nm) laser (Changchun New Industries Optoelectronics Tech. Co., Ltd, China) with a spot area of 0.2 cm2 at an appropriate power density. The temperature was recorded every 10 s using a thermocouple microprobe. Online infrared thermal images were acquired using a thermal infrared camera (FLIR, E60).
DOX loading and release experiments
For DOX loading, 5 mg of CuS HNs were dispersed in DOX aqueous solution (10 mL, 50 μg mL−1) and stirred at room temperature overnight. The excess DOX amount was removed by centrifugation and washed with ultrapure water. DOX-loaded CuS HNs (CuS + DOX) were dispersed in PBS with different pH values (4.5, 5.5, 6.5 and 7.4) to investigate the release capacity of DOX. The DOX amount was measured by UV-vis spectroscopy.
Cell culture
The human hepatocellular carcinoma cell line (HepG2), human umbilical vein endothelial cell line (HUVEC), and human normal hepatic cell line (LO2) were obtained from the American Type Culture Collection (Manassas, VA, USA). Cells were cultured in Dulbecco's modified Eagle's medium (GE Healthcare, Chicago, IL, USA) supplemented with 10% fetal bovine serum in a standard humidified incubator at 37 °C under a 5% CO2 atmosphere.
Cytotoxicity assay
Cell viability in the presence of CuS + DOX was investigated using a standard MTT assay conducted in triplicate as follows: HUVEC cells, LO2 cells, and HepG2 cells were seeded into 96-well plates containing 200 μL of culture medium at a density of 1 × 104 cells per well and grown overnight. The cells were then incubated with a series of CuS + DOX concentrations for 48 h and in a medium containing 20 μL of 5 mg mL−1 MTT for 4 h. The medium with MTT was removed, and 100 μL of DMSO was added to dissolve formazan crystals at room temperature for 30 min. Absorbance was recorded at 490 nm by using a multidetection microplate reader (ELx808, BioTek Instruments, Inc., Winooski, VT, USA).
Hemolysis assay
For in vitro hemolysis assay, the blood sample (5.0 mL) was stabilized with ethylenediamine tetraacetic acid and mixed with PBS (10 mL). Red blood cells (RBCs) were separated from the serum by centrifugation, washed, and diluted with PBS (50 mL). The diluted RBC suspension (400 μL) was mixed with CuS + DOX of different concentrations (1.6 mL, 0–1 mg mL−1). RBCs incubated with deionized water and PBS were employed as positive and negative control groups, respectively. After that, the samples were kept at room temperature for 4 h, and finally centrifuged (3000 rpm, 10 min). The absorbance values of the supernatants at 570 nm were measured using a multidetection microplate reader (ELx808, BioTek Instruments, Inc., Winooski, VT, USA). The RBC hemolysis percent was calculated using the following formula: percent hemolysis (%) = (Asample − Anegative)/(Apositive − Anegative) × 100%, where Asample, Anegative, and Apositive are the absorbance of the samples, and negative and positive control groups, respectively.
In vitro and in vivo MRI
In vitro and in vivo MRI experiments were performed on a Philips Achieva 3.0 T TX MRI System with imaging sequences widely used in clinical hepatic imaging. Magnevist was purchased from Bayer Greater China. Fe3O4 nanoparticles were prepared according to the literature.38 The specific imaging sequences were T1WI, T2-weighted imaging (T2WI), T2-FLAIR, T2-weighted spectral presaturation inversion recovery imaging (T2-SPIR), and T1 and T2 mapping. Briefly, 1.5 mL of the CuS + DOX dispersion at different concentrations was added to a 1.5 mL EP tube and placed in the testing system for signal detection in vitro. For Magnevist and Fe3O4, the same procedures of in vitro experiments were carried out. In vivo MRI of CuS + DOX was carried out on HepG2 tumor-bearing mice. The MRI data of the tumor site were collected before and after the intratumoral injection of the CuS + DOX solution (100 μL, 0.5 mg mL−1). The MRI signal intensities were average values, which were just collected from the MRI images using a Philips DICOM Viewer.
In vitro phototherapeutic effect
MTT and apoptosis assays were conducted to evaluate phototherapeutic effects at the cellular level. For MTT assay, HepG2 cells were seeded into 96-well plates containing 200 μL of culture medium at a density of 1 × 104 cells per well and grown overnight. A total of 200 μL of the medium containing the intervention drug was added to a cuvette to replace the culture medium. Samples were categorized as follows: (1) the control group (PBS only), (2) the PBS/laser group, (3) the DOX/laser group, (4) the CuS/laser group, and (5) the CuS + DOX/laser group (1 mg mL−1). Subsequently, the cells were incubated in a medium containing 20 μL of 5 mg mL−1 MTT for 4 h. The medium with MTT was then removed, and 100 μL of DMSO was added to dissolve formazan crystals at room temperature for 30 min. Absorbance was recorded at 490 nm by using a multidetection microplate reader (ELx808, BioTek Instruments, Inc., Winooski, VT, USA).
For apoptosis assay, HepG2 cells were plated in culture flasks. After 24 h, the cells were exposed to interventions, categorized into five groups the same as before, and maintained for 48 h. In brief, 1 × 105 cells were stained with fluorescein isothiocyanate (FITC)-conjugated annexin V and propidium iodide by using the Annexin V-FITC apoptosis detection kit (4A Biotech, Beijing, China) following the manufacturer's instructions to determine the extent of spontaneous apoptosis. The spontaneous apoptosis of cells was determined using an FACS Calibur II sorter and Cell Quest FACS system (BD Biosciences, Franklin Lakes, NJ, USA). The experiments were repeated three times.
In vivo antitumor effect
Female BALB/C nude mice (5 weeks, 18–22 g) were obtained from Beijing Vital River Experimental Animal Technology Co., Ltd. All in vivo experiments were implemented according to the criteria of the National Regulation of China for the Care and Use of Laboratory Animals. The tumors were inoculated by subcutaneous injection of 1 × 107 HepG2 cells in the left flank of each BALB/C nude mouse. After 2 weeks of growth, the tumor size reached approximately 200 mm3. The tumor-bearing nude mice were randomized into five groups (n = 3 each group), and 100 μL of PBS or CuS + DOX (1 mg mL−1) solution was intratumorally injected into each mouse. The five groups were as follows: (1) the control group (PBS only), (2) the PBS/laser group, (3) the DOX/laser group, (4) the CuS/laser group, and (5) the CuS + DOX/laser group. The power density of the NIR laser was 3 W cm−2, and the treatment began 2 h after the intratumoral injection. The phototherapeutic efficiency of each group was evaluated by measuring the tumor volume (V = length × width2/2).
Ultrasound
B-mode ultrasonography, Color Doppler Flow Imaging (CDFI), Color Power Angio (CPA), and Ultrasonic Elastosonography (USE) of Philips iU Elite Ultrasound System (Philips Healthcare, Amsterdam, the Netherlands) were used to evaluate tumor growth, angiogenesis, micro-angiogenesis, and tumor hardness degree after treatment in vivo, respectively. Analyses were conducted by an experienced ultrasound doctor, who was blinded to the study.
Results and discussion
Synthesis and characterization of CuS HNs
In our work, CuS HNs were synthesized using ZnO nanospheres as templates, reported in our previous work.37 First, a mixture solution containing Cu(NO3)2 and ZnO nanospheres was irradiated using a pulsed laser to obtain ZnO@CuO seeds. Subsequently, cation and anion ion-exchange reactions were carried out successively to obtain CuS HNs. The formation mechanism of CuS HNs is discussed in detail in the ESI (Fig. S1†). The SEM images (Fig. 1a) showed that CuS displayed a 3D hollow flower-like structure consisting of small 2D nanoplates. In Fig. 1b, the TEM image shows nanoplates with 30–120 nm diameters and approximately 5 nm thicknesses. These CuS nanoplates extended outwardly like petals so as to have sufficient immediate contact with water molecules. Such hierarchical CuS nanoflowers obviously possessed pores. The porosity of such a hollow structure was investigated via Brunauer–Emmett–Teller analysis of nitrogen adsorption–desorption isotherms (Fig. 1c). The CuS HNs sample has a surface area of 24.5 m2 g−1 and a total volume of 0.153 cm3 g−1, including mesopores and macropores. All the diffraction peaks of CuS HNs in the XRD pattern (Fig. 1d) can be well indexed to hexagonal CuS (JCPDS, 00-001-1281), thereby indicating the high purity of the nanocomposite.
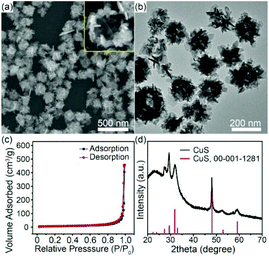 |
| Fig. 1 (a) SEM and (b) TEM images, (c) N2 adsorption-desorption isotherm, and (d) XRD patterns of CuS HNs. | |
Optical and photothermal properties of CuS HNs
PTT nanoagents should have a high NIR absorption capacity to exhibit high photothermal conversion efficiency. Fig. 2a shows the UV-vis-NIR spectra of aqueous dispersions of CuS HNs at various concentrations. CuS HNs showed a strong absorption in the NIR region, and the absorption capacity increased as the wavelength increased from 600 to 1200 nm. With the increasing concentration of CuS HNs from 6.25, 12.5, and 50 to 100 μg mL−1, the absorbance at 1064 nm increased linearly, indicating the good dispersion capability of CuS HNs in water. The mass extinction coefficient was calculated to be 21.2 L g−1 cm−1. To further investigate the photothermal conversion performance of CuS HNs, the laser source was selected as 1064 nm as a typifier in the second NIR region (1000–1350 nm), which could offer a longer efficient tissue penetration depth than the first NIR region (650–950 nm).9,39 As shown in Fig. 2b and c, the temperature of the CuS HN solutions increased upon 1064 nm laser irradiation with increasing material dosage and irradiation power. Under laser irradiation with a power density of 3 W cm−2, the increasing extent of temperature in CuS HN solution with various concentrations was from 8.4 °C to 22.5 °C, which was higher than that of ultrapure water (ΔT = +3 °C). Especially for 100 μg mL−1 CuS HN suspension (Fig. 2d), the temperature could rise from 17.3 °C to 40 °C, which was confirmed by IR thermal imaging. This was very close to the data measured using a thermocouple microprobe in Fig. 2b. In addition, the time constant (τs) of CuS HNs was obtained by fitting the cooling time versus the negative natural logarithm of the temperature driving force (θ), with a slope of 224.4 s (Fig. 2e and Fig. S2a and b†). Therefore, the photothermal conversion efficiency of CuS HNs was calculated to be 30%.
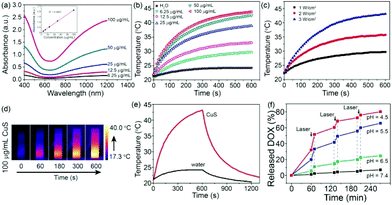 |
| Fig. 2 (a) UV-vis-NIR absorption spectra of CuS HN aqueous dispersions with different concentrations from 6.25 to 100 μg mL−1. (b) Temperature elevation of water and the CuS HN aqueous solutions under irradiation of a laser (1064 nm, 3 W cm−2). (c) Temperature elevation of 100 μg mL−1 CuS HN aqueous dispersion under the 1064 nm laser irradiation with different power densities. (d) IR thermal images of CuS HN aqueous dispersion in a quartz cuvette. (e) Heating and cooling curves of deionized water and the CuS HN aqueous suspension. (f) DOX release from the CuS + DOX triggered by 1064 nm laser irradiation at various pH and selected time points. | |
Drug loading and release
CuS HNs were expected to efficiently deliver drugs to tumor tissues for chemotherapy due to their large surface area and hollow structure. As a chemotherapeutic drug, DOX is widely used as a model to estimate the drug loading capacity of nanoagents.16,40,41 DOX was loaded through soaking CuS HNs in DOX aqueous solution overnight to investigate the drug loading capacity of CuS HNs. DOX showed a characteristic broad peak at 480 nm and three sharp peaks in the UV region (Fig. S3a†). Compared with pure CuS HNs, CuS + DOX exhibited an increased absorbance below 600 nm in the UV-vis-NIR spectra. Moreover, the DOX loading was confirmed from the FTIR spectra (Fig. S3b†). Therefore, DOX was successfully loaded in CuS HNs. Given the perfect linear relationship between the absorbance and concentration of DOX, the DOX loading capacity was estimated to be approximately 2.69% according to the UV-vis-NIR spectra in Fig. S3c and d.† Considering the variable pH value in vivo, the DOX release was investigated in PBS solutions at pH 4.5, 5.5, 6.5 and 7.4, respectively. As shown in Fig. 2f, CuS + DOX exhibited evident pH- and time-dependent drug release properties. With increasing time, DOX was gradually released in PBS solutions. In contrast to the extremely limited release of DOX at pH 7.4, the amount of DOX released reached 80.2%, 65.6% and 25.6% at pH 4.5, 5.5 and 6.5, respectively. This property might benefit drug release at tumor sites because of the acidic tumor microenvironment (pH approximately 6.5–6.8),42 where DOX-loaded CuS HNs can accumulate due to the enhanced permeability and retention effect. Furthermore, DOX-loaded CuS HNs displayed a laser stimuli-triggered drug release property. The amounts of DOX released were 32.1% (pH 4.5), 19.9% (pH 5.5) and 5.7% (pH 6.5) after 1 h at room temperature. After the irradiation of the product with a 1064 nm laser for 10 min, the amounts of DOX released increased by 19.5% (pH 4.5), 13.4% (pH 5.5) and 5.7% (pH 6.5). The speed of DOX release with laser irradiation was calculated to be four times as fast as that without laser irradiation (Fig. S3e†). The burst and rapid release could be attributed to the photothermal effect of CuS HNs because a high temperature can evoke the desorption of DOX from CuS HNs. Consequently, CuS HNs could rapidly release DOX from the hollow structure under the trigger of the 1064 nm laser.
In vitro and in vivo MR imaging
Owing to the excellent photothermal conversion efficiency and capability of drug delivery, low cytotoxicity, and facile synthesis, CuS HNs were expected to be used in MRI. According to the hysteresis loop of CuS HNs at 300 K (Fig. S4†), magnetization intensities (M) were linearly related to magnetic field intensities (H) ranging from −20 to 20 kOe. This suggests that CuS HNs were paramagnetic and the magnetic susceptibility of CuS HNs was calculated to be 1.81 × 10−6 emu g−1 Oe−1. These results indicated that CuS HNs might be capable for use in MRI. As for the in vitro and in vivo MR imaging experiments, longitudinal and transverse relaxation times (T1 and T2) were measured, and the corresponding mole relaxation rates and mass concentration-related relaxation rates both were calculated to evaluate the MRI capacity of CuS HNs. For comparison, Magnevist (T1 MRI contrast agent) and Fe3O4 (T2 MRI contrast agent) were also investigated under the same conditions. As shown in Fig. 3a and S5a,† the longitudinal mole relaxation rates (r1, the longitudinal relaxation rate per mM) ranged from large to small in the order of 2.86 (magnevist), 0.49 (CuS HNs) and 0.09 (Fe3O4) mM−1 s−1; the transverse mole relaxation rates (r2, the transverse relaxation rate per mM) of Fe3O4, magnevist and CuS HNs were 55.92, 13.21 and 1.98 mM−1 s−1, respectively. Such a low r2 value of CuS HNs indicated that they were unqualified to be a T2 MRI contrast agent. Although the r1 of CuS HNs was also smaller than that of magnevist, it was larger than that of CuO nanoparticles, reported as T1 MRI contrast agents.26 What's more, the total mass of agents ingested by patients is a key judgement criteria for clinicians. We further calculated the mass concentration-related r1 (mr1) and mass concentration-related r2 (mr2) shown in Fig. S5b.† The mr2 of Fe3O4 was much larger than those of both CuS HNs and magnevist. Besides, mr1 of CuS HNs was calculated to be 5.1 (mg mL−1)−1 s−1, which was 1.6 times as much as 3.1 (mg mL−1)−1 s−1 of magnevist due to the larger molecular weight of magnevist than CuS HNs (Fig. 3b). These results confirmed that CuS HNs could be utilized as a T1 MRI contrast agent. Therefore, mass concentration related in vitro MRI of CuS HNs was then conducted on four MRI sequences (T1WI, T2WI, T2-FLAIR, and T2-SPIR). Both CuS (Fig. 3c) and magnevist (Fig. S6a†) could significantly brighten the target areas on the T1WI or T2-FLAIR sequence as their mass concentrations increased. Above all, the relative MRI signal intensity of CuS HNs (Fig. S7†) was larger than that of magnevist (Fig. S6b†) at the same mass concentration. The results further suggested that CuS HNs could be used as T1 MRI contrast agents and exhibited better MRI performances than magnevist.
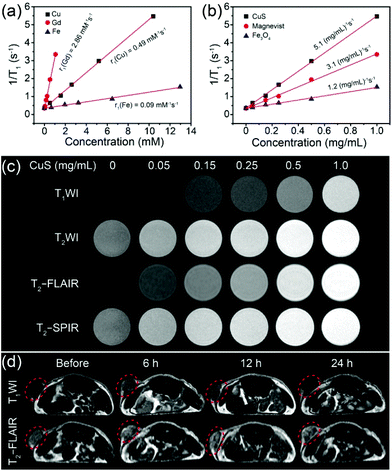 |
| Fig. 3 Linear fittings of 1/T1 of CuS HNs, magnevist, and Fe3O4 at different (a) Cu, Gd, and Fe mole concentrations and (b) mass concentrations, (c) in vitro MR images of CuS + DOX aqueous dispersions at different mass concentrations (TE/TR for T1WI, T2WI, T2-FLAIR and T2-SPIR are 28 ms/651 ms, 104 ms/3 s, 125 ms/8 s and 104 ms/3 s, respectively. The flip angle is 90°), and (d) MR images of tumors on mice before and 6, 12, and 24 h after intratumoral injection with CuS + DOX (1.0 mg mL−1, 100 μL) in T1WI and T2-FLAIR MRI. Tumor sites are marked with red dashed circles. | |
Noteworthily, according to Fig. S6b and S7,† it could be found that the MRI signal intensities of both CuS HNs and magnevist on the T2-FLAIR sequence were larger than that on the T1WI sequence at the same mass concentration. These phenomena could be explained through the MRI principle on T2-FLAIR,43 which consisted of the 180°, 90°, and 180° radiofrequency (RF) pulse, as shown in Fig. 4a. TI was inversion time, TE was echo time, and TR was recovery time. At first, the 180° RF pulse was employed to invert the net magnetic moment of protons. After a delay time TI, during which a small part of longitudinal magnetic moments (Mz) of protons recovered, a 90° RF pulse was applied to turn the recovered Mz into transverse magnetic moments (Mxy). Then spin echo signals of Mxy are detected to acquire T2WI images since Mxy are focused by a 180° RF pulse at the xy plane. In our in vitro case, compared with the control group (water only), CuS nanoplates could benefit from the sufficient and immediate contact between the exposed Cu(II) ions and water molecules so as to shorten the T1 relaxation time of protons from water. As a result, more protons recovered to Mz during the decay time TI, which then contributed to T2WI images (Fig. 4b), and the relative MRI signal intensity on the T2-FLAIR sequence increased with the ascending mass concentration of CuS HNs (Fig. S7†). The MRI signal intensities of water on the T2-FLAIR sequence were similar to that on the T1WI sequence. The increases in MRI signal intensity both originated from the protons of partial water molecules affected by the T1 MRI contrast agent, which were constant in a certain sample. Thereby, the problem was transformed into another problem of the comparison of the T1WI and T2WI signal intensities of the protons of partial water molecules. Nevertheless, MRI signal intensities of protons from water on the T2WI sequence are much higher than than those on the T1WI sequence and this phenomenon is difficult to reverse by the T1 MRI contrast agent with low concentration. Therefore, the T2-FLAIR sequence could not only be applied to the MRI images of T1 MRI contrast agents, such as CuS HNs, but also was superior to the T1WI sequence for detecting T1 MRI contrast agents with low concentrations.
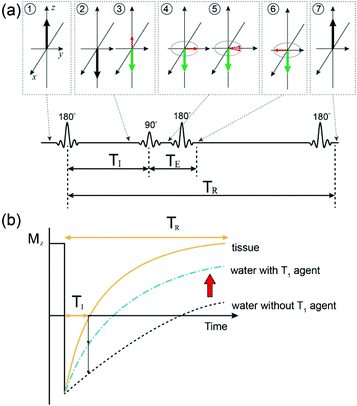 |
| Fig. 4 Scheme of (a) T2-FLAIR sequence and the magnetic moments of protons at different stages and (b) the recovery of longitudinal magnetic moments (Mz) after a 180° radiofrequency pulse with/without the T1 MRI contrast agent. | |
Based on the in vitro MRI results, we employed CuS + DOX for in vivo tumor MRI (Fig. 3d). The tumor-bearing mice were imaged before and after (6, 12, and 24 h) intratumoral injections with CuS + DOX (0.5 mg mL−1, 100 μL). The results showed that the tumor region was lightened at 6 h after the injection of CuS + DOX, and then gradually darkened on both T2-FLAIR and T1WI sequences. However, the T2-FLAIR sequence was more remarkable than the T1WI sequence. These results were consistent with the in vitro results. Thus, as a positive contrast agent in T2-FLAIR MRI, CuS HNs have great potential for efficient diagnosis of cancers.
In vitro cytotoxicity and phototherapeutic treatment
Biocompatibility is a prerequisite for biomedical applications of nanoagents. Cytocompatibility of the CuS + DOX was evaluated by MTT viability assay of HepG2 cells, HUVEC cells, and LO2 cells. As shown in Fig. 5c, after incubation with CuS + DOX for 48 h, no significant cytotoxicity was observed in all cells at CuS concentrations as high as 1 mg mL−1. A hemolytic assay was also conducted to assess the hemocompatibility of CuS + DOX by using deionized water (positive) and PBS (negative) as control groups to further examine the biocompatibility of the nanoagent. As demonstrated in Fig. 5b and Fig. S8,† negligible hemolysis was observed at all indicated CuS concentrations (from 0.15 to 1 mg mL−1), indicating its excellent hemocompatibility. All these results suggested that CuS + DOX exhibits good biocompatibility, which is essential for further biomedical applications.
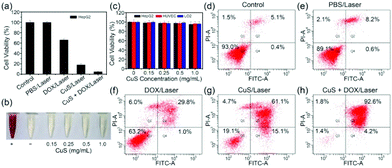 |
| Fig. 5 (a) Cell viability of HepG2 cells after treatment using PBS, DOX, CuS, or CuS + DOX upon NIR laser irradiation. (b) In vitro hemolysis assay of red blood cells incubated with CuS + DOX at various concentrations of CuS for 4 h. (c) Cell viability after incubation with CuS + DOX at different concentrations of CuS for 48 h. Apoptosis of cells in the control group (PBS only) (d) without or (e) with laser irradiation and (f–h) incubated with CuS, DOX, and CuS + DOX after laser irradiation. Error bars correspond to mean ± standard deviations. | |
The in vitro therapeutic effect of the combined PTT and chemotherapy of CuS + DOX on HepG2 cells was further investigated by MTT viability assay and apoptosis assay. As shown in Fig. 5a, when HepG2 cells treated with CuS + DOX were irradiated with a 1064 nm NIR laser (3 W cm−2), the cell viability was only 5%, which is significantly lower than that of HepG2 cells treated with CuS (18%) or DOX (66%) alone followed by the same laser irradiation. After only PBS treatment under laser irradiation for 10 min, HepG2 cells did not display any appreciable viability changes compared with the control group. These results suggested that the highest therapeutic efficacy can be acquired with the combined therapy of CuS + DOX compared with PTT of CuS or chemotherapy of DOX alone (Fig. S9†). Additionally, compared with the control group (Fig. 5d), NIR irradiation almost did not induce apoptosis (8.8%) for cells exposed to PBS solution (Fig. 5e), but induced a slight increase in apoptosis (30.8%) for the cells exposed to DOX solution (Fig. 5f). However, significant apoptosis (76.2%) was observed with laser irradiation for cells exposed to CuS solution (Fig. 5g). When the cells were exposed to CuS + DOX solution, NIR irradiation induced the largest percentage of apoptosis, which reached 96.8% (Fig. 5h). These results indicated that the drug loading nanoplatform of CuS + DOX showed a good synergistic effect between the PTT of CuS and the chemotherapy of DOX with the irradiation of the NIR laser.
In vivo combined therapy
In vivo experiments were performed due to the good photothermal properties of CuS HNs and the strong in vitro inhibition efficacy of the combination of PTT and chemotherapy of CuS + DOX toward the tumor. Tumor-bearing mice were randomly divided into five groups (three in each group): (1) control group (PBS only), (2) PBS/laser, (3) DOX/laser, (4) CuS/laser, and (5) CuS + DOX/laser. During irradiation, an IR thermal camera was used to monitor the temperature variation in tumors at selected time points (Fig. 6a). After 10 min of irradiation, only a slight tumor temperature increase (approximately 5 °C) was observed for the PBS/laser and DOX/laser groups. By contrast, the tumor temperature of the CuS/laser and CuS + DOX/laser groups rapidly increased and reached a plateau of approximately 61 °C after 2 min irradiation (Fig. 6b); this temperature was considered to be sufficient to induce hyperthermia and immediately eradicate the tumor. The large temperature increase confirmed the powerful in vivo PTT effect of the CuS. After 14 days, the weight and volume of the tumors were measured to evaluate the combined therapeutic efficiency. Fig. 6c and d show the tumor photographs and the mean tumor weights of each group. The weight of the tumors in the PBS/laser group was slightly lighter than that in the control group, suggesting that the laser alone has a slight impact on tumor growth. However, the weight and size of the tumors exposed to DOX or CuS under laser irradiation showed an evident decrease, which could be attributed to chemotherapy or PTT alone, respectively. Compared with DOX treatment, the lighter weight of the tumors treated with CuS indicated a stronger inhibition by PTT than chemotherapy for tumor growth. As was predicted, CuS + DOX showed the strongest inhibition of tumors. Among all the groups, the lowest mean tumor weight was 3 mg and found in the CuS + DOX/laser group. The tumors nearly completely disappeared in the mice after laser irradiation. Moreover, no apparent variation of the mice body weight was observed for all treatments (Fig. 6e), indicating the low/no systemic toxicity of the combined therapy in vivo. These in vivo results directly demonstrated the good biocompatibility of CuS + DOX and the considerably higher inhibition efficacy and synergistic therapeutic effect of thermochemotherapy than either PTT or chemotherapy alone.
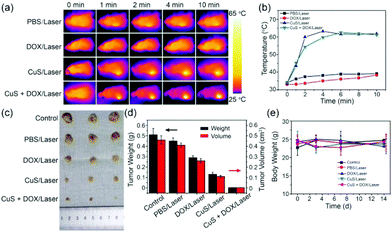 |
| Fig. 6 (a) In vivo IR thermal images of tumor-bearing mice intratumorally injected with PBS, DOX, CuS, or CuS + DOX upon NIR laser radiation. (b) Temperature variation of tumors as a function of irradiation time. (c) Tumor photos collected from the mice. (d) Average weight and volume of tumors. (e) Body weights of mice for different groups. Error bars correspond to mean ± standard deviations. | |
Ultrasound observation of solid tumor evolution
More significantly, clinical ultrasound imaging was employed to monitor and reveal the evolution of tumors after treatment with the merit of noninvasiveness, which can effectively prevent complications or death. B-mode ultrasonography was employed to monitor the evolution of tumors at designed time points after treatment. As shown in the B-mode sonogram (Fig. S10a, c and d†), the tumors of the control or PBS/laser group rapidly grew over the following days and doubled in length and width at 14 days. Under laser irradiation, the tumor size decreased during the first seven days and then regrew with the DOX treatment, but gradually decreased after CuS treatment over 14 days. By contrast, the tumor size of the CuS + DOX group rapidly decreased from the first to the seventh day and eventually completely vanished after 14 days of treatment, which is consistent with previous in vivo antitumor observations. Moreover, Color Doppler Flow Imaging (CDFI) and Color Power Angio (CPA) showed that the angiogenesis and micro-angiogenesis rates (Fig. S10b† and Fig. 7a) were in accordance with the varieties of B-mode ultrasonography, respectively. In addition, ultrasonic elastosonography (USE) revealed that the tumors of the CuS/laser and CuS + DOX/laser groups softened on the third day after treatment (Fig. 7b), which might be related to the necrosis after treatment.44 These results further confirmed the synergistic therapeutic effect of the combination of PTT and chemotherapy and the outstanding tumor ablation capability of the CuS + DOX vehicles.
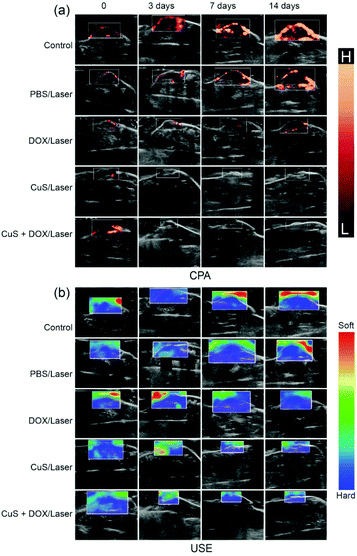 |
| Fig. 7 (a) Color Power Angio (CPA) and (b) Ultrasonic Elastosonography (USE) for monitoring the evolution of tumors after treatment. | |
Conclusion
By employing laser ablation in liquids and ion-exchange reactions, we developed a template method to construct uniform and porous CuS HNs for multifunctional application in MRI-guided PTT and drug delivery. This is the first time CuS has been successfully used as a MRI contrast agent in in vitro and in vivo experiments. The longitudinal mass relaxation rate of CuS HNs is 1.6 times as much as that of magnevist. With the intratumoral injection of CuS HNs in vivo, the tumor regions were evidently lightened in the T2-FLAIR MRI images. Comprehensive investigations demonstrated that CuS HNs exhibit preeminent cytocompatibility and hemocompatibility, high drug loading capability, easy drug release under acidic conditions triggered by laser irradiation, and a good synergistic therapeutic effect when combined with PTT and chemotherapy. Hence, CuS HNs showed great potential for T2-FLAIR MRI-guided thermochemotherapy of cancer.
Notes
All the in vivo experiments were implemented according to the criteria of the National Regulation of China for Care and Use of Laboratory Animals. All animal protocols were investigated and approved by the Committee on the Use of Live Animals in Teaching and Research of the Harbin Medical University, Harbin, China. All animal experiments were strictly performed in compliance with the Regulations on the Administration of Laboratory Animals approved by the State Council of the People's Republic of China (http://www.calas.org.cn/html/jypx/zcfg/20111129/1309.html) and the Guidelines for the Care and Use of Experimental Animals approved by the Heilongjiang Provincial People's Congress (http://www.hljkjt.gov.cn/html/ZWGK/ZCFG/heilongjiang/show-18086.html).
Conflicts of interest
There are no conflicts to declare.
Acknowledgements
This work was financially supported by the National Basic Research Program of China (no. 2014CB931704), the Instrument Developing Project of the Chinese Academy of Sciences (grant no. YZ201627) and the National Natural Science Foundation of China (NSFC, no. 51571186, 11504375, 11604320, 11674321).
References
- Y. X. Zhang, B. Li, Y. J. Cao, J. B. Qin, Z. Y. Peng, Z. Y. Xiao, X. J. Huang, R. J. Zou and J. Q. Hu, Dalton Trans., 2015, 44, 2771 RSC.
- B. Li, K. C. Ye, Y. X. Zhang, J. B. Qin, R. J. Zou, K. B. Xu, X. J. Huang, Z. Y. Xiao, W. J. Zhang, X. W. Lu and J. Q. Hu, Adv. Mater., 2015, 27, 1339 CrossRef CAS PubMed.
- Q. T. Jin, W. J. Zhu, D. W. Jiang, R. Zhang, C. J. Kutyreff, J. W. Engle, P. Huang, W. B. Cai, Z. Liu and L. Cheng, Nanoscale, 2017, 9, 12609 RSC.
- L. Cheng, D. W. Jiang, A. Kamkaew, H. F. Valdovinos, H. J. Im, L. Z. Feng, C. G. England, S. Goel, T. E. Barnhart, Z. Liu and W. B. Cai, Adv. Funct. Mater., 2017, 27, 1702928 CrossRef PubMed.
- Y. Yang, Y. Chao, J. J. Liu, Z. L. Dong, W. W. He, R. Zhang, K. Yang, M. W. Chen and Z. Liu, Npg Asia Mater., 2017, 9, e344 CrossRef CAS.
- Y. Y. Xu, J. Xiang, H. Zhao, H. S. Liang, J. Huang, Y. Li, J. Pan, H. T. Zhou, X. G. Zhang, J. H. Wang, Z. Liu and J. Wang, Biomaterials, 2016, 100, 91 CrossRef CAS PubMed.
- Z. H. Bao, X. R. Liu, Y. D. Liu, H. Z. Liu and K. Zhao, Asian J. Pharm. Sci., 2016, 11, 349 CrossRef.
- X. J. Song, Q. Chen and Z. Liu, Nano Res., 2015, 8, 340 CrossRef CAS.
- Z. C. Wu, W. P. Li, C. H. Luo, C. H. Su and C. S. Yeh, Adv. Funct. Mater., 2015, 25, 6527 CrossRef CAS.
- L. J. Jing, X. L. Liang, Z. J. Deng, S. S. Feng, X. D. Li, M. M. Huang, C. H. Li and Z. F. Dai, Biomaterials, 2014, 35, 5814 CrossRef CAS PubMed.
- R. C. Lv, P. P. Yang, F. He, S. L. Gai, G. X. Yang and J. Lin, Chem. Mater., 2015, 27, 483 CrossRef CAS.
- L. Y. Zeng, Y. W. Pan, S. J. Wang, X. Wang, X. M. Zhao, W. Z. Ren, G. M. Lu and A. G. Wu, ACS Appl. Mater. Interfaces, 2015, 7, 16781 CrossRef CAS PubMed.
- T. Bao, W. Y. Yin, X. P. Zheng, X. Zhang, J. Yu, X. H. Dong, Y. Yong, F. P. Gao, L. Yan, Z. J. Gu and Y. L. Zhao, Biomaterials, 2016, 76, 11 CrossRef CAS PubMed.
- W. Y. He, K. L. Ai, C. H. Jiang, Y. Y. Li, X. F. Song and L. H. Lu, Biomaterials, 2017, 132, 37 CrossRef CAS PubMed.
- Z. Y. Xiao, C. T. Xu, X. H. Jiang, W. L. Zhang, Y. X. Peng, R. J. Zou, X. J. Huang, Q. Liu, Z. Y. Qin and J. Q. Hu, Nano Res., 2016, 9, 1934 CrossRef CAS.
- Z. L. Li, J. Liu, Y. Hu, K. A. Howard, Z. Li, X. L. Fan, M. L. Chang, Y. Sun, F. Besenbacher, C. Y. Chen and M. Yu, ACS Nano, 2016, 10, 9646 CrossRef CAS PubMed.
- J. R. McCarthy, Nanomedicine, 2009, 4, 693 CrossRef PubMed.
- C. Y. Yang, Y. D. Chen, W. Guo, Y. Gao, C. Q. Song, Q. Zhang, N. N. Zheng, X. J. Han and C. S. Guo, Adv. Funct. Mater., 2018, 1706827 CrossRef.
- H. Sakuma, J. Magn. Reson. Imaging, 2007, 26, 3 CrossRef PubMed.
- D. Pan, A. H. Schmieder, S. A. Wickline and G. M. Lanza, Tetrahedron, 2011, 67, 8431 CrossRef CAS PubMed.
- K. S. Kim, W. Park, J. Hu, Y. H. Bae and K. Na, Biomaterials, 2014, 35, 337 CrossRef CAS PubMed.
- Z. H. Miao, H. Wang, H. J. Yang, Z. L. Li, L. Zhen and C. Y. Xu, ACS Appl. Mater. Interfaces, 2015, 7, 16946 CrossRef CAS PubMed.
- J. C. Li, Y. Hu, J. Yang, P. Wei, W. J. Sun, M. W. Shen, G. X. Zhang and X. Y. Shi, Biomaterials, 2015, 38, 10 CrossRef CAS PubMed.
- M. Lin, D. D. Wang, S. Y. Li, Q. Tang, S. W. Liu, R. Ge, Y. Liu, D. Q. Zhang, H. C. Sun, H. Zhang and B. Yang, Biomaterials, 2016, 104, 213 CrossRef CAS PubMed.
- R. Ge, M. Lin, X. Li, S. W. Liu, W. J. Wang, S. Y. Li, X. Zhang, Y. Liu, L. D. Liu, F. Shi, H. C. Sun, H. Zhang and B. Yang, ACS Appl. Mater. Interfaces, 2017, 9, 19706 CrossRef CAS PubMed.
- O. Perlman, I. S. Weitz and H. Azhari, Phys. Med. Biol., 2015, 60, 5767 CrossRef CAS PubMed.
- K. Okamoto, S. Kawai and R. Kiriyama, Jpn. J. Appl. Phys., 1969, 8, 718 CrossRef CAS.
- Q. W. Tian, M. H. Tang, Y. G. Sun, R. J. Zou, Z. G. Chen, M. F. Zhu, S. P. Yang, J. L. Wang, J. H. Wang and J. Q. Hu, Adv. Mater., 2011, 23, 3542 CrossRef CAS PubMed.
- Q. W. Tian, F. R. Jiang, R. J. Zou, Q. Liu, Z. G. Chen, M. F. Zhu, S. P. Yang, J. L. Wang, J. H. Wang and J. Q. Hu, ACS Nano, 2011, 5, 9761 CrossRef CAS PubMed.
- B. Li, Q. Wang, R. J. Zou, X. J. Liu, K. B. Xu, W. Y. Li and J. Q. Hu, Nanoscale, 2014, 6, 3274 RSC.
- B. L. Randall, Chem. Rev., 1987, 87, 901 CrossRef.
- H. Hu, S. L. Liu, D. Li, M. Z. Wang, R. Moats, H. Shan, P. S. Conti and Z. B. Li, J. Mater. Chem. B, 2014, 2, 3998 RSC.
- Y. X. Liu, Z. X. Zhou, S. P. Zhang, W. H. Luo and G. F. Zhang, Appl. Surf. Sci., 2018, 442, 711 CrossRef CAS.
- K. Dong, Z. Liu, Z. H. Li, J. S. Ren and X. G. Qu, Adv. Mater., 2013, 25, 4452 CrossRef CAS PubMed.
- J. P. Yang, D. K. Shen, L. Zhou, W. Li, X. M. Li, C. Yao, R. Wang, A. M. El-Toni, F. Zhang and D. Y. Zhao, Chem. Mater., 2013, 25, 3030 CrossRef CAS.
- X. Y. Meng, G. H. Tian, Y. J. Chen, R. T. Zhai, J. Zhou, Y. H. Shi, X. R. Cao, W. Zhou and H. G. Fu, CrystEngComm, 2013, 15, 5144 RSC.
- H. Zhang, S. L. Wu, J. Liu, Y. Y. Cai and C. H. Liang, Phys. Chem. Chem. Phys., 2016, 18, 22503 RSC.
- X. M. Guo, B. Guo, Q. Zhang and X. Sun, Dalton Trans., 2011, 40, 3039 RSC.
- K. Welsher, S. P. Sherlock and H. J. Dai, Proc. Natl. Acad. Sci. U. S. A., 2011, 108, 8943 CrossRef CAS PubMed.
- T. Liu, C. Wang, X. Gu, H. Gong, L. Cheng, X. Z. Shi, L. Z. Feng, B. Q. Sun and Z. Liu, Adv. Mater., 2014, 26, 3433 CrossRef CAS PubMed.
- Z. Q. Meng, F. Wei, R. H. Wang, M. G. Xia, Z. G. Chen, H. P. Wang and M. F. Zhu, Adv. Mater., 2016, 28, 245 CrossRef CAS PubMed.
- B. A. Webb, M. Chimenti, M. P. Jacobson and D. L. Barber, Nat. Rev. Cancer, 2011, 11, 671 CrossRef CAS PubMed.
- I. L. Pykett, J. H. Newhouse, F. S. Buonanno, T. J. Brady, M. R. Goldman, J. P. Kistler and G. P. Pohost, Radiology, 1982, 143, 157 CrossRef CAS PubMed.
- W. Guo, F. Wang, D. D. Ding, C. Q. Song, C. S. Guo and S. Q. Liu, Chem. Mater., 2017, 29, 9262 CrossRef CAS.
Footnotes |
† Electronic supplementary information (ESI) available. See DOI: 10.1039/c8bm01412d |
‡ These authors contributed equally to this work. |
|
This journal is © The Royal Society of Chemistry 2019 |
Click here to see how this site uses Cookies. View our privacy policy here.