DOI:
10.1039/C8BM01267A
(Paper)
Biomater. Sci., 2019,
7, 389-408
Disturbance of adhesomes by gold nanoparticles reveals a size- and cell type-bias†
Received
9th October 2018
, Accepted 18th November 2018
First published on 22nd November 2018
Abstract
Gold nanoparticles (AuNP) have been thoroughly studied as multifunctional theranosis agents for cell imaging and cancer therapy as well as sensors due to their tunable physical and chemical properties. Although AuNP have proved to be safe in a wide concentration range, yet other important biological effects can arise in the sublethal window of treatment. This is especially pivotal to understand how AuNP can affect cell biology when labeling steps are needed for cell tracking in vivo, as nanoparticle loading can affect cell migratory/invasion ability, a function mediated by filamentous actin-rich nanometric structures collectively called adhesomes. It is noteworthy that, although numerous research studies have addressed the cell response to AuNP loading, yet none of them focuses on adhesome dynamics as a target of intracellular pathways affected by AuNP. We intend to study the collective dynamics of adhesive F-actin rich structures upon AuNP treatment as an approach to understand the complex AuNP-triggered modulation of migration/invasion related cellular functions. We demonstrated that citrate-coated spherical AuNP of different sizes (3, 11, 16, 30 and 40 nm) disturbed podosome-forming rosettes and the resulting extracellular matrix (ECM) degradation in a murine macrophage model depending on core size. This phenomenon was accompanied by a reduction in metalloproteinase MMP2 and an increment in metalloproteinase inhibitors, TIMP-1/2 and SerpinE1. We also found that AuNP treatment has opposite effects on focal adhesions (FA) in endothelial and mesenchymal stem cells. While endothelial cells reduced their mature FA number and ECM degradation rate upon AuNP treatment, mouse mesenchymal stem cells increased the number and size of mature FA and, therefore, the ECM degradation rate. Overall, AuNP appear to disturb adhesive structures and therefore migratory/invasive cell functions measured as ECM degradation ability, providing new insights into AuNP–cell interaction depending on cell type.
1. Introduction
The application of nanoparticles in cancer therapy has attracted huge attention in the last two decades;1–3 however, few insightful reports have addressed the whole complexity of nanoparticle–cell interaction. The knowledge gap in cell behavior upon nanoparticle encounter ultimately affects our understanding of crucial aspects of nanoparticle-based therapy, such as nanoparticle biodistribution or immunogenicity. The need for a better understanding of the biological impact that nanoparticle internalization has on cell behavior is even more acute for cell-based targeted therapies involving nanoparticle labeling. Iron oxide nanoparticle4–6 or gold nanoparticle7,8 loading can be used to track cells in vivo. For instance, to avoid nanoparticle uptake by the mononuclear phagocytic system (MPS), mesenchymal stem cells were labeled with gold nanoparticles prior to injection to track vascular repair in a rat hind limb ischemia model through photoacoustic spectroscopy.7 The increasing use of nanoparticle-based labeling approaches to track cells in vivo raises questions on how such labeling can affect cellular physiology.
Gold nanoparticles (AuNP) are among the most exploited nanosized materials in biomedical approaches for cancer theranosis,9,10 mostly due to the wide possibility for chemical modification and their tunable optical and thermal capacity.11–13 In general, bare gold nanoparticles do not exhibit significant cell toxicity in a wide concentration range, facilitating their expanded use.14 Nonetheless, gold nanoparticle internalization by cells can trigger numerous stress-associated molecular pathways in a sublethal range, e.g., metabolism, cell migration and invasion, inflammation, etc. Naked gold nanoparticles inhibited vascular smooth muscle cell migration by compromising cell adhesion.15 Similarly, citrated gold nanoparticles disrupted actin filament architecture, thus inhibiting human dermal fibroblast adhesion and motility.16 In another study, gold nanoparticles modified the secretome of pancreatic cancer and pancreatic stelatte cells, hence dysregulating the bidirectional communication between these cell types in the tumor niche that led to a clear inhibition of tumor growth.17 Gold nanoparticles also attenuated tumor metastasis by disrupting epithelial-to-mesenchymal transition and normalizing the tumor vascular network.18 All these studies highlight the need for understanding how AuNP interact with different cell types of the tumor microenvironment and how they affect cell migration in tumors. Although gold nanoparticles appear to affect the cell cytoskeleton and cell migration, there is no direct evidence of the molecular mechanisms supporting such facts.19,20 Therefore, a molecular insight into this phenomenon would contribute to the comprehension of the effect of intrinsic biological activities of gold nanoparticles on cell motility.
Cell migration and invasion rely on complex dynamic processes that modulate the cytoskeleton architecture. Matrix-adhesive structures such as focal adhesions, podosomes and invadosomes are essential for cell movement and integrate most external signals so that cells can modulate morphological changes as they move both in two-dimension and three-dimension environments.21,22 These F-actin rich structures are dynamic and contain a complex network of functional, regulatory and scaffold proteins that modulate the cyclic assembly and disassembly processes in a tightly controlled manner.23–25 External stress signals can trigger cytoskeleton responses which allow cells to respond to environmental cues. Since nanoparticle uptake and transit through the cellular compartment can stress cells and induce subcellular morphology changes,26 we sought to decipher the dynamics of matrix-adhesive F-actin structures (collectively called adhesomes) upon AuNP uptake and investigate consequences for extracellular matrix (ECM) degradation. We used spherical citrated AuNP in a wide range of diameter (3–40 nm) as these canonical nanosystems did not show significant toxicities in our hands. For adhesome study, we chose macrophages to investigate podosome dynamics since cells from the MPS exhibit a podosome-dependent migration/invasion process; and endothelial and mesenchymal stem cells to study focal adhesions (FA) as these structures play a pivotal role in the motility and extracellular matrix (ECM)–cell interaction of these cells (Scheme 1). Macrophages exhibit an intermediate speed (1 μm min−1 up to 10 mm min−1 upon inflammation in vivo) supported by mesenchymal and amoeboid movements, and largely depend on actin-rich dot-like structures (podosomes) and their coalescent supra-structures (clusters and rosettes). This organization favors the active and fast degradation of ECM during mesenchymal movement within dense matrices helped by enzymes. Endothelial and mesenchymal stem cells exhibit a rather slow movement (0.1–0.5 μm min−1) as compared to immune system cells, such as macrophages, and largely rely on bundled actin-rich structures (focal adhesions) that not only attach cells to ECM (integrins), but also connect ECM with actin bundles of intracellular stress fibers. This allows cells to attach to ECM more strongly than, for instance, macrophages, as they form more complex tissues such as blood vessels. These cells’ (endothelial and mesenchymal) movement type is referred to as mesenchymal, and other cells, such as fibroblasts, exhibit this ECM degradation-dependent movement. We demonstrated for the first time that AuNP disturbed the dynamics of adhesomes, i.e., podosome and FA, and modified ECM degradation. Most importantly, this phenomenon appeared to be nanoparticle core size- and cell type-dependent.
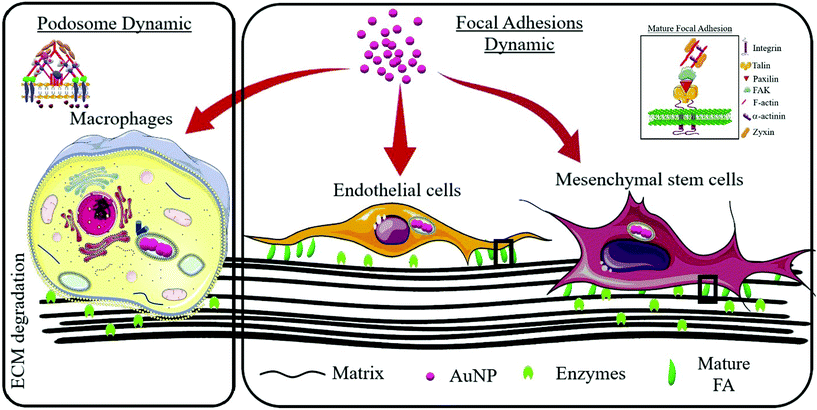 |
| Scheme 1 General experimental approach to investigate adhesome disturbance after gold nanoparticle internalization. Podosomes (left inset) are an integral part of monocyte/macrophage migration and invasion processes and thus a cell model from this lineage was used to study gold nanoparticle effects on podosome formation and activity. Focal adhesions (FA, right inset) are important in endothelial and mesenchymal stem cell functions; therefore, these cell types were used to explore FA dynamics. | |
2. Experimental section
2.1. Cell culture
The murine macrophage cell line RAW264.7 (ATCC, TIB-71) and SV40-transformed endothelial cells SVEC4-10 7 (ATCC, CRL-2181) as well as the murine mesenchymal stem cell line C3H10T1/2 (ATCC, CCL-226) were cultured in DMEM supplemented with 10% fetal bovine serum (FBS), penicillin/streptomycin, L-glucose and sodium pyruvate.
2.2. Reagents and antibodies
LPS (L4005) and phalloidin-TRITC (P1951) were purchased from Sigma-Aldrich. Primary antibodies were anti-phospho-p44/p42 MAPK (9102S), -phospho-cortactin (Y421) (4569S), -MT1-MMP (13130S), and -phospho (Tyr416)-Src (6943S) (all from Cell Signaling), anti-human vinculin (V9131) and anti-zyxin (Z4751) from Sigma-Aldrich; and DAPI (T3605) from Invitrogen. Secondary antibodies were Alexa488-goat anti-mouse IgG (A-11029) and Alexa488-goat anti-rabbit IgG (A-27034) and Alexa488-stained pig gelatin, all purchased from Invitrogen.
2.3. Citrate-coated gold nanoparticle synthesis
Gold nanoparticles (AuNP) were synthesized following a Turkevich protocol as stated elsewhere,27 based on the synthesis of 10 nm gold seeds followed by sequential seed growth. Briefly, sodium citrate (97 mg) was dissolved in 150 mL of Milli-Q water and boiled under magnetic stirring for 15 minutes followed by the addition of 1 mL of pre-heated 25 mM gold chloride solution. After 10 minutes, the reaction was cooled down to 70 °C and then diluted by extracting 55 mL of the AuNP solution and replenished with Milli-Q water (53 mL) and sodium citrate (2 mL, 60 mM). Then, gold chloride (1 mL, 25 mM) was added followed by a second addition after 30 min. The procedure was repeated until the desired AuNP sizes were obtained, e.g. once for the 16 nm, 3 times for the 30 nm, and 7 times for the 40 nm. The smallest AuNP (3 and 11 nm) were synthesized in the presence of tannic acid.28 Briefly, sodium citrate (97 mg) and potassium carbonate (20.7 mg) were dissolved in 150 mL of water and mixed with a tannic acid solution (1 mL, 2.5 mM). After heating at 70 °C under magnetic stirring, 1 mL of 25 mM gold chloride solution was added, and the reaction was stopped when the solution turned orange.
The AuNP were characterized using transmission electron microscopy (TEM). TEM images of aqueous dispersion were obtained with a JEOL JEM-1400PLUS transmission electron microscope operating at an acceleration voltage of 120 kV (Jaques Monod Institute, Paris, France).
2.4. Cytokine secretion assay
RAW264.7 (ATCC, TIB-71) were seeded on 24-well plates and treated with 10 μg ml−1 AuNP for 24 h, and supernatants were collected. Lipopolysaccharide (LPS) was used as a positive control (10 μg ml−1). The levels of murine secreted IL-10 (DuoSet ELISA DY417-05, R&D Systems), IL-6 (DuoSet ELISA DY406-05, R&D Systems), RANTES/CCL5 (DuoSet ELISA DY478-05, R&D Systems), and TNFα (DuoSet ELISA DY410-05, BD Biosciences) were quantified according to the manufacturer's instructions. Levels of TIMP1 (Mouse TIMP1 ELISA Kit, RAB0468, Sigma-Aldrich), TIMP2 (DuoSet ELISA DY6304-05, BD Biosciences), MMP2 (Mouse MMP2 ELISA Kit, RAB0366, Sigma-Aldrich), and SerpinE1 (DuoSet ELISA DY3828-05, BD Biosciences) were determined following the manufacturer's protocol. Absorbance was read with a Multimode Plate Reader (EnSpire, PerkinElmer). Triplicate samples were analyzed.
2.5. Cell TEM imaging
After incubation with AuNP for 24 h, cells were harvested, washed 3× with PBS 1×, and resuspended in fixing buffer (0.1 M sodium cacodylate, 2.5% glutaraldehyde). Cell suspensions were mixed gently for 1 h at 4 °C. After being washed twice, cells were resuspended in 0.1 M sodium cacodylate buffer until the TEM procedure. Samples were then contrasted with Oolong Tea Extract (OTE; 0.5%) in cacodylate buffer, post-fixed with 1% osmium tetroxide containing 1.5% potassium cyanoferrate, gradually dehydrated in ethanol (30% to 100%) and substituted gradually in a mix of ethanol and Epon and embedded in Epon (Delta Microscopie, Labège, France). Thin sections (70 nm) were collected onto 200 mesh copper grids, and counterstained with lead citrate. Grids were examined using a Hitachi HT7700 electron microscope operated at 80 kV (Elexience, France), and images were acquired using a charge-coupled device camera (AMT, MIMA2 MET – GABI platform, INRA, Agroparistech, 78352 Jouy-en-Josas, France).
2.6. Confocal microscopy
Images were captured using an Olympus TRI 514 AN0005 457/515/647 DUAL DM 488/532 confocal microscope with a 63×/1.4 NA water immersed objective and cells were viewed using an Andor Technology with an Olympus JX81/BX61 Device/Yokogawa CSU device (Andor Technology Plc, Belfast, Northern Ireland) using appropriate filters. Specific staining and analysis were carried out as follows:
Podosome/focal adhesion visualization and quantification.
RAW264.7 cells (ATCC, TIB-71) were incubated with 10 μg ml−1 AuNP for 30, 60, 120 min and 24 h when necessary, fixed, permeabilized, and stained for vinculin (mouse anti-vinculin) and F-actin (phalloidin-TRITC); nuclei were contrasted by DAPI staining (Prolong Diamond Antifade Mountant with DAPI, Invitrogen, P36962). For mature FA, zyxin was co-stained with F-actin, instead of vinculin. Images were processed using ImageJ. Cell mask and podosome/FA counts were obtained using the F-actin channel from images acquired at the ventral part of the cells following the software-based analysis described in ref. 29 and further described in the ESI.†
Phospho-cortactin, phospho-p44/p42 and phospho-Src analysis.
RAW264.7 cells were incubated with 10 μg ml−1 AuNP for 30, 60, 90, and 120 min, fixed, permeabilized, and stained for phospho-Src (rabbit anti-mouse), phospho-p44/p42 (rabbit anti-mouse) or phospho-cortactin (rabbit anti-mouse) and F-actin (phalloidin-TRITC); nuclei were contrasted by DAPI staining (ProLong Diamond Antifade Mountant with DAPI, Invitrogen, P36962). Images were processed using ImageJ, and colocalization was analyzed with a colocalization plugin (developed at the Institute Jacques Monod, Paris, France), as further explained in the ESI.† Arbitrary 8-bit intensity of colocalized pixels was used for comparison.
2.7. Gelatin degradation assay
For the fluorescent gelatin degradation assay, gelatin was prepared as described in ref. 30. Briefly, Alexa Fluor 488-gelatin type A from porcine skin (1 mg ml−1, Sigma-Aldrich) was diluted at 60 °C in 1× PBS containing 2% sucrose to obtain a solution of 0.2 mg ml−1. Coverslips (12 mm) were coated with 15 μL pre-warmed diluted fluorescent gelatin and dried out on air; gelatin was then crosslinked with 0.5% glutaraldehyde in PBS for 15 min, washed with PBS and quenched with 5 mg ml−1 sodium borohydride (3 min). Coverslips were sterilized with 70% ethanol and UV and kept in antibiotic-containing sterile PBS until use. Cells were harvested, counted and cultured on coverslips (50 × 103 cells) alone or incubated with 10 μg ml−1 AuNP in supplemented DMEM for 24 h, washed, fixed and processed for immunofluorescence. Non-fluorescent areas (degraded gelatin) were counted by examining >25 random fields imaged with a 63× objective in three independent experiments. Images of the AlexaFluor488 channel were analyzed using ImageJ according to a gelatin degradation analysis macro described in the ESI.†
2.8. Statistical analysis
For all experiments requiring image analysis, triplicates (n = 3, >25 images, thus >100 cells were analyzed per replicate and condition, for a total of at least three independent experiments) were analyzed using ImageJ Fiji software (NIH, USA) and primary data were retrieved in Excel format. Pre-processing (rosette-positive cell percentage calculation, the number of podosomes per cell and podosome density, as well as phospho-cortactin, phospho-Src normalization) was carried out in Microsoft Excel and the resulting data were analyzed and depicted using GraphPad Prism software. Data are presented as the mean ± SEM, and the number of samples and independent experiments are stated in each figure caption for precision. Data were analyzed using a two-tailed Mann–Whitney test under the assumption of a non-Gaussian distribution (nonparametric test), with 95% confidence, and *p < 0.05, **p < 0.01, and ***p < 0.001. When stated, treatment groups were compared by using a two-way ANOVA test with Bonferroni correction, *p < 0.05, **p < 0.01, and ***p < 0.001, and 95% confidence. Also, when stated, all groups were compared to the control by using a one-way ANOVA test with Dunnett's post-test, *p < 0.05, **p < 0.01, and, ***p < 0.001, and 95% confidence.
3. Results and discussion
3.1. Gold nanoparticle internalization and cytokine secretion by macrophages
The mononuclear monocytic system (MMS), which comprises mainly monocytes/macrophages, is one of the first protective barriers of the innate immune system. These cells act as sentinels in tissues, survey their surroundings and scavenge potential threats such as viruses and bacteria.31 As a result, macrophages express and secrete a wide and versatile cytokine profile that usually counteracts the threats. Nanomaterials are foreign agents that often trigger an innate immune response.32 We thus sought to determine the effect of gold nanoparticles (AuNP) on the cytokine profile secreted by macrophages. After 24 h of incubation with citrated AuNP of core diameter 3, 11, 16, 30, and 40 nm (Fig. S1†), the murine macrophage cell line RAW264.7 clearly presented endosome/lysosome-confined intracellular nanoparticle clusters (Fig. S2a–e†) with no significant cell death (Fig. S2f†), regardless of particle size. We observed an increase in soluble pro-inflammatory cytokine TNFα in 24 h AuNP-treated RAW264.7 cell supernatants (Fig. S3a†), while AuNP did not induce secretion of the anti-inflammatory IL-10, contrary to the positive control lipopolysaccharide (LPS) that triggered the secretion of both cytokines (Fig. S3b†). Macrophage production of the monocyte chemoattractant RANTES/CCL5 depended on AuNP size, i.e., AuNP11 nm significantly decreased CCL5 secretion while AuNP16 nm had the opposite effect (Fig. S3c†). No effect was observed on IL-6 level after AuNP treatment (Fig. S3d†). Overall, the most stringent effect of AuNP internalization by macrophages is an enhanced secretion of pro-inflammatory cytokine TNFα above the level of cells treated with LPS.
3.2. Gold nanoparticles disturb macrophage podosome dynamics
Ultrastructural observations of cells by TEM show that AuNP endocytosis drives intracellular nanoparticle agglomeration within endosomal compartments (Fig. S2†). Intracellular concentration of other inorganic nanoparticles, such as iron oxide nanoparticles,33 has been linked to the disturbance of the cytoskeleton. Moreover, it is increasingly accepted that nanoparticle endocytosis triggers cytoskeleton re-arrangement as nanoparticle-loaded endosomes interact with actin during endocytosis and trafficking dynamics.34,35 We thus hypothesized that AuNP internalization affects the dynamics of macrophage cytoskeleton. As part of the latter, adhesomes play an important role in cell biology by cueing external signals to trigger intracellular molecular signalosomes associated with several functions, e.g., metabolism, stress-induced response, and cellular movement. Macrophages indeed depend on podosomes, invasion-mediating adhesions belonging to the adhesome family, to migrate and/or invade nearby tissues. Therefore, podosomes act as a complex molecular frontier between the extracellular matrix and cells in both 2D and 3D architectures. Thus, to analyze these molecular nanocomplexes, we investigated the ECM–cell contact interface, i.e., the cell ventral z-plane in 2D cell culture, where podosomes dynamically assemble and disassemble to transduce extracellular signals and mediate cell movement.25,36 Upon external signal, the MAPK pathway is activated, thereby triggering a cell response to the threat.37 We indeed detected a significant accumulation of phospho-p44/42 MAPK, also known as p-Erk, phosphorylated at activation loop residues Thr202/Tyr204, at the cell–ECM interface after 120 minutes of AuNP treatment (Fig. 1a). This effect is already observed after 30 minute treatment for the largest AuNP (16 nm, 30 nm, and 40 nm), while all the AuNP induced an increase of p-Erk after 120 minutes in comparison with untreated cells which decrease their amount of p-Erk in the same time (Fig. 1b). Concomitantly, p-Erk colocalized more abundantly with clusters of filamentous actin (F-actin) as shown in Fig. 1c, indicating p-Erk recruitment to adhesome cores upon AuNP treatment. It is indeed well documented that the Erk pathway plays a pivotal role in podosome assembly not only by inducing phosphorylation of the actin-associated protein cortactin38 through the liberation of cortactin-SH3 domains, but also by triggering the recruitment of PKCζ and MMP9 to podosomes.39
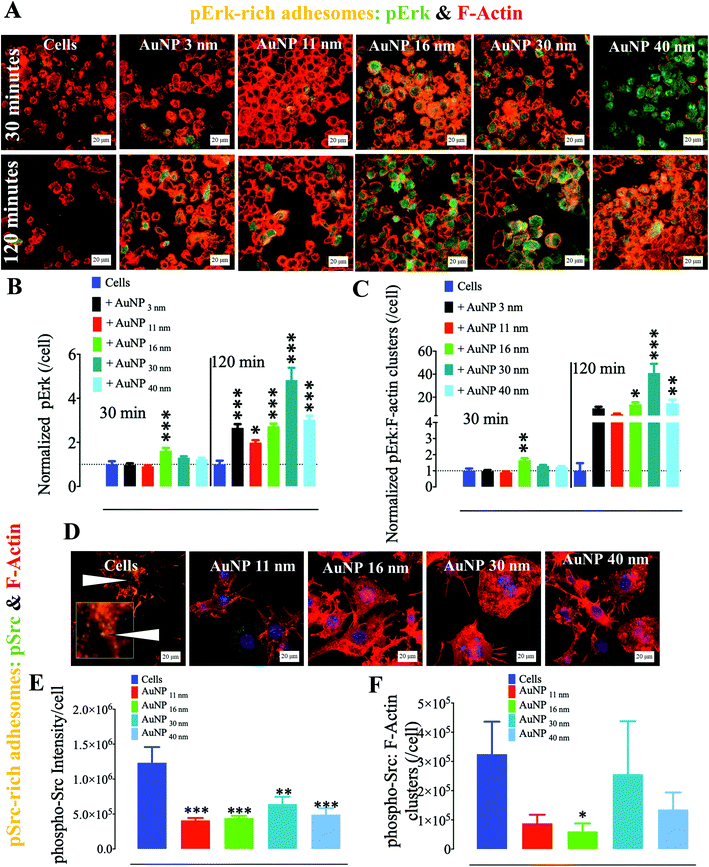 |
| Fig. 1 Effect of AuNP on phosphorylated Erk (p44/p42) and phosphorylated Src at the ventral plane (cell–surface contact). RAW264.7 macrophages were incubated with AuNP (10 μg ml−1), and stained for phospho-Erk (AlexaFluor488: green) or phospho-Src (AlexaFluor488) and filamentous actin (phalloidin: red) at 30 and 120 min. (A) Visualization of F-actin and phospho-Erk by confocal laser scanning microscopy (CLSM). Scale bar, 20 μm. (B) Quantification of phospho-Erk per cell at the cell–surface contact (n = 3, >75 images). Values are normalized against the level of phospho-Erk in untreated cells at each time point. (C) Quantification of phospho-Erk localizing with F-actin at the cell–surface contact per cell (n = 3, >75 images). Values are normalized against the level of phospho-Erk colocalized with F-actin in untreated cells at each time point. (D) Visualization of F-actin and phospho-Src by CLSM at 120 min (yellow, white arrows, and inset). (E) Quantification of phospho-Src at the cell–surface contact per cell at 120 min. Scale bar, 20 μm. (F) Quantification of cell ventral phospho-Src colocalized with F-actin per cell at 120 min (n = 3, >75 images) (*p, **p, ***p; a one-way ANOVA test with Dunnett's post-test for comparison of all columns with the control was applied). Each experiment described here was carried out in triplicate (biological) (n = 3), and the results are expressed as the mean ± SEM. For each replicate, >25 images (when necessary) were taken to analyze >100 cells. Data are representative of at least three independent experiments. | |
Another critical kinase involved in podosome regulation is the lipid-anchored Src kinase.38 Src kinase targets numerous substrates including the actin regulatory protein cortactin. Cortactin phosphorylation at Y421 and Y466 residues by Src facilitates the interaction of cortactin with actin polymerization-associated proteins N-WASP, Arp2/3, and Nck-1, thereby leading to the formation and maturation of podosomes and other adhesions.40 Cortactin can also be phosphorylated by serine/threonine kinases, such as phospho-Erk, at S405 and S418 residues, liberating the SH3 domain (SRC Homology 3 Domain) and facilitating the cortactin-N-WASP and -Arp2/3 interactions, hence the persistence of adhesome formation.41 Nonetheless, if a previously phosphorylated cortactin by phospho-Erk is concomitantly phosphorylated by activated (i.e., phosphorylated at Y416) Src, the activation of N-WASP and Arp2/3 terminates and podosome disassembly takes place.38 This negative feedback is known as the Erk/Src cortactin switch (Scheme 2). We observed that activated Src not only accumulated less in the ECM–cell contact area (Fig. 1d and e) after 120 minutes, but also colocalized less with F-actin-rich clusters (Fig. 1d and f). Consistently, we observed an impact of AuNP on phosphorylated cortactin dynamics and activity at the ECM–cell interface. The level of phosphorylated (Y421) cortactin at the cell–surface contact of AuNP-treated macrophages surpassed the level in the untreated cells after 2 hour treatment (Fig. 2a and b). Concomitantly, the number of ventral F-actin-rich clusters colocalizing with phospho-cortactin was ten-fold higher after two hours treatment with AuNP regardless of particle size (Fig. 2c). Since the induction of phospho-cortactin recruitment to the cell–ECM interface coincided with an increment in phospho-Erk and a decrease in phospho-Src, it is plausible that AuNP are affecting the Erk/Src cortactin switch, i.e., AuNP inhibit the accumulation of activated Src at 120 min at the cell–ECM contact through an unknown mechanism and therefore the negative effect on phospho-Erk-dependent cortactin activation disappears. Indeed, podosome assembly into rosettes was promoted by AuNP treatment during the 2 hours of observation (Fig. 2d). Altogether, AuNP trigger a change in the Erk/Src cortactin switch that promotes the podosome/rosette formation by decreasing the level of phospho-Src and, therefore, abrogating its inhibitory effect on phospho-Erk-activated cortactin (Scheme 2).
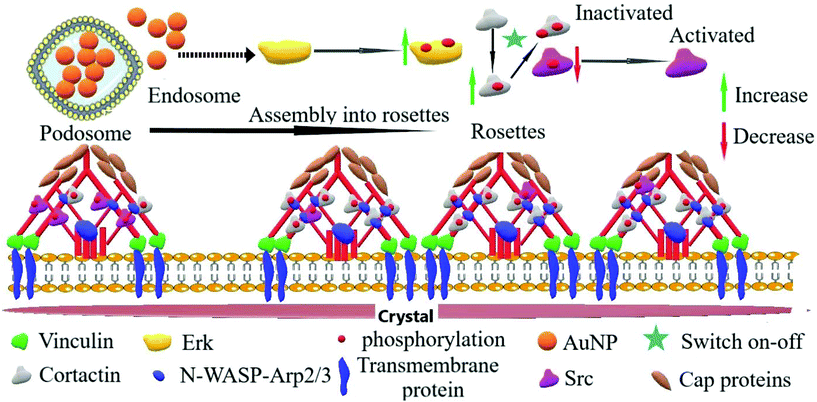 |
| Scheme 2 Proposed mechanism through which AuNP modulate podosome/rosette dynamics by controlling the Erk/Src cortactin switch on–off molecular pathway. Upon AuNP uptake, the Erk (p44/p42 MAPK) is phosphorylated and recruited to the podosome machinery where, in turn, it phosphorylates the actin-associated regulatory protein cortactin. Through an unknown pathway, AuNP internalization decreases the recruitment of phospho-Src, an inhibitor of Erk-activated cortactin; thus, AuNP favor the persistence of recruitment of activated cortactin to the podosome machinery. These events disturbed podosome dynamics leading to increased podosome rosette assembly. | |
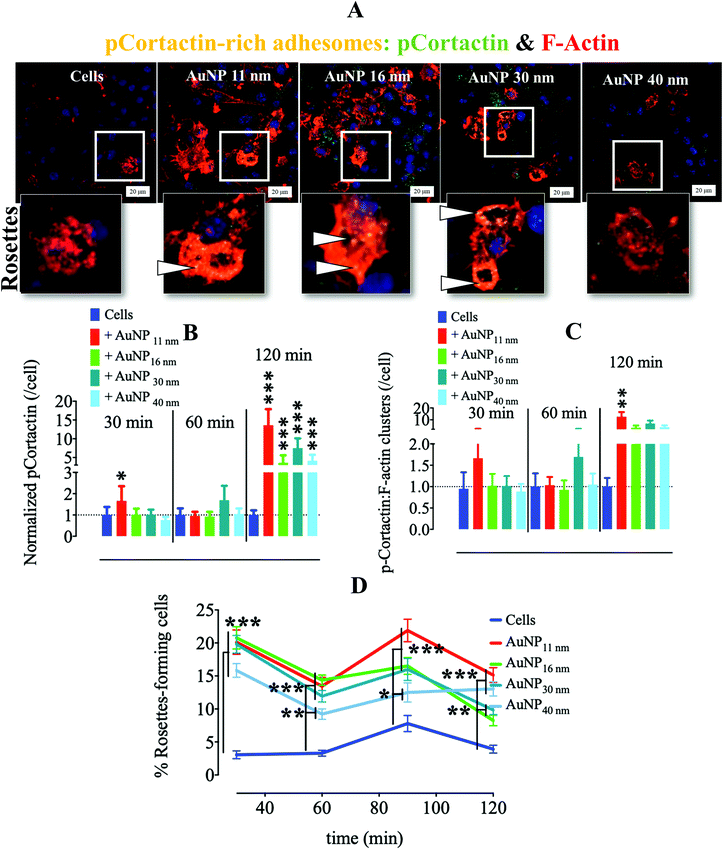 |
| Fig. 2 Effect of AuNP on podosome and phosphorylated cortactin at the adhesome plane (cell–surface contact). RAW264.7 macrophages were incubated with AuNP (10 μg ml−1), and stained for phospho–cortactin (AlexaFluor488: green) and filamentous actin (phalloidin: red) at 30, 60, 90 and 120 min. (A) Visualization of RAW264.7 cells (120 min) by CLSM. Zoomed-in images (lower) show characteristic podosome-formed rosettes with phospho-cortactin-rich areas (yellow, white arrows). Scale bar, 20 μm. (B) Quantification of phospho-cortactin at the cell–surface contact per cell. A one-way ANOVA test with Dunnett's post-test for comparison of all columns with the control was applied, *p < 0.05, **p < 0.01, and, ***p < 0.001, 95% confidence (n = 3, images >75). Values are normalized against level of phospho-cortactin in untreated cells at each time point. (C) Quantification of phospho-cortactin colocalizing with filamentous actin at cell–surface contact per cell. A one-way ANOVA test, with Dunnett's post-test for comparison of all columns with control was applied; *p < 0.05, **p < 0.01, and, ***p < 0.001, 95% confidence (n = 3, images >75). Values are normalized against the level of phospho-cortactin colocalized with filamentous actin in untreated cells at each time point. (D) Percentage of rosette-expressing cells over time. Curves were compared to the control by a two-way ANOVA test with Bonferroni correction; *p < 0.05, **p < 0.01, and, ***p < 0.001, 95% confidence (n = 3, images >75). Each experiment described here was carried out at least in triplicate (biological) (n = 3), and the results are expressed as the mean ± SEM. For each replicate, >25 images (when necessary) were taken to analyze >100 cells. Data are representative of at least three independent experiments. | |
3.3. Gold nanoparticles affect the extracellular matrix (ECM) degradation ability of mouse macrophages
Since podosome/rosette assembly was altered in macrophages cultured on glass in the first 2 hours of AuNP treatment, we reasoned that this could translate into a modulation of RAW264.7's ability to degrade ECM. AuNP3 nm and AuNP11 nm exacerbated macrophages’ capacity to degrade collagen-rich pig gelatin as compared to untreated cells. Conversely, AuNP30 nm- and AuNP40 nm-loaded macrophages showed a reduction in ECM degradation rate after 24 h (Fig. 3a and b). No significant difference in gelatin degradation was observed for AuNP16 nm-loaded cells. This effect on ECM degradation matched the variation in cell spreading and podosome/rosette assembly (Fig. 3c–e). Cell spreading on gelatin was augmented in macrophages treated with the smallest AuNP (3, 11 and 16 nm) (Fig. 3c). Smaller AuNP also boosted podosome number per cell from 4 h to 24 h treatment, while larger AuNP (40 nm) reduced podosome numbers on gelatin (Fig. 3d). This increment in podosome numbers for AuNP3 nm-, AuNP11 nm-, and AuNP16 nm-loaded macrophages coincided with an increase in the percentage of rosette-forming cells (Fig. 3e), which actively mediate ECM degradation. Conversely, podosome assembly into rosettes was disrupted in AuNP30 nm- and AuNP40 nm-loaded cells from 4 h after treatment (Fig. 3d). Altogether, smaller AuNP provoke an enhancement in ECM degradation by macrophages while larger nanoparticles have the opposite effect. The effects of AuNP on ECM degradation are likely due to their capacity to modulate podosome/rosettes dynamics (Scheme 3). According to this finding we can speculate that once small AuNP are injected in vivo, AuNP internalization by resident macrophages would trigger an enhancement of their migration through ECM degradation. ECM degradation and softening can in turn have numerous consequences, e.g., increment of tumor-infiltrating lymphocytes (including macrophages themselves) or higher drug penetration, all facilitating tumor immune response or therapy efficacy. However, if the exacerbated ECM degradation is not desired in certain injured tissues such as inflamed articulations (arthritis), larger AuNP might be safer as they do exhibit the opposite effect. Therefore, how AuNP affect macrophage motility would indicate whether their use is advised under certain pathological conditions.
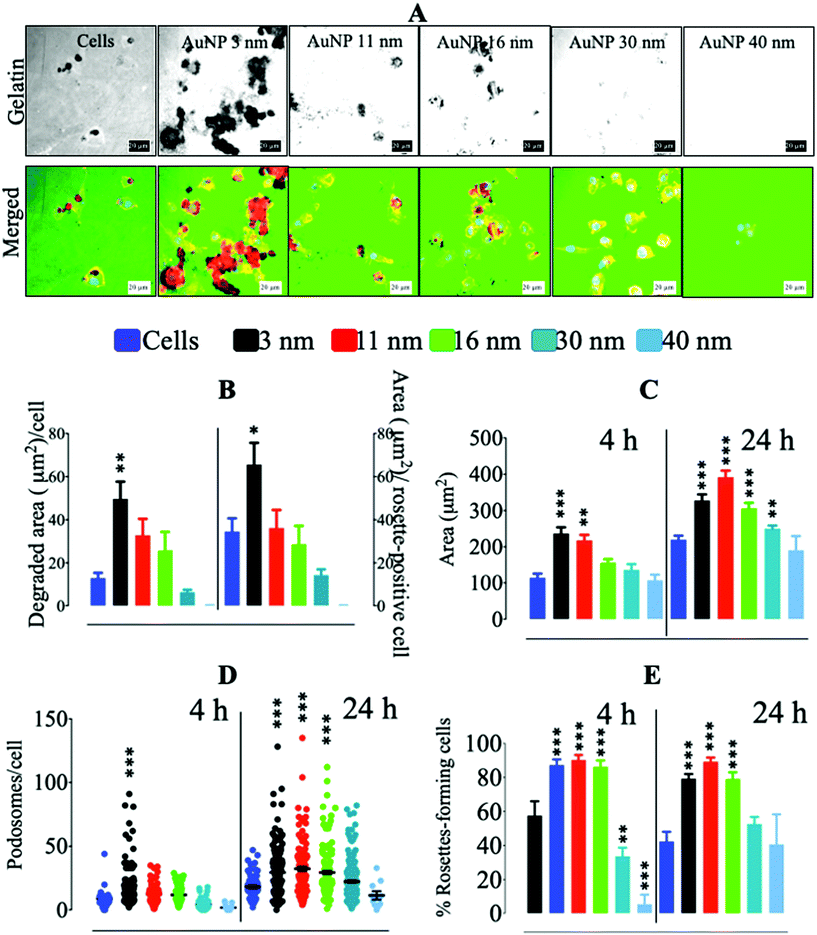 |
| Fig. 3 Gelatin degradation by AuNP-treated macrophages. (A) Alexa488-stained pig collagen gelatin degradation by AuNP-treated RAW264.7 macrophages. Scale bar, 20 μm. (B) Quantification of gelatin degradation per cell (left) and per rosette-positive cell. (C) Effect of AuNP on RAW264.7 cell area after 4 and 24 h. (D) Podosome number per cell after 4 and 24 h culture on gelatin. (E) Rosette-forming cell percentage on gelatin after 4 and 24 h of culture (n = 3, >50 images). A one-way ANOVA test with Dunnett's post-test for comparison of all columns with the control was applied; *p < 0.05, **p < 0.01, and, ***p < 0.001, 95% confidence. Each experiment was performed at least in triplicate (biological) (n = 3), and the results are expressed as the mean ± SEM. | |
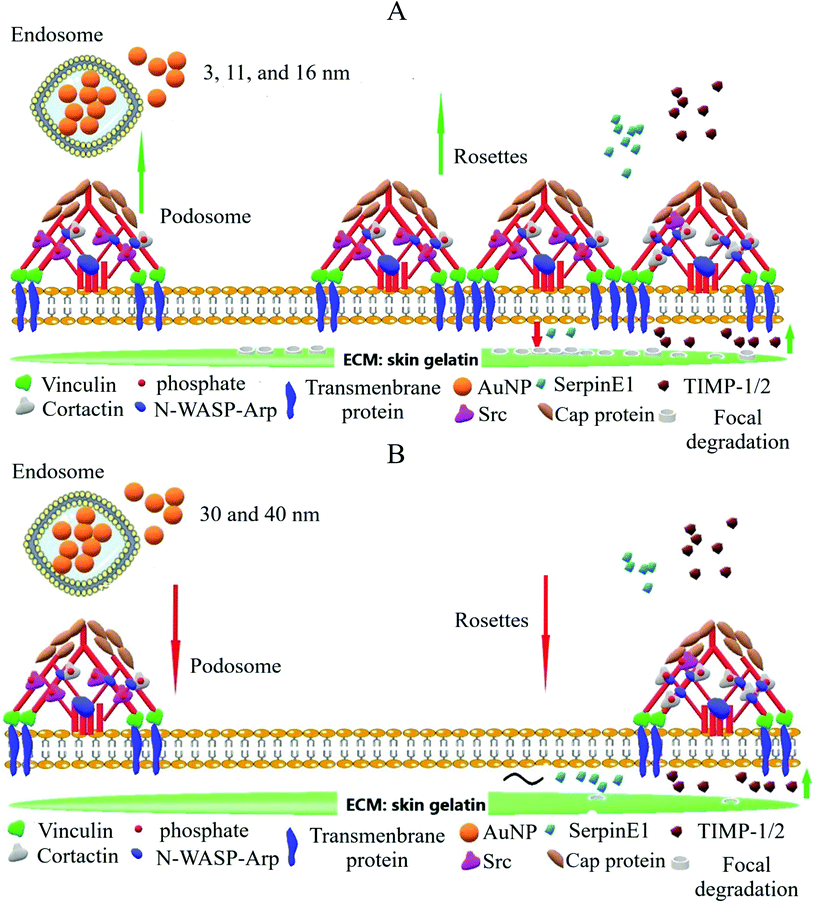 |
| Scheme 3 General overview of the effects of AuNP on ECM degradation at 24 h. (A) Smaller AuNP – 3 nm, 11 nm, and 16 nm – enhance podosome and podosome rosette assembly in macrophages, while reducing the secretion of the MMP-inhibitor SerpinE1. Altogether, these events exacerbated ECM degradation. (B) AuNP30 nm and AuNP40 nm treatment hinders podosome and rosette assembly; it triggers MMP-inhibitor TIMP-1/2 release into the supernatant and inhibits ECM degradation. | |
To gain further insight into the mechanism underlying the differences in ECM degradation that AuNP produced in macrophages, we determined the quantity of soluble factors involved in ECM degradation which are pivotal elements in podosome/rosette focal ECM degradation. Secretion of MMP2, a metalloproteinase directly involved in ECM degradation by macrophages, was not greatly affected by AuNP treatment. AuNP40 nm-loaded cells showed, nonetheless, a modest reduction in secreted MMP2 which could partially contribute to their reduced ECM degradative capacity (Fig. 4a). A significant increase in secreted tissue inhibitor of metalloproteinase-1 (TIMP1) was particularly detected after AuNP16 nm, AuNP30 nm, or AuNP40 nm treatment (Fig. 4b). Despite the persistence of podosome assembly into rosettes to a greater extent as compared to untreated cells (Fig. 3e), the increment of the metalloproteinase inhibitor for AuNP16 nm-treated macrophages partially explains the unchanged ECM degradation. Moreover, the significant decrease of podosome assembly into rosettes along with the spiking increment in the metalloproteinase inhibitor explains the hampered ECM degradation by AuNP30 nm- and AuNP40 nm-treated macrophages. Furthermore, the tissue inhibitor of metalloproteinase-2 (TIMP2) was also augmented in the supernatant of AuNP-treated cells although to a lesser extent than TIMP-1 (Fig. 4c). Secreted levels of the plasminogen activator inhibitor type-1 (PAI-1; also known as SerpinE1) decreased significantly in AuNP3 nm- and AuNP11 nm-loaded macrophages (Fig. 4d). SerpinE1 inhibits plasminogen-mediated activation of MMP in the ECM. Reduced SerpinE1 levels in the extracellular environment of AuNP3 nm- and AuNP11 nm-loaded macrophages could allow plasminogen activation of MMP and thus promote ECM degradation. To date, studies on the effect of AuNP on metalloproteinases are sparse, focusing only on the inhibition of MMP function without a mechanistic insight,42 with few reports on epigenetic/signaling modulation induced by AuNP.43 Herein, we revealed a mechanism through which AuNP can disrupt the MMP-dependent ECM degradation process in macrophages. Overall, we can conclude that gold nanoparticles of small size (3 and 11 nm) activate the assembly of podosome and rosettes at the ECM–cell interface, facilitating ECM degradation. This activity is likely supported by decreased SerpinE1 production in cells treated with small size AuNP. Conversely, larger gold nanoparticles (30 and 40 nm) decreased podosome/rosette assembly concomitantly with a significant increment in TIMP1, which probably inhibited ECM degradation (Scheme 3).
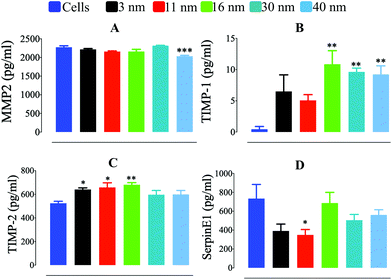 |
| Fig. 4 Quantification of supernatant (A) MMP2, (B) TIMP-1, (C) TIMP-2, and (D) SerpinE1 secreted by AuNP-treated RAW264.7 cells for 24 h (n = 6). A one-way ANOVA test with Dunnett's post-test for comparison of all columns with the control was applied; *p < 0.05, **p < 0.01, and, ***p < 0.001, 95% confidence. Each experiment was performed at least in triplicate (biological) (n = 3), and the results are expressed as the mean ± SEM. | |
Macrophage motility relies on two types of movements, i.e., amoeboid and mesenchymal. The former is triggered when macrophages encounter a porous ECM, which allows cells to glide through the inner porous space; the latter, however, is favored upon encountering dense and non-porous matrices, such as a tumor microenvironment, thereby triggering the proteolytic machinery. Such a proteolytic machinery is recruited to podosome/rosettes through which macrophages erode ECM. We have specifically demonstrated that AuNP size influences macrophage mesenchymal movement (ECM degradation, proteolytic activity), which might have a pivotal non-toxic effect on cell-based theranostics. One issue that nanomedicine has thoroughly addressed is the proper availability of drugs at tumor sites. In this regard, leukocytes have been a good ally and Trojan horses for delivery and persistence of therapeutic nanoparticles at targeted tissues, including neutrophils, T cells and macrophages,44,45 due to their unique and microenvironment-dependent tropism toward tumors and inflamed tissues.46,47 However, when loading AuNP we demonstrated that macrophage mobility can be modulated resulting in a more invasive or less invasive behavior. Therefore, AuNP can improve or hamper the ability of macrophages to erode tumor ECM depending on AuNP size. Indeed, small gold nanorod-loaded macrophages injected intratumorally showed a high tumor coverage for photothermia as compared to bare nanoparticles, most likely due to the macrophage proteolytic activity.48 It is noteworthy that it is not only intratumoral injection of AuNP-loaded macrophages that has proved to be an efficient way to guarantee tumor coverage, but also peritumoral injection whereby macrophages are able to penetrate the tumor mass and distribute gold nanoshells.49 In this sense, we speculate that smaller spherical AuNP-loaded macrophages might exhibit a high tumor penetration when injected intravenously or near the tumor; on the opposite, larger AuNP-loaded macrophages might exhibit low tumor penetration when injected intravenously, but a high tumor retention when injected intratumorally which might facilitate persistence drug delivery or photothermia. Therefore, macrophage proteolytic capacity harnessed by podosome/rosettes dynamics should be a pivotal knowledge when taken as a nanoparticle carrier for tumor therapy.
3.4. Gold nanoparticles disturb focal adhesion dynamics in murine endothelial cells
In endothelial cell (EC) biology, focal adhesions (FA) play an important role in endothelium architecture by regulating cell–cell and ECM–cell contact dynamics, determining their roles as barriers, environmental sensing intermediates, and modulators of capillary morphogenesis.50 FA differ from podosomes in the geometry and dynamics of their associated molecular machinery; while podosomes exhibit a round-shaped geometry, FA tend to be elongated when matured. Furthermore, FA appear at the end of stress fibers and are therefore largely connected to the cytoskeleton to which they transduce external signals into mechanical forces. These adhesome structures harbor a mechanosensitive machinery that modulates EC migration/invasion.51,52 Depending on cell status, FA either play as holding feeds that abrogate membrane contraction, hence promoting protrusion at leading edge, in migrating and spreading cells or provide anchorage in stationary cells.53 Among the proteins involved in FA dynamics, vinculin plays a pivotal role in FA formation by interacting with talin and actin. Such an interaction regulates FA formation and recruitment and residency of integrins (mechanosensitive proteins) in FA.54 Moreover, vinculin holds FA structure integrity in subcellular high-tension regions, while releasing it in low-tension microdomains; therefore vinculin defines the localization of fully integral FA.55 We observed AuNP within the endosomal compartment of SVEC4–10 cells incubated with 10 μg ml−1 AuNP (Fig. S4†) with negligible toxicity (Fig. S5†). We thus analyzed the effect that AuNP (16, 30, and 40 nm) internalization has on FA, defined as vinculin-positive F-actin-rich nanoclusters at the ECM–cell interface. Within the first 60 minutes of AuNP treatment, FA number per cell did not change; however, FA size significantly increased at 30 minutes as compared to untreated endothelial cells. FA size returned to the baseline after 60 minutes (Fig. 5a–c). During the FA cycle, mechanosensitive actin-associated proteins exhibit distinguishable lifespan behavior. This is the case for zyxin, a phospho-protein involved in linking of adhesion components at the cell membrane and bundles of the cytoskeleton.56 Nascent FA generated upon high-tension signal indeed lack zyxin; however, as FA grow and lose tension, there is an increment in zyxin recruitment to FA. Therefore, zyxin reliably identifies mature FA.57 We then studied AuNP's effects on mature focal adhesions (mFA, defined as zyxin positive-F-actin-rich nanoclusters). mFA number per cell decreased after 30 minutes of AuNP 16 nm, AuNP 30 nm, or AuNP 40 nm treatment (Fig. 5d–f). This effect was more pronounced at 90 minutes. mFA size did not undergo changes in the time lapse analyzed (Fig. 5f). Taking into consideration these results, we speculate that AuNP internalization might disturbed the natural balance between external mechanical and actinomycin forces (microtubules), leading to an enhancement of intracellular tension. Indeed, TiO2 and SiO2 nanoparticles perturbed intracellular forces in epithelial cells through disruption of microtubules, thereby increasing intracellular tension and retarding cell migration as cells hold tight to ECM.58 Future experiments should determine whether a similar effect of AuNP occurs on EC.
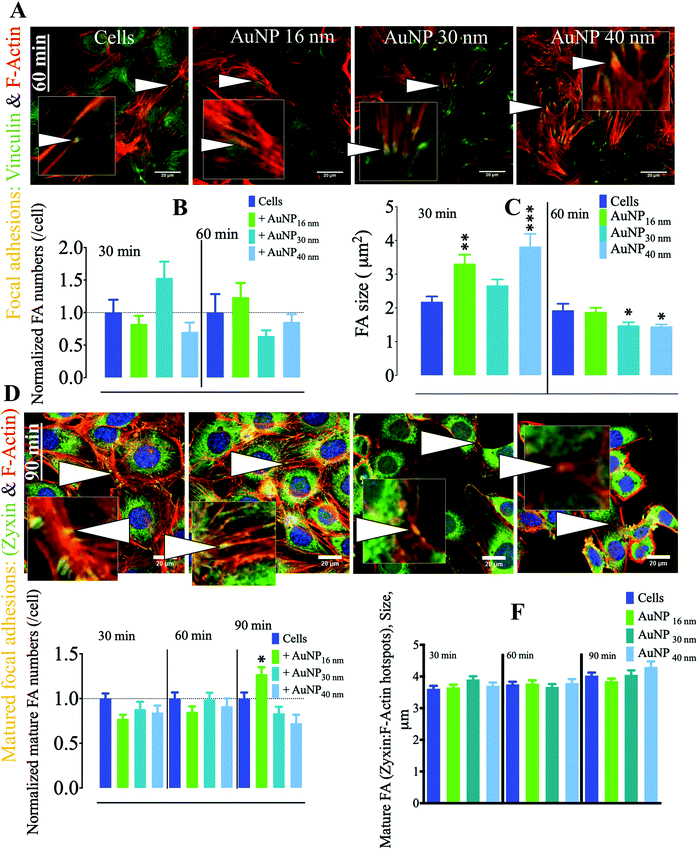 |
| Fig. 5 Effect of AuNP on focal adhesions of endothelial cells SVEC4–10. (A) Representative confocal imaging of the cell ventral area (cell–surface contact) showing the colocalization (yellow, arrowheads and insets) of F-actin (red) and the focal adhesion marker vinculin (green) at 60 minutes. Focal adhesions are defined by the colocalization of F-actin with vinculin. Scale bar, 20 μm. (B) Quantification of FA number and (C) size (n = 3, >75 images). A one-way ANOVA test with Dunnett's post-test for comparison of all columns with the control was applied; *p < 0.05, **p < 0.01, and, ***p < 0.001, 95% confidence. Each experiment was performed in triplicate (biological) (n = 3), and the results are expressed as the mean ± SEM. For each replicate, >25 images (when necessary) were taken to analyze >100 cells. Data are representative of at least three independent experiments. Values are normalized against FA number in untreated cells at each time point. (D) Representative confocal imaging of the cell ventral area (cell–surface contact) showing the colocalization (yellow, arrowheads, and insets) of F-actin (red) and the mature focal adhesion marker zyxin (green) at 90 minutes. Mature focal adhesions are defined by the colocalization of F-actin with zyxin. Scale bar, 20 μm. (E) Quantification of mature FA number and (F) size (n = 3, >75 images). A one-way ANOVA test with Dunnett's post-test for comparison of all columns with the control was applied; *p < 0.05, 95% confidence. Values are normalized against the level of mature FA number in untreated cells at each time point. Each experiment described herein was carried out in triplicate (biological) (n = 3), and the results are expressed as the mean ± SEM. For each replicate, >25 images (when necessary) were taken to analyze >100 cells. Data are representative of at least three independent experiments. | |
AuNP appeared to transiently disturb the proper maturation process of FA in endothelial cells. Since these structures are well known to be involved in EC migration/invasion,59 we determined whether AuNP can affect ECM degradation by ECs. We in fact observed a significant reduction in AuNP-loaded SVEC4–10 cell's ability to degrade collagen-rich pig gelatin (up to 2 times less) as compared to untreated ECs (Fig. 6a and b). The transmembrane metalloproteinase MT1–MMP is a master switch metalloproteinase that plays a major role in ECM remodeling and triggers the cleavage and activation of several other MMPs.60 Here, the reduced ECM degradation capacity of AuNP-loaded ECs simultaneously occurred with the decrease in membrane metalloproteinase MT1–MMP colocalization with F-actin-rich clusters, thus suggesting reduced enzymatically-active adhesomes at the ECM–cell interface (Fig. 6c and d). The extracellular level of MMP2, a metalloproteinase cleaved by MT1–MMP, decreased consistently (24 h), concurring with a significant increase in metalloproteinase inhibitors, TIMP2 and SerpinE1 (Fig. 6f–h), with no effect on TIMP-1 (Fig. S6†). It is known that tumor cells induce FA-dependent ECM degradation by phosphorylating the MT1–MMP cytoplasmic tail via Src kinase and interacting with Focal Adhesion Kinase (FAK) present in FA.59 Here, one can relate the decrease in EC capacity to degrade ECM upon treatment with AuNP, regardless of core size, to the diminution of MT1–MMP recruitment to adhesomes and to the subsequent slight reduction in soluble MMP2. This result adds new insights to the described AuNP-dependent inhibition of VEGF-dependent angiogenesis.61 Moreover, endothelial cell-secreted metalloproteinases play a pivotal role in several physiological scenarios62,63 and pathological vascular conditions.64,65 Therapeutic AuNP therefore have potential intrinsic effects by reducing MMP2-mediated angiogenesis, and more specifically the MMP-regulated new vessel sprouting.66 For instance, AuNP might hamper tumor angiogenesis, a process known to be tightly regulated by MMP2 among other factors,67 as a side-effect of therapeutic AuNP when used for photothermia or drug delivery in cancer theranostics.
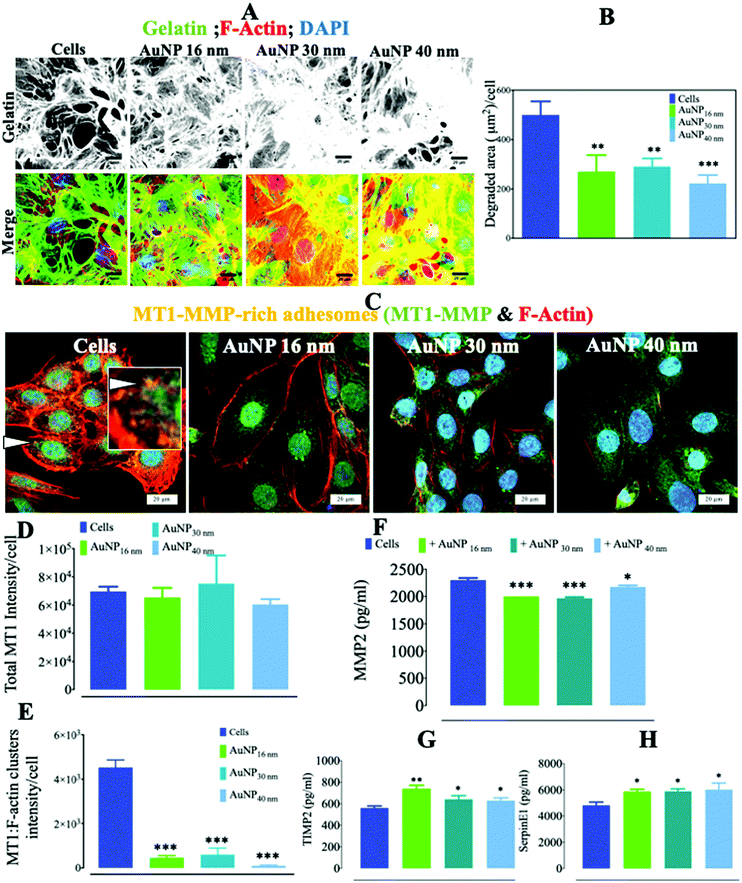 |
| Fig. 6 Gelatin degradation by AuNP-treated endothelial cells. (A) Alexa488-stained pig collagen gelatin degradation by AuNP-treated SVEC4–10 cells (n = 4, >50 images). Scale bar, 20 μm. (B) MT1–MMP (AlexaFluor488: green) colocalization with F-actin (phalloidin: red) after 24 h (n = 4, >50 images). (C) Representative confocal imaging of the cell ventral area (cell–surface contact) showing the colocalization (yellow, arrow, and inset) of F-actin (red) and MT1–MMP (green); scale bar, 20 μm. (D) Signal intensity of total MT1–MMP (green) recruited to the cell ventral area (n = 4, >50 images); and (E) signal intensity of MT1–MMP colocalized with F-actin at the ventral plane (n = 3, >50 images). ELISA quantification of (F) MMP2, (G) TIMP2, and (H) SerpinE1 secreted by AuNP-loaded endothelial cells (n = 6). A one-way ANOVA test with Dunnett's post-test for comparison of all columns with the control was applied; *p < 0.05, 95% confidence. Each experiment was performed at least in triplicate (biological) (n = 3), and the results are expressed as the mean ± SEM. For each replicate, >25 images (when necessary) were taken to analyze >100 cells. Data are representative of at least three independent experiments. | |
3.5. Gold nanoparticles’ effects on focal adhesion dynamics differ in murine mesenchymal stem cells and endothelial cells
To assess whether AuNP's effects on focal adhesions were cell dependent, we chose mouse mesenchymal stem cells (MSC) as another model, since these cells have been widely studied for their homing properties in tumors68 and tissue regeneration model.69 Contrary to endothelial cells, AuNP-treated mouse MSCs displayed an increase in the FA number and size after 60 and 120 minutes of AuNP treatment in comparison with non-treated cells (Fig. 7a–c). In addition, mature FA as measured by the colocalization of zyxin with F-actin also increased after 120 minutes of treatment, which could exacerbate ECM degradation rate in AuNP-treated mouse MSCs (Fig. 7d–f). Indeed, we observed that AuNP (30 nm, 40 nm)-treated mMSC augmented ECM degradation as compared to untreated cells (Fig. 8a and b). Therefore, AuNP's effects on FA are different in mMSC and endothelial cells (Scheme 4).
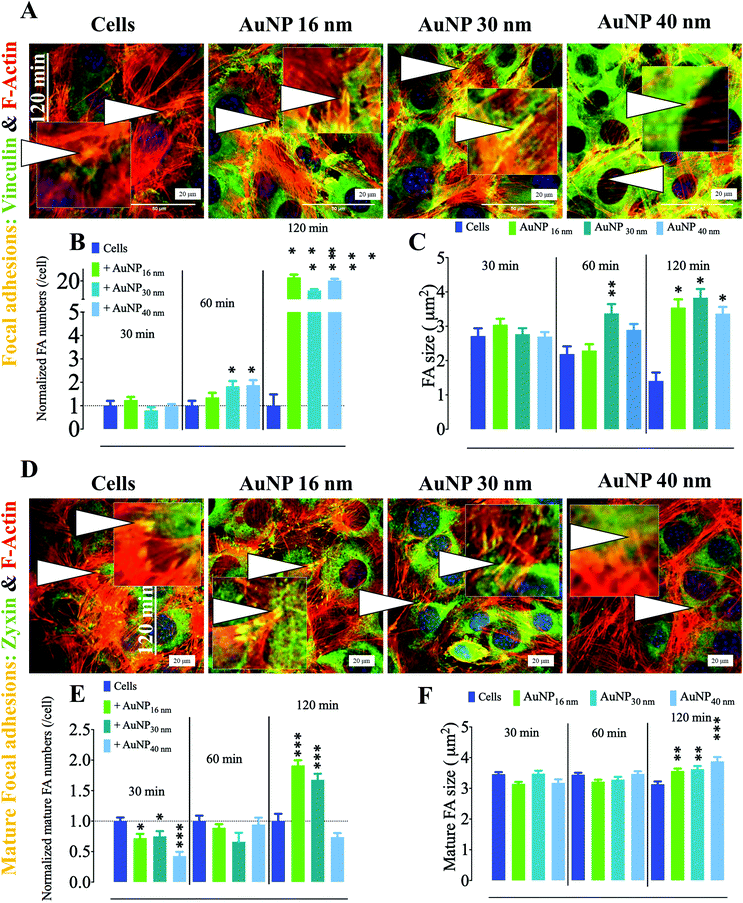 |
| Fig. 7 Effect of AuNP on focal adhesions in mesenchymal stem cells. (A) Representative confocal imaging of the cell ventral area (cell-surface contact) showing the colocalization (yellow, arrowheads and insets) of F-actin (red) and the focal adhesion marker vinculin (green). Focal adhesions are defined by the colocalization of F-actin with vinculin. Scale bar, 20 μm. (B) Quantification of FA number and (C) size (n = 3, >75 images). Values are normalized against FA number in untreated cells at each time point. (D) Representative confocal imaging of the cell ventral area (cell–surface contact) showing the colocalization (yellow, arrowheads, and insets) of F-actin (red) and the mature focal adhesion marker zyxin (green). Mature focal adhesions are defined by the colocalization of F-actin with zyxin. Scale bar, 20 μm. (E) Quantification of mature FA number and (F) size (n = 3, >75 images). A one-way ANOVA test with Dunnett's post-test for comparison of all columns with the control was applied; *p < 0.05, 95% confidence. Values are normalized against mature FA number in untreated cells at each time point. Each experiment described herein was carried out in triplicate (biological) (n = 3), and the results are expressed as the mean ± SEM. For each replicate, >25 images (when necessary) were taken to analyze >100 cells. Data are representative of at least three independent experiments. | |
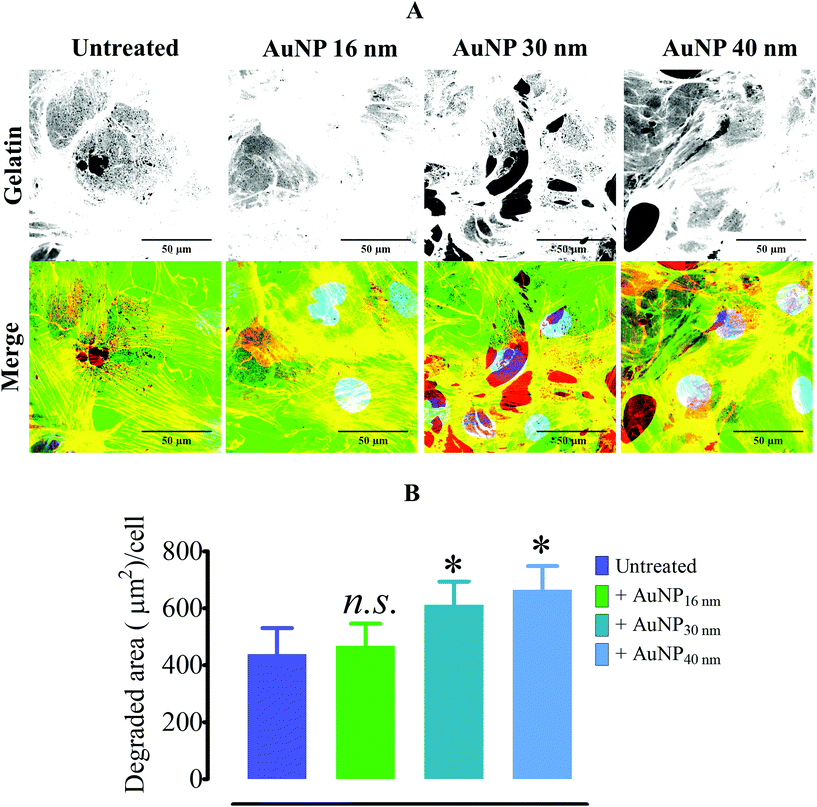 |
| Fig. 8 Gelatin degradation by AuNP-treated mesenchymal stem cells. (A) Alexa488-stained pig collagen gelatin degradation by AuNP-treated mMSC cells (n = 3, >50 images). Scale bar, 50 μm. (B) Summary of the ECM degradation assay (n = 3). A one-way ANOVA test with Dunnett's post-test for comparison of all columns with the control was applied; *p < 0.05, 95% confidence. Each experiment was performed at least in triplicate (biological) (n = 3) and expressed as the mean ± SEM. Data are representative of at least two independent experiments. | |
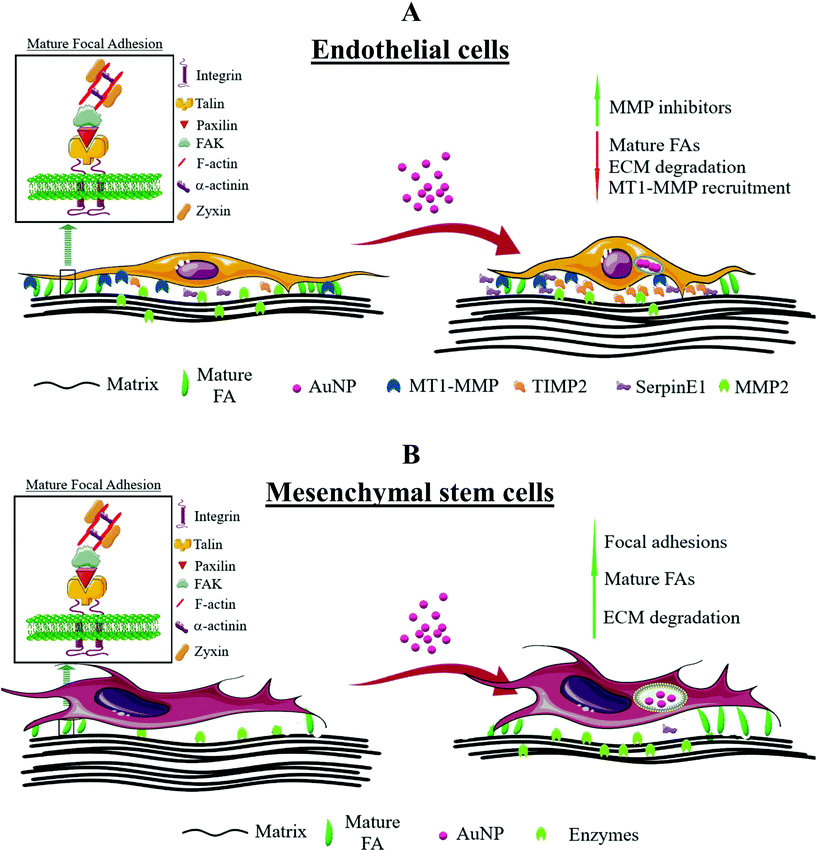 |
| Scheme 4 General overview of the effects of AuNP on focal adhesion dynamics and ECM degradation in endothelial and mesenchymal stem cells. (A) In endothelial cells, AuNP reduced the number of mature FA concomitantly with increased secretion of metalloproteinase inhibitors, TIMP-2 and SerpinE1, and decreased the production of metalloproteinase MMP2. As result, AuNP-loaded endothelial cells were less effective at extracellular matrix degradation. Conversely, (B) mouse mesenchymal stem cells increased the number and size of mature FA and, hence, the rate of extracellular matrix degradation. | |
While AuNP impaired FA maturation and ECM degradation in endothelial cells, these same AuNP promoted FA maturation and ECM degradation in mesenchymal stem cells. Such findings could have a huge implication for mesenchymal stem cell-based therapies that pursue not only the proper tissue tropism, but also AuNP delivery. During migration throughout the bloodstream and adjacent tissues,70 MSC display adhesive cell membrane microdomains to the blood vessel wall, which is related to a major focal adhesion reinforcement. Increased FA turnover has been associated with an enhancement of MSC toward SDF-1a in neuronal differentiation, highlighting the importance of FA dynamics for MSC homing.71 More importantly, this influence of FA turnover on MSC is diminished or negligible for semi- or fully differentiated MSC. Further study should address the impact of AuNP on FA dynamics on MSC at different differentiation states. FA are not only important for engraftment and regeneration of damaged tissues, but also for crosstalk between graft and host cells, and therefore nanoparticles affecting FA can in turn influence these processes. SiO2 nanoparticles indeed exacerbated FA maturation and growth in human MSC, improving the engraftment in myocardial tissue in an ex vivo infarcted rat heart.72 In addition, ECM degradation by MSC is a key event in physiological tissue remodeling, and has been linked to MSC trafficking and differentiation,73,74 both pivotal biological characteristics to MSC-based therapy. We thus hypothesize that AuNP can facilitate MSC therapeutic benefits through improving cell adhesion and ECM degradation. The theranostic use of AuNP-loaded MSC to enhance phototherapy efficiency75 or facilitate MSC tracking7 prompts the study of how AuNP might affect MSC biology to improve therapeutic outcomes. We believe that by loading therapeutic AuNP into MSCs we will be able to increase their tissue penetration capacity and, therefore, improve MSC-based tissue engraftment or drug delivery. Furthermore, when injected in vivo, AuNP could provoke the increment of ECM degradation by nascent MSC as a consequence of AuNP internalization, thereby facilitating MSC migration to the injured tissues (e.g., infarcted heart). Altogether, we demonstrated that not only AuNP disturbed FA dynamics in a size-dependent manner, but also their effect was also dependent on cell type. Such differential effects probably rely on cell type-specific biology and should prompt scientists to better understand and evaluate how AuNP loading will affect cell biology in cell-based and nanoparticle-based therapies.
4. Conclusions
Despite the large numbers of publications on cytotoxic effects of AuNP on cells, including cell migratory behavior, none of them directly address how AuNP disturb adhesome dynamics. Herein, we established that AuNP affect podosome dynamics in a murine macrophage model in a core size-dependent manner. ECM degradation was exacerbated in AuNP-treated macrophages for smaller nanoparticles (3 and 11 nm) but was inhibited in macrophages loaded with larger counterparts (30 and 40 nm) (Scheme 3). We proved that changes in podosome dynamics mediated by AuNP relied on the disturbance of the molecular degradative on–off switch based on the activation of the Erk/Src/cortactin triad (Scheme 2). Nevertheless, AuNP proved to affect FA dynamics depending on the cell type analyzed. As such, while AuNP negatively affect the maturation process of FA in endothelial cells and, thereby, inhibited ECM degradation, AuNP induced FA maturation in mouse MSC and consequently exacerbated ECM degradation (Scheme 4). Collectively, here we presented pivotal results to better understand AuNP interference with cell migration, and how these effects are dependent on AuNP size and cell type.
Conflicts of interest
The authors declare no competing financial interests.
Acknowledgements
VMA received a post-doc fellowship from the Association pour le Recherche contre le Cancer (ARC, Aides Individuelles, post-doctorant, dossier 20150603405). ANB received a PhD fellowship from the Institute thematique multi-organismes (ITMO) Cancer and the doctoral school Frontières du Vivant (FdV) – Programme Bettencourt. AB received a PhD fellowship from the doctoral school Physique en Ile de France (EDPIF). This work was supported by the ITMO-Inserm Plan Cancer 2014–2019. We are grateful to Christine Péchoux (INRA, UMR 1313, Plateforme MIMA2, Jouy en Josas, France) for electron microscopy preparation and observation.
References
- H. Lee, M. K. Yu, S. Park, S. Moon, J. J. Min, Y. Y. Jeong, H.-W. Kang and S. Jon, Thermally Cross-Linked Superparamagnetic Iron Oxide Nanoparticles: Synthesis and Application as a Dual Imaging Probe for Cancer in Vivo, J. Am. Chem. Soc., 2007, 129(42), 12739–12745 CrossRef CAS PubMed.
- M. K. Yu, J. Park and S. Jon, Targeting Strategies for Multifunctional Nanoparticles in Cancer Imaging and Therapy, Theranostics, 2012, 2(1), 3–44 CrossRef CAS PubMed.
- V. Mulens, P. Morales and D. F. Barber, Development of Magnetic Nanoparticles for Cancer Gene Therapy: A Comprehensive Review, ISRN Nanomater., 2013, 646284 Search PubMed.
- J. Kolosnjaj-Tabi, C. Wilhelm, O. Clément and F. Gazeau, Cell Labeling with Magnetic Nanoparticles: Opportunity for Magnetic Cell Imaging and Cell Manipulation, J. Nanobiotechnol., 2013, 11(Suppl 1), S7 CrossRef PubMed.
- A. A. Faraj, N. Luciani, J. Kolosnjaj-Tabi, E. Mattar, O. Clement, C. Wilhelm and F. Gazeau, Real-Time High-Resolution Magnetic Resonance Tracking of Macrophage Subpopulations in a Murine Inflammation Model: A Pilot Study with a Commercially Available Cryogenic Probe, Contrast Media Mol. Imaging, 2013, 8(2), 193–203 CrossRef PubMed.
- M. Selt, A. Tennstaedt, A. Beyrau, M. Nelles, G. Schneider, C. Löwik and M. Hoehn, In Vivo Non-Invasive Tracking of Macrophage Recruitment to Experimental Stroke, PLoS One, 2016, 11(6), 1–21 CrossRef PubMed.
- L. M. Ricles, S. Y. Nam, E. A. Trevino, S. Y. Emelianov and L. J. Suggs, A Dual Gold Nanoparticle System for Mesenchymal Stem Cell Tracking, Biophys. Chem., 2005, 257(5), 2432–2437 Search PubMed.
- P. Chhour, P. C. Naha, S. M. O'Neill, H. I. Litt, M. P. Reilly, V. A. Ferrari and D. P. Cormode, Labeling Monocytes with Gold Nanoparticles to Track Their Recruitment in Atherosclerosis with Computed Tomography, Biomaterials, 2016, 87, 93–103 CrossRef CAS PubMed.
- S. Gudlur, C. Sanden, P. Matouskova, C. Fasciani and D. Aili, Liposomes as Nanoreactors for the Photochemical Synthesis of Gold Nanoparticles, J. Colloid Interface Sci., 2015, 456, 206–209 CrossRef CAS PubMed.
- A. K. Rengan, M. Jagtap, A. De, R. Banerjee and R. Srivastava, Multifunctional Gold Coated Thermo-Sensitive Liposomes for Multimodal Imaging and Photo-Thermal Therapy of Breast Cancer Cells, Nanoscale, 2014, 6(2), 916–923 RSC.
- R. Cheheltani, R. M. Ezzibdeh, P. Chhour, K. Pulaparthi, J. Kim, M. Jurcova, J. C. Hsu, C. Blundell, H. I. Litt and V. A. Ferrari,
et al., TunableBiodegradable Gold Nanoparticles as Contrast Agents for Computed Tomography and Photoacoustic Imaging, Biomaterials, 2016, 102, 87–97 CrossRef CAS PubMed.
- W. Li and X. Chen, Gold Nanoparticles for Photoacoustic Imaging, Nanomed., 2015, 10(2), 299–320 CrossRef CAS PubMed.
- Y. Liu, J. He, K. Yang, C. Yi, Y. Liu, L. Nie, N. M. Khashab, X. Chen and Z. Nie, Folding Up of Gold Nanoparticle Strings into Plasmonic Vesicles for Enhanced Photoacoustic Imaging, Angew. Chem., Int. Ed., 2015, 54(52), 15809–15812 CrossRef CAS PubMed.
- L. A. Dykman and N. G. Khlebtsov, Gold Nanoparticles in Biology and Medicine: Recent Advances and Prospects, Acta Nat., 2011, 3(2), 34–55 CAS.
- H.-M. Lo, M.-C. Ma, J. Shieh, H.-L. Chen and W. Wu, Naked Physically Synthesized Gold Nanoparticles Affect Migration, Mitochondrial Activity, and Proliferation of Vascular Smooth Muscle Cells, Int. J. Nanomed., 2018, 13, 3163–3176 CrossRef PubMed.
- N. Pernodet, X. Fang, Y. Sun, A. Bakhtina, A. Ramakrishnan, J. Sokolov, A. Ulman and M. Rafailovich, Adverse Effects of Citrate/Gold Nanoparticles on Human Dermal Fibroblasts, Small, 2006, 2(6), 766–773 CrossRef CAS PubMed.
- S. Saha, X. Xiong, P. K. Chakraborty, K. Shameer, R. R. Arvizo, R. A. Kudgus, S. K. D. Dwivedi, M. N. Hossen, E. M. Gillies and J. D. Robertson,
et al., Gold Nanoparticle Reprograms Pancreatic Tumor Microenvironment and Inhibits Tumor Growth, ACS Nano, 2016, 10(12), 10636–10651 CrossRef CAS PubMed.
- W. Li, X. Li, S. Liu, W. Yang, F. Pan, X. Y. Yang, B. Du, L. Qin and Y. Pan, Gold Nanoparticles Attenuate Metastasis by Tumor Vasculature Normalization and Epithelial-Mesenchymal Transition Inhibition, Int. J. Nanomed., 2017, 12, 3509–3520 CrossRef CAS PubMed.
- C. Noël, J. C. Simard and D. Girard, Gold Nanoparticles Induce Apoptosis, Endoplasmic Reticulum Stress Events and Cleavage of Cytoskeletal Proteins in Human Neutrophils, Toxicol. In Vitro, 2016, 31, 12–22 CrossRef PubMed.
- Y. Liu, N. Rogel, K. Harada, L. Jarett, C. H. Maiorana, G. K. German, G. J. Mahler and A. L. Doiron, Nanoparticle Size-Specific Actin Rearrangement and Barrier Dysfunction of Endothelial Cells, Nanotoxicology, 2017, 11(7), 846–856 CrossRef PubMed.
- D. Hoshino, K. M. Branch and A. M. Weaver, Signaling Inputs to Invadopodia and Podosomes, J. Cell Sci., 2013, 126(Pt 14), 2979–2989 CrossRef CAS PubMed.
- C. Albiges-Rizo, O. Destaing, B. Fourcade, E. Planus and M. R. Block, Actin Machinery and Mechanosensitivity in Invadopodia, Podosomes and Focal Adhesions, J. Cell Sci., 2009, 122(Pt 17), 3037–3049 CrossRef CAS PubMed.
- A. S. Andersen, H. Aslan, M. Dong, X. Jiang and D. S. Sutherland, Podosome Formation and Development in Monocytes Restricted by the Nanoscale Spatial Distribution of ICAM1, Nano Lett., 2016, 16(3), 2114–2121 CrossRef CAS PubMed.
- R. Guiet, C. Verollets, I. Lamsoul, C. Cougoule, R. Poincloux, A. Labrousse, D. A. Calderwood, M. Glogauer, P. G. Lutz and I. Maridonneau-Parini, Macrophage Mesenchymal Migration Requires Podosome Stabilization by Filamin A, J. Biol. Chem., 2012, 287(16), 13051–13062 CrossRef CAS PubMed.
- D. A. Murphy and S. A. Courtneidge, The “ins” and “Outs” of Podosomes and Invadopodia: Characteristics, Formation and Function, Nat. Rev. Mol. Cell Biol., 2011, 12(7), 413–426 CrossRef CAS PubMed.
- X. Ma, R. Hartmann, D. Jimenez De Aberasturi, F. Yang, S. J. H. Soenen, B. B. Manshian, J. Franz, D. Valdeperez, B. Pelaz and N. Feliu,
et al., Colloidal Gold Nanoparticles Induce Changes in Cellular and Subcellular Morphology, ACS Nano, 2017, 11(8), 7807–7820 CrossRef CAS PubMed.
- N. G. Bastús, J. Comenge and V. Puntes, Kinetically Controlled Seeded Growth Synthesis of Citrate-Stabilized Gold Nanoparticles of up to 200 Nm: Size Focusing versus Ostwald Ripening, Langmuir, 2011, 27(17), 11098–11105 CrossRef PubMed.
- J. Piella, N. G. Bastús and V. Puntes, Size-Controlled Synthesis of Sub-10-Nanometer Citrate-Stabilized Gold Nanoparticles and Related Optical Properties, Chem. Mater., 2016, 28(4), 1066–1075 CrossRef CAS.
- V. Mulens-Arias, J. M. Rojas, S. Pérez-Yagüe, M. P. Morales and D. F. Barber, Polyethylenimine-Coated SPIONs Trigger Macrophage Activation through TLR-4 Signaling and ROS Production and Modulate Podosome Dynamics, Biomaterials, 2015, 52, 494–506 CrossRef CAS PubMed.
-
http://Www.Bio-Protocol.Org997 Vol 3, Iss 24, Dec 20, 2013. 2013, 3, 1–8.
- C. J. Stocks, M. A. Schembri, M. J. Sweet and R. Kapetanovic, For When Bacterial Infections Persist:
Toll-like Receptor-Inducible Direct Antimicrobial Pathways in Macrophages, J. Leukocyte Biol., 2018, 103(1), 35–51 CrossRef CAS PubMed.
- S. Najafi-Hajivar, P. Zakeri-Milani, H. Mohammadi, M. Niazi, M. Soleymani-Goloujeh, B. Baradaran and H. Valizadeh, Overview on Experimental Models of Interactions between Nanoparticles and the Immune System, Biomed. Pharmacother., 2016, 83, 1365–1378 CrossRef CAS PubMed.
- S. J. H. Soenen, N. Nuytten, S. F. De Meyer, S. C. De Smedt and M. De Cuyper, High Intracellular Iron Oxide Nanoparticle Concentrations Affect Cellular Cytoskeleton and Focal Adhesion Kinase-Mediated Signaling, Small, 2010, 6(7), 832–842 CrossRef CAS PubMed.
- O. Lunov, V. Zablotskii, T. Syrovets, C. Röcker, K. Tron, G. U. Nienhaus and T. Simmet, Modeling Receptor-Mediated Endocytosis of Polymer-Functionalized Iron Oxide Nanoparticles by Human Macrophages, Biomaterials, 2011, 32(2), 547–555 CrossRef CAS PubMed.
- L. Treuel, X. Jiang, G. U. Nienhaus, L. Treuel, X. Jiang and G. U. Nienhaus, New Views on Cellular Uptake and Trafficking of Manufactured Nanoparticles New Views on Cellular Uptake and Trafficking of Manufactured Nanoparticles, J. R. Soc., Interface, 2013, 20120939 CrossRef PubMed.
- C. Wiesner, V. Le-Cabec, K. El Azzouzi, I. Maridonneau-Parini and S. Linder, Podosomes in Space: Macrophage Migration and Matrix Degradation in 2D and 3D Settings, Cell Adhes. Migr., 2014, 8(3), 179–191 CrossRef.
- K. M. K. Rao, MAP Kinase Activation in Macrophages, J. Leukoc Biol., 2001, 69, 3–10 CAS.
- N. Martinez-Quiles, H.-Y. H. Ho, M. W. Kirschner, N. Ramesh and R. S. Geha, Erk/Src Phosphorylation of Cortactin Acts as a Switch on-Switch off Mechanism That Controls Its Ability to Activate N-WASP, Mol. Cell. Biol., 2004, 24(12), 5269–5280 CrossRef CAS PubMed.
- H. Xiao, X. H. Bai, Y. Wang, H. Kim, A. S. Mak and M. Liu, MEK/ERK Pathway Mediates PKC Activation-Induced Recruitment of PKCζ and MMP-9 to Podosomes, J. Cell. Physiol., 2013, 228(2), 416–427 CrossRef CAS PubMed.
- L. R. Boateng and A. Huttenlocher, Spatiotemporal Regulation of Src and Its Substrates at Invadosomes, Eur. J. Cell Biol., 2012, 91(11–12), 878–888 CrossRef CAS PubMed.
- L. C. Kelley, K. E. Hayes, A. G. Ammer, K. H. Martin and S. A. Weed, Cortactin Phosphorylated by ERK1/2 Localizes to Sites of Dynamic Actin Regulation and Is Required for Carcinoma Lamellipodia Persistence, PLoS One, 2010, 5(11), 1–13 CrossRef PubMed.
- M. Hashimoto, J. I. Sasaki, S. Yamaguchi, K. Kawai, H. Kawakami, Y. Iwasaki and S. Imazato, Gold Nanoparticles Inhibit Matrix Metalloproteases without Cytotoxicity, J. Dent. Res., 2015, 94(8), 1085–1091 CrossRef CAS PubMed.
- Y. J. Chen, Y. C. Lee, C. H. Huang and L. S. Chang, Gallic Acid-Capped Gold Nanoparticles Inhibit EGF-Induced MMP-9 Expression through Suppression of P300 Stabilization and NFκB/c-Jun Activation in Breast Cancer MDA-MB-231 Cells, Toxicol. Appl. Pharmacol., 2016, 310, 98–107 CrossRef CAS PubMed.
- S. J. Madsen, C. Christie, S. J. Hong, A. Trinidad, Q. Peng, F. A. Uzal and H. Hirschberg, Nanoparticle-Loaded Macrophage-Mediated Photothermal Therapy: Potential for Glioma Treatment, Lasers Med. Sci., 2015, 30(4), 1357–1365 CrossRef PubMed.
- L. C. Kennedy, A. S. Bear, J. K. Young, N. A. Lewinski, J. Kim, A. E. Foster and R. A. Drezek, T Cells Enhance Gold Nanoparticle Delivery to Tumors in Vivo. TL - 6, Nanoscale Res. Lett., 2010, 6 VN-re(1), 283 CrossRef PubMed.
- Z. Wang, J. Li, J. Cho and A. B. Malik, Prevention of Vascular Inflammation by Nanoparticle Targeting of Adherent Neutrophils, Nat. Nanotechnol., 2014, 9, 204 CrossRef CAS PubMed.
- M. R. Choi, K. J. Stanton-Maxey, J. K. Stanley, C. S. Levin, R. Bardhan, D. Akin, S. Badve, J. Sturgis, J. P. Robinson and R. Bashir,
et al., A Cellular Trojan Horse for Delivery of Therapeutic Nanoparticles into Tumors, Nano Lett., 2007, 7(12), 3759–3765 CrossRef CAS PubMed.
- Z. Li, H. Huang, S. Tang, Y. Li, X. F. Yu, H. Wang, P. Li, Z. Sun, H. Zhang and C. Liu,
et al., Small Gold Nanorods Laden Macrophages for Enhanced Tumor Coverage in Photothermal Therapy, Biomaterials, 2016, 74, 144–154 CrossRef CAS PubMed.
- T. D. Yang, W. Choi, T. H. Yoon, K. J. Lee, J.-S. Lee, J. H. Joo, M.-G. Lee, H. S. Yim, K. M. Choi and B. Kim,
et al., In Vivo Photothermal Treatment by the Peritumoral Injection of Macrophages Loaded with Gold Nanoshells, Biomed. Opt. Express, 2016, 7(1), 185 CrossRef CAS PubMed.
- B. Geiger, J. P. Spatz and A. D. Bershadsky, Environmental Sensing through Focal Adhesions, Nat. Rev. Mol. Cell Biol., 2009, 10(1), 21–33 CrossRef CAS PubMed.
- M. H. Wu, Endothelial Focal Adhesions and Barrier Function, J. Physiol., 2005, 569(2), 359–366 CrossRef CAS PubMed.
- S. Kanda, Y. Miyata and H. Kanetake, Role of Focal Adhesion Formation in Migration and Morphogenesis of Endothelial Cells, Cell. Signalling, 2004, 16(11), 1273–1281 CrossRef CAS PubMed.
- M. R. Morgan, M. J. Humphries and M. D. Bass, Synergistic Control of Cell Adhesion by Integrins and Syndecans, Nat. Rev. Mol. Cell Biol., 2007, 8(12), 957–969 CrossRef CAS PubMed.
- J. D. Humphries, P. Wang, C. Streuli, B. Geiger, M. J. Humphries and C. Ballestrem, Vinculin Controls Focal Adhesion Formation by Direct Interactions with Talin and Actin, J. Cell Biol., 2007, 179(5), 1043–1057 CrossRef CAS PubMed.
- A. Carisey, R. Tsang, A. M. Greiner, N. Nijenhuis, N. Heath, A. Nazgiewicz, R. Kemkemer, B. Derby, J. Spatz and C. Ballestrem, Vinculin Regulates the Recruitment and Release of Core Focal Adhesion Proteins in a Force-Dependent Manner, Curr. Biol., 2013, 23(4), 271–281 CrossRef CAS PubMed.
- M. C. Beckerle, Zyxin: Zinc Fingers at Sites of Cell Adhesion, BioEssays, 1997, 19(11), 949–957 CrossRef CAS PubMed.
- K. A. Beningo, M. Dembo, I. Kaverina, J. V. Small and Y. L. Wang, Nascent Focal Adhesions Are Responsible for the Generation of Strong Propulsive Forces in Migrating Fibroblasts, J. Cell Biol., 2001, 153(4), 881–887 CrossRef CAS PubMed.
- C. Y. Tay, P. Cai, M. I. Setyawati, W. Fang, L. P. Tan, C. H. L. Hong, X. Chen and D. T. Leong, Nanoparticles Strengthen Intracellular Tension and Retard Cellular Migration, Nano Lett., 2014, 14(1), 83–88 CrossRef CAS PubMed.
- Y. Wang and M. A. McNiven, Invasive Matrix Degradation at Focal Adhesions Occurs via Protease Recruitment by a FAK-P130Cas Complex, J. Cell Biol., 2012, 196(3), 375–385 CrossRef CAS PubMed.
- Y. Itoh, MT1-MMP: A Key Regulator of Cell Migration in Tissue, IUBMB Life, 2006, 58(10), 589–596 CrossRef CAS PubMed.
- Y. Pan, Q. Wu, L. Qin, J. Cai and B. Du, Gold Nanoparticles Inhibit VEGF 165 -Induced Migration and Tube Formation of Endothelial Cells via the Akt Pathway, BioMed Res. Int., 2014, ID418624 Search PubMed.
- L. Wang, Matrix Metalloproteinase 2 (MMP2) and MMP9 Secreted by Erythropoietin-Activated Endothelial Cells Promote Neural Progenitor Cell Migration, J. Neurosci., 2006, 26(22), 5996–6003 CrossRef CAS PubMed.
- S. Jana, K. Chatterjee, A. K. Ray, P. Dasmahapatra and S. Swarnakar, Regulation of Matrix Metalloproteinase-2 Activity by COX-2-PGE2-PAKT Axis Promotes Angiogenesisin Endometriosis, PLoS One, 2016, 11(10), 1–18 CrossRef PubMed.
- I. A. Arenas, Y. Xu, P. Lopez-Jaramillo and S. T. Davidge, Angiotensin II-Induced MMP-2 Release from Endothelial Cells Is Mediated by TNF-α, Am. J. Physiol.: Cell Physiol., 2004, 286(4), C779–C784 CrossRef CAS PubMed.
- A. H. Webb, B. T. Gao, Z. K. Goldsmith, A. S. Irvine, N. Saleh, R. P. Lee, J. B. Lendermon, R. Bheemreddy, Q. Zhang and R. C. Brennan,
et al., Inhibition of MMP-2 and MMP-9 Decreases Cellular Migration, and Angiogenesis in in Vitro Models of Retinoblastoma, BMC Cancer, 2017, 17(1), 1–11 CrossRef PubMed.
- V. W. M. Van Hinsbergh and P. Koolwijk, Endothelial Sprouting and Angiogenesis: Matrix Metalloproteinases in the Lead, Cardiovasc. Res., 2008, 78(2), 203–212 CrossRef CAS PubMed.
- R. Du, C. Petritsch, K. Lu, P. Liu, A. Haller, R. Ganss, H. Song, S. Vandenberg and G. Bergers, Matrix Metalloproteinase-2 Regulates Vascular Patterning and Growth Affecting Tumor Cell Survival and Invasion in GBM, Neuro-Oncology, 2008, 10(3), 254–264 CrossRef CAS PubMed.
- C. Xie, Z. Yang, Y. Suo, Q. Chen, D. Wei, X. Weng, Z. Gu and X. Wei, Systemically Infused Mesenchymal Stem Cells Show Different Homing Profiles in Healthy and Tumor Mouse Models, Stem Cells Transl. Med., 2017, 6(4), 1120–1131 CrossRef CAS PubMed.
- L. Bagno, K. E. Hatzistergos, W. Balkan and J. M. Hare, Mesenchymal Stem Cell-Based Therapy for Cardiovascular Disease: Progress and Challenges, Mol. Ther., 2018, 26(7), 1610–1623 CrossRef CAS PubMed.
- F. Nitzsche, C. Müller, B. Lukomska, J. Jolkkonen, A. Deten and J. Boltze, The MSC Adhesion Cascade - Insights into Homing and Transendothelial Migration, Stem Cells, 2017, 1446–1460 CrossRef PubMed.
- Y. Hu, J. Lu, X. Xu, J. Lyu and H. Zhang, Regulation of Focal Adhesion Turnover in SDF-1α-Stimulated Migration of Mesenchymal Stem Cells in Neural Differentiation, Sci. Rep., 2017, 7(1), 1–14 CrossRef PubMed.
- J. Popara, L. Accomasso, E. Vitale, C. Gallina, D. Roggio, S. Raimondo, R. Rastaldo, G. Alberto, F. Catalano and G. Martra,
et al., Silica Nanoparticles Actively Engage with Mesenchymal Stem Cells in Improving Cardiac Pro-Regenerative Functional Effects, Vasc. Pharmacol., 2018, 103–105, 66 CrossRef.
- C. Lu, X. Li, Y. Hu, R. G. Rowe and S. J. Weiss, MT1-MMP Controls Human Mesenchymal Stem Cell Trafficking and Differentiation, Cell, 2010, 115(2), 221–229 CAS.
- F. Mannello, G. A. M. Tonti, G. P. Bagnara and S. Papa, Role and Function of Matrix Metalloproteinases in the Differentiation and Biological Characterization of Mesenchymal Stem Cells, Stem Cells, 2006, 24(3), 475–481 CrossRef CAS PubMed.
- S. Kang, S. H. Bhang, S. Hwang, J. K. Yoon, J. Song, H. K. Jang, S. Kim and B. S. Kim, Mesenchymal Stem Cells Aggregate and Deliver Gold Nanoparticles to Tumors for Photothermal Therapy, ACS Nano, 2015, 9(10), 9678–9690 CrossRef CAS PubMed.
Footnote |
† Electronic supplementary information (ESI) available. See DOI: 10.1039/c8bm01267a |
|
This journal is © The Royal Society of Chemistry 2019 |
Click here to see how this site uses Cookies. View our privacy policy here.