DOI:
10.1039/C8SC03236J
(Edge Article)
Chem. Sci., 2018,
9, 7866-7873
Alternating oligo(o,p-phenylenes) via ruthenium catalyzed diol–diene benzannulation: orthogonality to cross-coupling enables de novo nanographene and PAH construction†
Received
21st July 2018
, Accepted 17th August 2018
First published on 30th August 2018
Abstract
Ruthenium(0) catalyzed diol–diene benzannulation is applied to the conversion of oligo(p-phenylene vinylenes) 2a–c, 5 and 6 to alternating oligo(o,p-phenylenes) 10a–c, 11–13. Orthogonality with respect to conventional palladium catalyzed biaryl cross-coupling permits construction of p-bromo-terminated alternating oligo(o,p-phenylenes) 10b, 11–13, which can be engaged in Suzuki cross-coupling and Scholl oxidation. In this way, structurally homogeneous nanographenes 16a–f are prepared. Nanographene 16a, which incorporates 14 fused benzene rings, was characterized by single crystal X-ray diffraction. In a similar fashion, p-bromo-terminated oligo(p-phenylene ethane diol) 9, which contains a 1,3,5-trisubstituted benzene core, is converted to the soluble, structurally homogeneous hexa-peri-hexabenzocoronene 18. A benzothiophene-terminated pentamer 10c was prepared and subjected to Scholl oxidation to furnish the helical bis(benzothiophene)-fused picene derivative 14. The steady-state absorption and emission properties of nanographenes 14, 16a,b,d,e,h and 18 were characterized. These studies illustrate how orthogonality of ruthenium(0) catalyzed diol–diene benzannulation with respect to classical biaryl cross-coupling streamlines oligophenylene and nanographene construction.
Introduction
Oligophenylenes comprise a wide-ranging and varied class of PAH compounds, which due to their tunable physical properties are promising candidates for organic photovoltaic materials.1 Additionally, Scholl oxidation2–4 of oligophenylenes and related structures enables access to structurally homogeneous nanographene materials.5,6 Despite the longstanding importance of oligophenylenes and nanographenes to the field of molecular electronics, their construction de novo remains challenging and relatively few methods for their synthesis are broadly applied. Strategies involving biaryl cross-coupling7 followed by Scholl oxidation2–4 or palladium catalyzed cyclo-dehydrohalogenations8–10 are among the most powerful. While many other benzannulation protocols have been reported,11 scalable, non-cryogenic catalytic methods that are orthogonal to biaryl cross-coupling would be especially valuable in terms of streamlining access to PAH chemical space.
Utilizing the concept of alcohol-mediated carbonyl addition,12 a ruthenium(0) catalyzed diene–diol [4 + 2] cycloaddition was recently developed in our laboratory (Scheme 1).12e,13a,b Aromatization of the cycloadducts occurs readily, enabling access to products of benzannulation from abundant diol and diene reactants.13c In an initial application of this method, a homologous series of rod-like triple-stranded phenylene cages was prepared.13d,14 This exercise suggested the feasibility of modular nanographene syntheses wherein diol–diene benzannulation is used to generate bromide-containing oligophenylenes amenable to late-stage diversification through metal catalyzed biaryl cross-coupling followed by Scholl oxidation. In fulfillment of this objective, we report syntheses of alternating oligo(o,p-phenylenes)15via ruthenium(0) catalyzed diol–diene benzannulation and, therefrom, structurally homogeneous nanographene materials containing as many as 22 aromatic rings.
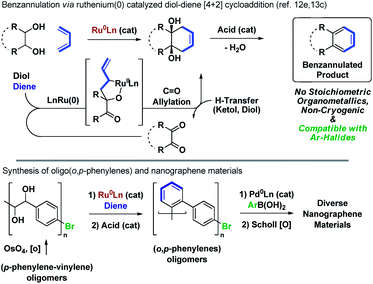 |
| Scheme 1 Alternating o,p-oligophenylenes and nanographenes via ruthenium catalyzed diol–diene benzannulation. | |
Research design and methods
Synthesis of oligo(o,p-phenylenes) and related PAH compounds
The synthesis of the requisite oligo(p-phenylene vinylene) diols 3a–3c is readily accomplished through Wittig olefination (Scheme 2).16,17 Thus, in close analogy to the literature procedure,17d terephthalaldehyde 1a was exposed to the indicated phosphonium salts in the presence of tBuOK to furnish the respective oligo(p-phenylene vinylenes) 2a–c in good yields. Alternate bases such as KOH, NaOH, NaOtBu, nBuLi and lithium diisopropylamide (LDA) led to incomplete conversion and the use of Horner–Wadsworth–Emmons (HWE) reagents was accompanied by substantial quantities of homo-coupling byproducts.18 Dihydroxylation of the oligo(p-phenylene vinylenes) 2a–c proved challenging due to competing oxidative cleavage to form aldehyde byproducts.19 Upjohn dihydroxylation conditions using N-methylmorpholine N-oxide (NMO)20 as the terminal oxidant attenuated this side reaction, delivering the oligo(p-phenylene vinylene) diols 3a–c in good to excellent yields. In a similar manner, a three-directional synthesis of tris-diol 9 was accomplished from benzene-1,3,5-tricarbaldehyde 1b (eqn (1)).13d | 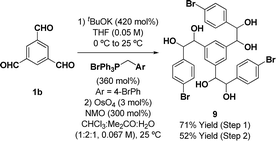 | (1) |
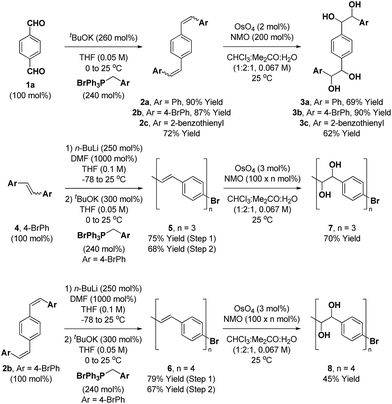 |
| Scheme 2 Synthesis of oligo(p-phenylene vinylene) diols 3a–3c, 7 and 8. a Yields are of material isolated by silica gel chromatography. See ESI† for further experimental details. | |
The synthesis of higher oligo(p-phenylene vinylene) diols 7 and 8 was accomplished in an iterative fashion through homologation of dibromo-styrene 4 and the 4-bromo-terminated oligo(p-phenylene vinylene) 2b (Scheme 2). Thus, lithiation of 4 and 2b followed by treatment with DMF provided the respective formyl derivatives,21 which upon Wittig olefination furnished the homologous 4-bromo-terminated oligo(p-phenylene vinylenes) 5 and 6. Exposure of the oligo(p-phenylene vinylenes) 5 and 6 to Upjohn dihydroxylation20 provided the oligo(p-phenylene vinylene) diols 7 and 8. To minimize competitive oxidative cleavage to form aldehydes observed in the formation of 8,19 a higher loading of OsO4 was required to shorten the reaction time. Additionally, for the synthesis of 7 and 8, use of the predominantly (Z)-selective Wittig olefination was important, as the less soluble products of (E)-selective HWE olefination were difficult to engage in dihydroxylation.
As the ruthenium(0) catalyzed [4 + 2] cycloaddition can be conducted from the ketol oxidation level,12e,13 routes involving benzoin condensation were explored. The crossed-benzoin condensation of terephthalaldehyde 1a with benzaldehyde occurred efficiently using an N-heterocyclic carbene (NHC) catalyst, providing ketol dehydro-3a in good yield (eqn (2)).22 However, these conditions were quite substrate dependent and attempted benzoin condensation of 4-bromo benzaldehyde and 2-benzothiophene carboxaldehyde was inefficient due to competing self-condensation.
| 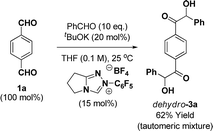 | (2) |
Benzannulation of oligo(p-phenylene vinylene) diols 3a–3c, 7–9 to form alternating oligo(o,p-phenylenes) 10a–c, 11–13 was next explored (Table 1). To our delight, the ruthenium(0) catalyzed cycloaddition of 1,3-butadiene with oligo(p-phenylene vinylene) diols 3a–3c, 7–9 proceeded smoothly in the presence of a carboxylic acid cocatalyst to furnish the corresponding cyclohexene diols in good to excellent yield.12e,13 Subsequent exposure of the cycloadducts to substoichiometric quantities of p-toluenesulfonic acid (p-TsOH) resulted in dehydration to form the alternating oligo(o,p-phenylenes) 10a–c, 11–13 in moderate to high yields.13c The dehydration reaction is highly temperature dependent and minor deviations from the optimal temperatures identified for each substrate caused a significant decrease in yield. Perhaps related to this observation, one-pot cycloaddition–dehydration, which was effective for the synthesis of fluoranthenes and acenes,13c was less efficient in the context of the present oligo(o,p-phenylene) syntheses.
Table 1 Ruthenium(0) catalyzed benzannulation of oligo(p-phenylene vinylene) diols 3a–3c, 7–9 to form oligo(o,p-phenylenes) 10a–c, 11–13a
Yields are of material isolated by silica gel chromatography.
Yield from ketol dehydro-3a. See ESI for further experimental details.
|
|
The alternating oligo(o,p-phenylenes) 10a–c, 11–13 prepared by our methods raise numerous possibilities for the synthesis of diverse PAH compounds, including helicenes, graphene nanodots and nanoribbons. To illustrate, the benzothiophene derived oligomer 10c was subjected to Scholl oxidation conditions employing anhydrous FeCl3 (ref. 3b) to form the S-doped helical picene derivative 14, which was characterized by single crystal X-ray diffraction (eqn (3)).23 The regioisomeric compound iso-14 was not observed. This result is consistent with the findings of Hilt and co-workers, who observe similar regioselectivities in related FeCl3-mediated Scholl oxidations of alternating oligo(o,p-phenylenes).24
| 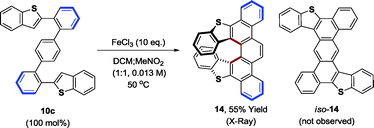 | (3) |
Access to bromo-terminated oligomers, such as 11, led us to explore modular nanographene syntheses wherein late-stage diversification through metal catalyzed biaryl cross-coupling is followed by Scholl oxidation (Schemes 3 and 4). Toward this end, heptaphenylene 11 was subjected to Suzuki cross-coupling conditions25 with aryl boronate or aryl boronic acid partners that were selected to facilitate Scholl oxidation and confer solubility to the resultant nanographenes (Scheme 3).26 Thus, heptaphenylene 11 was converted to the bis(2,4,6-trimethylphenyl) nonaphenylene 15a, which was exposed to DDQ and triflic acid.27 However, as confirmed by single crystal X-ray crystallography (Fig. 1), Scholl oxidation was accompanied by aryl and methyl migration to form 16a, which was highly soluble in chloroform. Skeletal rearrangement is often observed during Scholl oxidation and can be difficult to predict.3b,28 Alternate Scholl oxidation conditions resulted in diminished yields of 16a or produced complex mixtures of numerous products. The structure of nanographene 16a, which contains 14 fused aromatic rings, is nevertheless quite remarkable, as crystal structures of large planar PAH compounds remain quite uncommon.29,30 The supramolecular structure of nanographene 16a in the solid state is dominated by π–π stacking interactions, consistent with King's observation that large flanking groups on nanographenes disrupt the herringbone packing typically seen in crystal structures of PAH materials.26,31,32
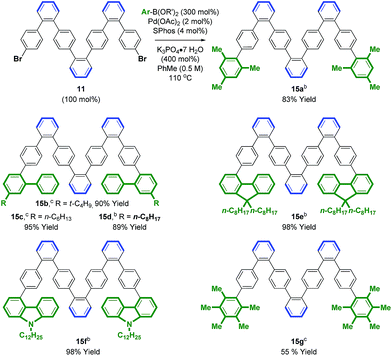 |
| Scheme 3 Palladium catalyzed cross-coupling of 11 to form oligophenylenes 15a–g. a Yields are of material isolated by silica gel chromatography. See ESI† for further experimental details. b Pinacol boronate. c Boronic acid. | |
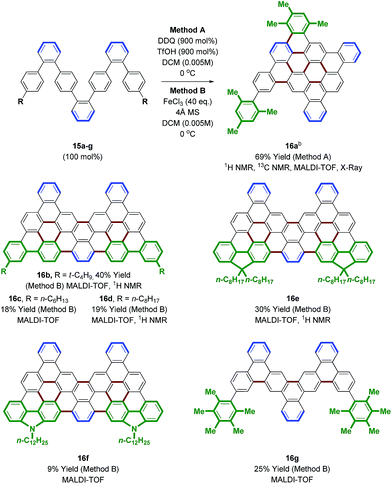 |
| Scheme 4 Scholl oxidation of oligophenylenes 15a–g to form nanographenes 16a–g. a Yields are of material isolated by silica gel chromatography or by trituration. See ESI† for further experimental details. b DDQ (600 mol%), TfOH (600 mol%). | |
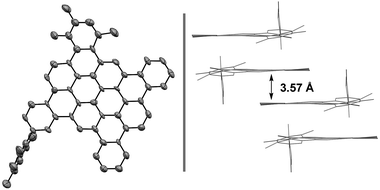 |
| Fig. 1 Single-crystal X-ray diffraction data of nanographene 16a. Displacement ellipsoids are scaled to 50% probability. Hydrogens have been omitted for clarity and packing in the solid state. See ESI† for further structural details. | |
An effort was made to design oligophenylenes that are less prone to skeletal rearrangement under Scholl oxidation conditions. It was recognized that Scholl oxidation to form triangular tribenzo[a,g,m]coronene motifs occurs in a highly efficient manner.33 Hence, Suzuki coupling was conducted with ortho-biarylboron reagents to form oligomers 15b–f (Scheme 3). Additionally, oligomer 15g, which incorporates pentamethylphenyl termini, was targeted, as methyl migration is not possible on the fully substituted aromatic ring. Indeed, Scholl oxidation using either DDQ and triflic acid27 or FeCl3 in the presence of molecular sieves34 gave 16b–g without rearrangement. Nanographenes 16b, 16d and 16e were sparingly soluble in chloroform and nanographenes 16c, 16f and 16g were highly insoluble. MALDI-TOF mass spectrometry was used to characterize all compounds, as well as 1H NMR for 16b, 16d and 16e. Finally, an alternate strategy for Scholl oxidation in the absence of skeletal rearrangement entailed conversion of heptaphenylene 11 to the bis(n-octyl ethyl) 15hvia copper catalyzed C–O bond formation.35 Scholl oxidation of bis(n-octyl ethyl) 15h under DDQ and triflic acid conditions27 gave the nanographene 16h in 65% yield (eqn (4)). This derivative was soluble enough to be characterized by 1H and 13C NMR, in addition to MALDI-TOF mass spectrometry.
| 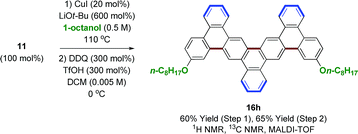 | (4) |
Hexa-peri-hexabenzocoronenes (HBCs) represent yet another class of fully benzenoid PAHs that have garnered interest as potential materials for opto-electronic devices.6 However, current methods available for HBC synthesis are limited. This is especially true for HBCs with low symmetry,36 electron deficient HBCs37 or those substituted at the bay region.38 The synthesis of the electron deficient D3h symmetric HBC 17, which incorporates bromo-substituents in the bay region, was achieved through Scholl oxidation of the branched heptaphenylene 13 under DDQ and triflic acid conditions (eqn (5)).27 Although the resulting HBC 17 is quite insoluble, Sonogashira coupling39 occurred in good yield to furnish the chloroform-soluble 18, which was characterized by 1H and 13C NMR and MALDI-TOF mass spectrometry.
| 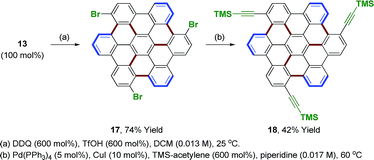 | (5) |
Spectroscopic analysis
The photophysical properties of a subset of the molecules presented herein that were sufficiently soluble in dichloromethane (14, 16a,b,d,e,h, 18) were characterized by steady-state absorption and fluorescence spectroscopies. Experimental details and spectra measured for 16a and 18 are included in the ESI.†Fig. 2a displays absorption and emission spectra of the helicene 14. This compound features a broad absorption profile that rises from ∼2.9 eV and contains a series of sharp resonances best explained by comparison with similar picene and helicene examples previously explored.40,41 In particular, the absorption spectrum can be qualitatively explained as a combination of low-lying higher helicene transitions40 and high-energy transitions associated with the fused thiophene rings contained in the picene backbone, as studied by Morin and coworkers.41b Likewise, the emission spectrum of 14 is consistent with other higher helicenes, particularly [7]-helicene, the first in the series for which ring overlap begins with an increasing number of rings.40e,g It is worth noting that helicenes, particularly higher helicenes containing thiophenes, have attracted interest as chiral nonlinear optical materials.42 To our knowledge, compound 14 is the first reported example of a picene-helicene hybrid and we believe its chiroptical properties will be of future interest.
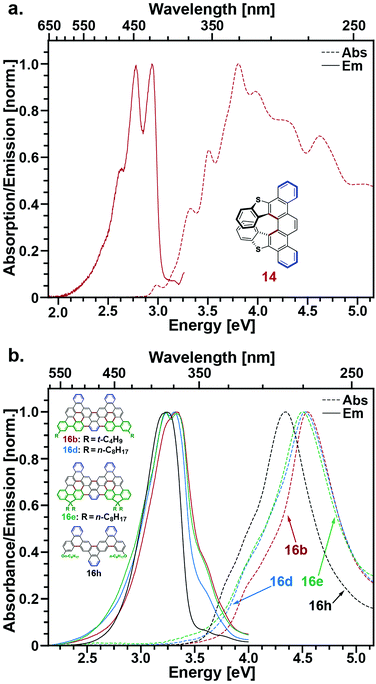 |
| Fig. 2 (a) Absorption (dashed) and emission (solid) of 14. (b) Absorption (dashed) and emission (solid) of 16b (red), 16d (blue), 16e (green), and 16h (black). | |
Fig. 2b displays absorption and emission spectra of the nanographenes 16b,d,e, and h. Although these compounds feature the largest extended π-conjugated systems among the compounds we have characterized spectroscopically, their absorption and emission spectra peak at higher energies than compounds 14, 16a, and 18. This is best explained by the sole presence of arm-chair edges along the periphery of these materials, which are thought to bestow nanographenes with larger bandgaps.43 Interestingly, reducing the number of rings along the nanographene short axis, as is done for 16h, acts to reduce its optical bandgap relative to 16b, 16d, and 16e even though this also reduces the size of its π-system.
Conclusions
In summary, we report the synthesis of oligophenylenes and various PAH materials constructed though the use of Ru(0)-catalyzed diol–diene cycloaddition coupling.4 Oligo-1,2-diols were constructed via iterative Wittig coupling and dihydroxylation. Furthermore, orthogonality to Pd-catalyzed cross coupling allows for bromo-terminated polyphenylenes that could be functionalized to provide various nanographenes 16a–h after Scholl oxidation. Additionally, Scholl oxidation of 10c and 13 provided benzothiophene helical picene 14 and hexa-peri-hexabenzocoronene 18, respectively. Thus, we have demonstrated the use of Ru(0) catalyzed diol–diene benzannulation in the fabrication of three distinct types of PAH materials. Photophysical analysis of 14, 16a,b,d,e,h and 18 demonstrated that nanographenes prepared by these synthetic routes can display highly variable optical properties, which make these methods useful for the preparation of organic electronic materials. Future studies will focus on the development of related methods for alcohol-mediated benzannulation and their application to PAH construction, including the use of symmetric 2,3-diaryl-substituted butadiene building blocks.
Conflicts of interest
There are no conflicts to declare.
Acknowledgements
The Robert A. Welch Foundation (F-0038 and F-1885), the NSF (CHE-1565688), the NIH (1 S10 OD021508-01), the Japan Student Services Organization (HS, YM), the China Scholarship Council (JN) and the American Chemical Society DOC Graduate Fellowship (ZAK) are acknowledged for partial financial support.
References
- For selected reviews on the synthesis and materials chemistry of oligophenylenes, see:
(a) J. M. Tour, Adv. Mater., 1994, 6, 190 CrossRef;
(b) A. J. Berresheim, M. Müller and K. Müllen, Chem. Rev., 1999, 99, 1747 CrossRef PubMed;
(c) B. D. Steinberg and L. T. Scott, Angew. Chem., Int. Ed., 2009, 48, 5400 CrossRef PubMed;
(d) C. Li, M. Liu, N. G. Pschirer, M. Baumgarten and K. Müllen, Chem. Rev., 2010, 110, 6817 CrossRef PubMed;
(e) R. Jasti and C. R. Bertozzi, Chem. Phys. Lett., 2010, 494, 1 CrossRef PubMed;
(f) O. S. Wenger, Chem. Soc. Rev., 2011, 40, 3538 RSC;
(g) H. Omachi, Y. Segawa and K. Itami, Acc. Chem. Res., 2012, 45, 1378 CrossRef PubMed;
(h) D. E. Gross, L. Zang and J. S. Moore, Pure Appl. Chem., 2012, 84, 869 Search PubMed;
(i) X. Guo, M. Baumgarten and K. Müllen, Prog. Polym. Sci., 2013, 38, 1832 CrossRef;
(j) M. R. Golder and R. Jasti, Acc. Chem. Res., 2015, 48, 557 CrossRef PubMed;
(k) B. A. G. Hammer and K. Müllen, Chem. Rev., 2016, 116, 2103 CrossRef PubMed;
(l) C. S. Hartley, Acc. Chem. Res., 2016, 49, 646 CrossRef PubMed;
(m) Y. Segawa, A. Yagi, K. Matsui and K. Itami, Angew. Chem., Int. Ed., 2016, 55, 5136 CrossRef PubMed;
(n) Y. Segawa, H. Ito and K. Itami, Nat. Rev. Mater., 2016, 1, 15002 CrossRef.
- For the seminal reports of the Scholl oxidation, see:
(a) R. Scholl and J. Mansfeld, Chem. Ber., 1910, 43, 1734 CrossRef;
(b) R. Scholl, C. Seer and R. Weitzenbök, Chem. Ber., 1910, 43, 2202 CrossRef.
- For selected reviews on the Scholl oxidation, see:
(a) P. Kovacic and M. B. Jones, Chem. Rev., 1987, 87, 357 CrossRef;
(b) B. T. King, J. Kroulík, C. R. Robertson, P. Rempala, C. L. Hilton, J. D. Korinek and L. M. Gortari, J. Org. Chem., 2007, 72, 2279 CrossRef PubMed;
(c) M. Grzybowski, K. Skonieczny, H. Butenschön and D. T. Gryko, Angew. Chem., Int. Ed., 2013, 52, 9900 CrossRef PubMed.
- For a mechanistic study on the Scholl oxidation, see: L. Zhai, R. Shukla, S. H. Wadumethrige and R. Rathore, J. Org. Chem., 2010, 75, 4748 CrossRef PubMed.
- For selected reviews on bottom up approaches to nanographene materials, see:
(a) L. Chen, Y. Hernandez, X. Feng and K. Müllen, Angew. Chem., Int. Ed., 2012, 51, 7640 CrossRef PubMed;
(b) U. H. F. Bunz, S. Menning and N. Martín, Angew. Chem., Int. Ed., 2012, 51, 7094 CrossRef PubMed;
(c) K. Itami, Pure Appl. Chem., 2012, 84, 907 Search PubMed;
(d) A. Narita, X.-Y. Wang, X. Feng and K. Müllen, Chem. Soc. Rev., 2015, 44, 6616 RSC;
(e) M. Stepien, E. Gonka, M. Zyla and N. Sprutta, Chem. Rev., 2017, 117, 3479 CrossRef PubMed.
- For selected reviews on molecular electronics applications of graphene materials, see:
(a) J. Wu, W. Pisula and K. Müllen, Chem. Rev., 2007, 107, 718 CrossRef PubMed;
(b) S. Dutta and S. K. Pati, J. Mater. Chem., 2010, 20, 8207 RSC;
(c) N. O. Weiss, H. Zhou, L. Liao, Y. Liu, S. Jiang, Y. Huang and X. Duan, Adv. Mater., 2012, 24, 5782 CrossRef PubMed;
(d) A. Ambrosi, C. K. Chua, A. Bonanni and M. Pumera, Chem. Rev., 2014, 114, 7150 CrossRef PubMed;
(e) N. Gao and X. Fang, Chem. Rev., 2015, 115, 8294 CrossRef PubMed;
(f) N. Zhang, M.-Q. Yang, S. Liu, Y. Sun and Y.-J. Xu, Chem. Rev., 2015, 115, 10307 CrossRef PubMed;
(g) J. Duan, S. Chen, M. Jaroniec and S. Z. Qiao, ACS Catal., 2015, 5, 5207 CrossRef.
- For selected reviews on biaryl cross-coupling for PAH construction, see:
(a) J. Hassan, M. Sévignon, C. Gozzi, E. Schulz and M. Lemaire, Chem. Rev., 2002, 102, 1359 CrossRef PubMed;
(b) M. Iyoda, Adv. Synth. Catal., 2009, 351, 984 CrossRef.
- For selected reviews on palladium catalyzed cyclo-dehydrohalogenations for PAH construction, see:
(a) S. Pascual, P. de Mendoza and A. M. Echavarren, Org. Biomol. Chem., 2007, 5, 2727 RSC;
(b) T. Jin, J. Zhao, N. Asao and Y. Yamamoto, Chem.–Eur. J., 2014, 20, 3554 CrossRef PubMed.
- For selected examples of palladium-catalyzed cyclo-dehydrohalogenations, see:
(a) D. E. Ames and A. Opalko, Tetrahedron, 1984, 40, 1919 CrossRef;
(b) J. E. Rice and Z.-W. Cai, Tetrahedron Lett., 1992, 33, 1675 CrossRef;
(c) J. J. González, N. García, B. Gómez-Lor and A. M. Echavarren, J. Org. Chem., 1997, 62, 1286 CrossRef;
(d) H. A. Wegner, L. T. Scott and A. J. de Meijere, Org. Chem., 2003, 68, 883 CrossRef PubMed;
(e) J. M. Quimby and L. T. Scott, Adv. Synth. Catal., 2009, 351, 1009 CrossRef.
- For a related one-step annulative dimerization, see: Y. Koga, T. Kaneda, Y. Saito, K. Murakami and K. Itami, Science, 2018, 359, 435 CrossRef PubMed.
- For selected reviews on benzannulation and the synthesis of polycyclic aromatic hydrocarbons (PAHs), see:
(a) L. T. Scott, Pure Appl. Chem., 1996, 68, 291 Search PubMed;
(b) S. Kotha, S. Misra and S. Halder, Tetrahedron, 2008, 64, 10775 CrossRef;
(c) H. Qu and C. Chi, Curr. Org. Chem., 2010, 14, 2070 CrossRef;
(d) D. Wu, H. Ge, S. H. Liu and J. Yin, RSC Adv., 2013, 3, 22727 RSC;
(e) J. Li and Q. Zhang, Synlett, 2013, 24, 686 CrossRef;
(f) D. Pérez, D. Peña and E. Guitián, Eur. J. Org. Chem., 2013, 5981 CrossRef;
(g) S. J. Hein, D. Lehnherr, H. Arslan, F. J. Uribe-Romo and W. R. Dichtel, Acc. Chem. Res., 2017, 50, 2776 CrossRef PubMed;
(h) W. Yang and W. A. Chalifoux, Synlett, 2017, 28, 625 CrossRef and ref. 3c..
- For selected reviews on alcohol-mediated carbonyl addition, see:
(a) J. M. Ketcham, I. Shin, T. P. Montgomery and M. J. Krische, Angew. Chem., Int. Ed., 2014, 53, 9142 CrossRef PubMed;
(b) F. Perez, S. Oda, L. M. Geary and M. J. Krische, Top. Curr. Chem., 2016, 374, 365 Search PubMed;
(c) K. D. Nguyen, B. Y. Park, T. Luong, H. Sato, V. J. Garza and M. J. Krische, Science, 2016, 354, 300 CrossRef PubMed;
(d) S. W. Kim, W. Zhang and M. J. Krische, Acc. Chem. Res., 2017, 50, 2371 CrossRef PubMed;
(e) H. Sato, B. W. H. Turnbull, K. Fukaya and M. J. Krische, Angew. Chem., Int. Ed., 2018, 57, 3012 CrossRef PubMed.
-
(a) L. M. Geary, B. W. Glasspoole, M. M. Kim and M. J. Krische, J. Am. Chem. Soc., 2013, 135, 3796 CrossRef PubMed;
(b) Z. A. Kasun, L. M. Geary and M. J. Krische, Chem. Commun., 2014, 7545 RSC;
(c) L. M. Geary, T.-Y. Chen, T. P. Montgomery and M. J. Krische, J. Am. Chem. Soc., 2014, 136, 5920 CrossRef PubMed;
(d) H. Sato, J. A. Bender, S. T. Roberts and M. J. Krische, J. Am. Chem. Soc., 2018, 140, 2455 CrossRef PubMed.
- Only two other all-benzene phenylene cages have been reported, which are of spherical topology:
(a) K. Matsui, Y. Segawa, T. Namikawa, K. Kamada and K. Itami, Chem. Sci., 2013, 4, 84 RSC;
(b) E. Kayahara, T. Iwamoto, H. Takaya, T. Suzuki, M. Fujitsuka, T. Majifma, N. Yasuda, N. Matsuyama, S. Seki and S. Yamago, Nat. Commun., 2013, 4, 2694 CrossRef PubMed;
(c) K. Matsui, Y. Segawa and K. Itami, J. Am. Chem. Soc., 2014, 136, 16452 CrossRef PubMed.
- For selected examples of related oligo(o-phenylenes), see:
(a) J. He, J. L. Crase, S. H. Wadumethrige, K. Thakur, L. Dai, S. Zou, R. Rathore and C. S. Hartley, J. Am. Chem. Soc., 2010, 132, 13848 CrossRef PubMed;
(b) S. Mathew, J. T. Engle, C. J. Ziegler and C. S. Hartley, J. Am. Chem. Soc., 2013, 135, 6714 CrossRef PubMed;
(c) S. Mathew, L. A. Crandall, C. J. Ziegler and C. S. Hartley, J. Am. Chem. Soc., 2014, 136, 16666 CrossRef PubMed and ref. 1l..
- For a review encompassing the synthesis of poly(p-phenylene vinylene) via Wittig olefination, see: A. J. Blayney, I. F. Perepichka, F. Wudl and D. F. Perepichka, Isr. J. Chem., 2014, 54, 674 CrossRef.
-
(a) U. Schöllkopf, Angew. Chem., 1959, 71, 260 CrossRef;
(b) R. N. McDonald and T. W. Campbell, J. Am. Chem. Soc., 1960, 82, 4669 CrossRef;
(c) D. Tanner, O. Wennerström and U. Norinder, Tetrahedron, 1986, 42, 4499 CrossRef;
(d) J. Heinze, J. Mortensen, K. Müllen and R. Schenk, J. Chem. Soc., Chem. Commun., 1987, 701 RSC;
(e) R. E. Gill, A. Meetsma and G. Hadziioannou, Adv. Mater., 1996, 8, 212 CrossRef.
- J. N. Ngwendson, C. M. Schultze, J. W. Bollinger and A. Banerjee, Can. J. Chem., 2008, 86, 668 CrossRef.
- C. Döbler, G. M. Mehltretter, U. Sundermeier and M. Beller, J. Organomet. Chem., 2001, 621, 70 CrossRef.
-
(a) V. VanRheenen, R. C. Kelly and D. Y. Cha, Tetrahedron Lett., 1976, 17, 1973 CrossRef;
(b) P. Dupau, R. Epple, A. A. Thomas, V. V. Fokin and K. B. Sharpless, Adv. Synth. Catal., 2002, 344, 421 CrossRef.
- T. Bosanac and C. S. Wilcox, Org. Lett., 2004, 6, 2321 CrossRef PubMed.
- D. Enders and U. Kallfass, Angew. Chem., Int. Ed., 2002, 41, 1743 CrossRef PubMed.
- For selected reviews on organic materials with benzothiophene cores, see:
(a) J. E. Anthony, Chem. Rev., 2006, 106, 5028 CrossRef PubMed;
(b) Y. Shen and C.-F. Chen, Chem. Rev., 2012, 112, 1463 CrossRef PubMed;
(c) W. Jiang, Y. Li and Z. Wang, Chem. Soc. Rev., 2013, 42, 6113 RSC;
(d) K. Takimiya, I. Osaka, T. Mori and M. Nakano, Acc. Chem. Res., 2014, 47, 1493 CrossRef PubMed;
(e)
E. Licandro, S. Cauteruccio and D. Dova, in Advanced in Heterocyclic Chemistry: Thiahelicenes: From Basic Knowledge to Applications, ed. E. F. V. Scriven and C. A. Ramsden, Elsevier, New York, 2016, vol. 118, pp. 1–46 Search PubMed;
(f) H. Yao, L. Ye, H. Zhang, S. Li, S. Zhang and J. Hou, Chem. Rev., 2016, 116, 7397 CrossRef PubMed.
- M. Danz, R. Tonner and G. Hilt, Chem. Commun., 2012, 48, 377 RSC.
- S. D. Walker, T. E. Barder, J. R. Martinelli and S. L. Buchwald, Angew. Chem., Int. Ed., 2004, 43, 1871 CrossRef PubMed.
- For the effect of non-planarity and tert-butyl groups on PAH solubility, see: B. Kumar, C. E. Strasser and B. T. King, J. Org. Chem., 2012, 77, 311 CrossRef PubMed.
-
(a) D. J. Jones, B. Purushothaman, S. Ji, A. B. Holmes and W. W. H. Wong, Chem. Commun., 2012, 48, 8066 RSC;
(b) M. R. Ajayakumar, Y. Fu, F. Hennersdorf, H. Komber, J. J. Weigand, A. Alfonsov, A. A. Popov, R. Berger, J. Liu and K. Müllen, J. Am. Chem. Soc., 2018, 140, 6240 CrossRef PubMed.
- For examples of skeletal rearrangement in Scholl oxidation, see:
(a) F. Dötz, J. D. Brand, S. Ito, L. Gherghel and K. Müllen, J. Am. Chem. Soc., 2000, 122, 7707 CrossRef;
(b) X. Dou, X. Yang, G. J. Bodwell, M. Wagner, V. Enkelmann and K. Müllen, Org. Lett., 2007, 9, 2485 CrossRef PubMed;
(c) A. Ajaz, E. C. McLaughlin, S. L. Skraba, R. Thamatam and R. P. Johnson, J. Org. Chem., 2012, 77, 9487 CrossRef PubMed;
(d) A. Pradhan, P. Dechambenoit, H. Bock and F. Durola, J. Org. Chem., 2013, 78, 2266 CrossRef PubMed;
(e) J. Liu, A. Narita, S. Osella, W. Zhang, D. Schollmeyer, D. Beljonne, X. Feng and K. Müllen, J. Am. Chem. Soc., 2016, 138, 2602 CrossRef PubMed.
- K. Kawasumi, Q. Zhang, Y. Segawa, L. T. Scott and K. Itami, Nat. Chem., 2013, 5, 739 CrossRef PubMed : “To date, the 26-ring nanographene…is the largest PAH…to have been characterized by X-ray crystallography”..
- For recent examples of large PAH crystal structures, see:
(a) S. Seifart, K. Shoyama, D. Schmidt and F. Würthner, Angew. Chem., Int. Ed., 2016, 55, 6390 CrossRef PubMed;
(b) K. Y. Cheung, C. K. Chan, Z. Liu and Q. Miao, Angew. Chem., Int. Ed., 2017, 56, 9003 CrossRef PubMed;
(c) V. Berezhnaia, M. Roy, N. Vanthuyne, M. Villa, J.-V. Naubron, J. Rodriguez, Y. Coquerel and M. Gingras, J. Am. Chem. Soc., 2017, 139, 18508 CrossRef PubMed;
(d) S. H. Pun, C. K. Chan, J. Luo, Z. Liu and Q. Miao, Angew. Chem., Int. Ed., 2018, 57, 1581 CrossRef PubMed.
- For a review of aromatic interactions, see: C. A. Hunter, K. R. Lawson, J. Perkins and C. J. Urch, J. Chem. Soc., Perkin Trans. 2, 2001, 651 RSC.
- For analysis on crystal packing of PAH materials, see:
(a) A. Gavezzotti and G. R. Resiraju, Acta Crystallogr., 1988, B44, 427 CrossRef;
(b) A. Gavezzotti, Chem. Phys. Lett., 1989, 161, 67 CrossRef;
(c) A. Guijarro, J. A. Vergés, E. San-Fabián, G. Chiappe and E. Louis, ChemPhysChem, 2016, 17, 3548 CrossRef PubMed.
- Z. Li, L. Zhi, N. T. Lucas and Z. Wang, Tetrahedron, 2009, 65, 3417 CrossRef.
- In Scholl oxidations using MoCl5, molecular sieves were shown to sequester HCl and increase product yields: B. Kramer, R. Fröhlich and S. R. Waldvogel, Eur. J. Org. Chem., 2003, 3549 CrossRef.
- J. Huang, Y. Chen, J. Chan, M. L. Ronk, R. D. Larsen and M. M. Faul, Synlett, 2011, 1419 CrossRef.
-
(a) J. Wu, M. D. Watson and K. Müllen, Angew. Chem., Int. Ed., 2003, 42, 5329 CrossRef PubMed;
(b) J. Wu, M. Baumgarten, M. G. Debije, J. M. Warman and K. Müllen, Angew. Chem., Int. Ed., 2004, 43, 5331 CrossRef PubMed;
(c) X. Feng, J. Wu, V. Enkelmann and K. Müllen, Org. Lett., 2006, 8, 1145 CrossRef PubMed.
- Attempted Scholl oxidation to form halogen-substituted HBCs results in incomplete conversion: J. Wu, M. D. Watson, L. Zhang, Z. Wang and K. Müllen, J. Am. Chem. Soc., 2004, 126, 177 CrossRef PubMed and ref. 27..
- Attempted Scholl oxidation to form HBC that are substituted in the bay region results in skeletal rearrangement or incomplete oxidation: A. Pradhan, P. Dechambenoit, H. Bock and F. Durola, Angew. Chem., Int. Ed., 2011, 50, 12582 CrossRef PubMed and ref. 28c and d..
- For selected reviews on Sonogashira coupling, see:
(a) R. Chinchilla and C. Nájera, Chem. Soc. Rev., 2011, 40, 5084 RSC;
(b) M. Schilz and H. Plenio, J. Org. Chem., 2012, 77, 2798 CrossRef PubMed.
- For higher and thiolated helicenes, see:
(a) W. S. Brickell, A. Brown, C. M. Kemp and S. F. Mason, J. Chem. Soc. A, 1971, 756 RSC;
(b) M. B. Groen and H. Wynberg, J. Am. Chem. Soc., 1971, 93, 2968 CrossRef;
(c) J. H. Dopper, D. Oudman and H. Wynberg, J. Am. Chem. Soc., 1973, 95, 3692 CrossRef;
(d) S. Odenland and W. Schmidt, J. Am. Chem. Soc., 1975, 97, 6633 CrossRef;
(e) J. B. Birks, D. J. S. Birch, E. Cordemans and E. vander Donckt, Chem. Phys. Lett., 1976, 43, 33 CrossRef;
(f) F. Furche, R. Ahlrichs, C. Wachsmann, E. Weber, A. Sobanski, F. Vögtle and S. Grimme, J. Am. Chem. Soc., 2000, 122, 1717 CrossRef;
(g) T. Caronna, R. Sinisi, M. Catellani, S. Luzzati, S. Abbate and G. Longhi, Synth. Met., 2001, 119, 79 CrossRef;
(h) Y. Kitahara and K. Tanaka, Chem. Commun., 2002, 932 RSC;
(i) K. Tanaka, H. Osuga and Y. Kitahara, J. Org. Chem., 2002, 67, 1795 CrossRef PubMed;
(j) M. Miyasaka, A. Rajca, M. Pink and S. Rajca, J. Am. Chem. Soc., 2005, 127, 13806 CrossRef PubMed;
(k) L. Rulísek, O. Exner, L. Cwiklik, P. Jungwirth, I. Stary, L. Popísil and Z. Havlas, J. Phys. Chem. C, 2007, 111, 14948 CrossRef;
(l) Y.-H. Tian, G. Park and M. Kertesz, Chem. Mater., 2008, 20, 3266 CrossRef;
(m) A. Rajca, M. Pink, S. Xiao, M. Miyasaka, S. Rajca, K. Das and K. Plessel, J. Org. Chem., 2009, 74, 7504 CrossRef PubMed;
(n) C. Kim, T. J. Marks, A. Facchetti, M. Schiavo, A. Bossi, S. Maiorana, E. Licandro, F. Todescato, S. Toffanin, M. Muccini, C. Graiff and A. Tiripicchio, Org. Electron., 2009, 10, 1511 CrossRef;
(o) A. Rajca, M. Miyasaka, S. Xiao, P. J. Boratynski, M. Pink and S. Rajca, J. Org. Chem., 2009, 74, 9105 CrossRef PubMed;
(p) Y. Nakai, T. Mori and Y. Inoue, J. Phys. Chem. A, 2012, 116, 7372 CrossRef PubMed;
(q) D. Waghray, A. Cloet, K. Van Hecke, S. F. L. Mertens, S. De Feyter, L. Van Meervelt, M. Van der Auweraer and W. Dehaen, Chem.–Eur. J., 2013, 19, 12077 CrossRef PubMed;
(r) F. Chen, T. Tanaka, Y. S. Hong, T. Mori, D. Kim and A. Osuka, Angew. Chem., Int. Ed., 2017, 56, 14688 CrossRef PubMed;
(s) K. Uematsu, K. Noguchi and K. Nakano, Phys. Chem. Chem. Phys., 2018, 20, 3286 RSC.
-
(a) Y. Nishihara, M. Kinoshita, K. Hyodo, Y. Okuda, R. Eguchi, H. Goto, S. Hamao, Y. Takabayashi and Y. Kubozono, RSC Adv., 2013, 3, 19341 RSC;
(b) D. Miao, M. Daigle, A. Lucotti, J. Boismenu-Lavoie, M. Tommasini and J.-F. Morin, Angew. Chem., Int. Ed., 2018, 57, 3588 CrossRef PubMed.
- For helicene nonlinearity, see:
(a) K. Clays, K. Wostyn, A. Persoons, S. Maiorana, A. Papagni, C. A. Daul and V. Weber, Chem. Phys. Lett., 2003, 372, 438 CrossRef;
(b) E. Botek, J.-M. André, B. Champagne, T. Verbiest and A. Persoons, J. Chem. Phys., 2005, 122, 234713 CrossRef PubMed;
(c) E. Botek, M. Spassova, B. Champagne, I. Asselberghs, A. Persoons and K. Clays, Chem. Phys. Lett., 2005, 412, 274 CrossRef;
(d) B. Jansik, A. Rizzo, H. Ågren and B. Champagne, J. Chem. Theory Comput., 2008, 4, 457 CrossRef PubMed;
(e) A. Bossi, E. Licandro, S. Maiorana, C. Rigamonti, S. Righetto, G. R. Stephenson, M. Spassova, E. Botek and B. Champagne, J. Phys. Chem. C, 2008, 112, 7900 CrossRef;
(f) B. Champagne and S. N. Labidi, Chem. Phys. Lett., 2016, 644, 195 CrossRef.
-
(a) J.-I. Aihara, J. Phys. Chem. A, 1999, 103, 7487 CrossRef;
(b) R. Ruiterkamp, T. Halasinski, F. Salama, B. H. Foing, L. J. Allamandola, W. Schmidt and P. Ehrenfreund, Astron. Astrophys., 2002, 390, 1153 CrossRef;
(c) G. Malloci, G. Mulas and C. Joblin, Astron. Astrophys., 2004, 426, 105 CrossRef;
(d) Y. Ruiz-Morales, J. Phys. Chem. A, 2004, 108, 10873 CrossRef;
(e) L. R. Radovic and B. Bockrath, J. Am. Chem. Soc., 2005, 127, 5917 CrossRef PubMed;
(f) M. Kastler, J. Schmidt, W. Pisula, D. Sebastiani and K. Müllen, J. Am. Chem. Soc., 2006, 128, 9526 CrossRef PubMed;
(g) X. Feng, W. Pisula and K. Müllen, Pure Appl. Chem., 2009, 81, 2203 Search PubMed;
(h) F. Cataldo, O. Ursini, G. Angelini and S. Iglesias-Groth, Fullerenes, Nanotubes, Carbon Nanostruct., 2011, 19, 712 Search PubMed;
(i) X.-L. Fan, X.-Q. Wang, J.-T. Wang and H.-D. Li, Phys. Lett. A, 2014, 378, 1379 CrossRef;
(j) M. A. Sk, A. Ananthanarayanan, L. Huang, K. H. Lim and P. Chen, J. Mater. Chem. C, 2014, 2, 6954 RSC;
(k) T. Hayakawa, H. Song, Y. Ishii and S. Kawasaki, AIP Conf. Proc., 2016, 1733, 020007 CrossRef.
Footnote |
† Electronic supplementary information (ESI) available: Experimental procedures and spectroscopic data for all new compounds (1H NMR, 13C NMR, IR, HRMS), including images of NMR spectra. Single crystal X-ray diffraction data for compounds 11 (1856649), 14 (1856650) and 16a (1856651). For ESI and crystallographic data in CIF or other electronic format see DOI: 10.1039/c8sc03236j |
|
This journal is © The Royal Society of Chemistry 2018 |