DOI:
10.1039/C8RA09291E
(Paper)
RSC Adv., 2018,
8, 41938-41949
Biguanidine functional chitooligosaccharide modified reverse osmosis membrane with improved anti-biofouling property
Received
10th November 2018
, Accepted 28th November 2018
First published on 17th December 2018
Abstract
In this work, a novel anti-biofouling reverse osmosis (RO) membrane was fabricated by second interfacial polymerization of synthesized cationic biguanidine functional chitooligosaccharide (COSG) with the acyl chloride groups on the nascent RO membrane surface. COSG fully combined the bioactivity of oligosaccharides and guanidine-based polymers. Meanwhile, the chitooligosaccharide (COS)-modified RO membranes were prepared for comparison. Both the COS and COSG modified membrane surface negative charge and roughness were reduced, and the hydrophilicity was significantly improved. The water flux of the COSG-modified membrane increased 16.61% than that of the virgin membrane, while the salt rejection exhibited slight decrease. Membrane fouling tests of lysozyme and bovine serum albumin illustrated that the COSG-modified membrane exhibited improved anti-fouling property with low flux decline ratios and high flux recovery ratios. Besides, the COSG-modified membranes exhibited excellent anti-bacterial property with mortalities of 99.9% against both Escherichia coli and Bacillus subtilis. Moreover, the COSG-modified membrane maintained high desalination performance after being immersed in the bacteria suspensions for seven days.
1. Introduction
As an economical separation technology, reverse osmosis (RO) membrane technology has been used in many fields, and will achieve greater success in ecological restoration and environmental management.1–4 However, the membrane fouling has been identified as a challenge which restricts the further development and application of RO membrane technology.5 The fouling layer can cause several adverse impacts including higher operating pressure, lower membrane performance, increased energy consumption and shortened membrane life span.6,7
Although both the pretreatment of feed solution and membrane cleaning can effectively alleviate membrane fouling, bacteria cannot be fully removed. Once the residual bacteria are adsorbed on the membrane surface, they begin to colonize and produce extracellular polymeric substances (EPSs) in situ and ultimately form a mature biofilm, which makes the biofouling more complex and difficult to control. Many biocides (such as chlorine, ozone) are often applied in the pretreatment process to control biofouling.8 However, the active chlorine as an oxidizing agent has adverse effects on the performance of aromatic polyamide RO membranes.9 The dosing of biocides in RO processes maybe also toxic to human, and couldn't be used for potable water production.10 Thus, it is urgently needed to develop anti-biofouling RO membranes in practical applications.
Currently, many anti-biofouling membranes have been prepared via surface modification.7,11–16 The methods of surface modification include physical coating or deposition, layer by layer self-assembly, free radical initiated polymerization and so on. Among those methods, the second interfacial polymerization (SIP) which utilizes the unreacted active groups on the membrane surface is not only more stable than physical methods but also easier and more effective than most of chemical binding methods.7,14,15,17 The anti-biofouling strategies usually focus on improving the anti-adhesive and/or antimicrobial properties of membranes. The surface properties (such as hydrophilicity, roughness, charge) are supposed as essential factors to control membrane biofouling.5,7,18–20 Accordingly, many hydrophilic materials (such as poly(ethylene glycol), polyvinyl alcohol, zwitterionic polymers) have been immobilized on the membrane to mitigate biofouling by reducing the interaction between biofoulants and the membrane surface, and suppressing the initial microbial adhesion.15,16,21–23 However, they are ineffective to restrain microbial colonization once a small amount of bacteria attached onto the membrane surface. Many biocides which involve metallic nanoparticles (such as Ag, Cu, ZnO NPs), antibiotics, quaternary ammonium salts, carbon-based materials (carbon nanotubes, graphene oxide) and N-halamines have also been incorporated onto the membrane surface to kill the bacteria effectively and reduce the rate of biofilm formation.12,14,24–27 Nevertheless, the bactericidal surface may make microorganisms attach and colonize on the membrane surface more easily due to the rapid accumulation of dead microbial cells.28 Hence, it is more efficient to fabricate anti-biofouling membranes with both anti-adhesive and anti-microbial features.13,29,30
As a kind of cationic anti-bacterial agents, biguanides have the broad-spectrum antibacterial property with low mammalian toxicity and have long been applied as medical and food protection agents, antiseptics for industry products and other commodities.31–36 The positive charged biguanide groups of biguanides have strong electrostatic interaction with the negative charges of bacterial surface, causing the bacterial cell membrane dissolution and finally the cell death.37 Recently, polymeric biguanides have been used to prepare anti-bacterial membranes.38–41 However, the desalination performance of the modified membrane was greatly impaired and the modification process was complex and time-consuming.
In order to improve membrane anti-bacterial property while maintain high membrane flux, we chose a hydrophilic chitooligosaccharide (COS) to optimize the surface characteristics. COS is a degraded product of chitin or chitosan. It has several advantages for surface modification due to resource-rich, low cost, water solubility, stability, cytocompatibility and various biological activities.42,43 Biological activities of COS include anti-bacterial, anti-fungal, anti-viral, anti-tumor, anti-oxidant, immunoregulatory, exert fat and blood pressure control effects.44,45 Meanwhile, according to molecular dynamics results, this hydrophilic modification may increase the free volume of the membrane as it swells when hydrated and vibrates due to molecular collisions, thereby increasing the water flux of the membrane.46–50 Moreover, COS is rich in amine groups, which could be easily functionalized and immobilized onto the nascent RO membrane surface.14,29,51,52
In this paper, to simultaneously improve the water flux and anti-biofouling performance of RO membrane, we prepared a biguanidine functional chitooligosaccharide (COSG) and grafted the COSG on the nascent RO membrane by second interfacial polymerization. The biguanide groups of COSG could increase the cationic density and improve the anti-bacterial performance of COS significantly.53 The prepared membranes were analyzed by X-ray photoelectron spectroscopy (XPS), scanning electron microscopy (SEM), atomic force microscopy (AFM), contact angle goniometer and electrokinetic analyzer. Membrane desalination performance was experimentally evaluated. Bovine serum albumin (BSA) and lysozyme were chosen to evaluate the anti-fouling properties of membranes. Escherichia coli (E. coli) and Bacillus subtilis (B. subtilis) were chosen as typical stains to investigate the anti-bacterial and anti-biofouling properties of membranes.
2. Experimental
2.1. Materials
The polysulfone (PSf) ultrafiltration membrane with molecular weight cut off (MWCO) of 30
000 dalton was used as supports and purchased from Pureach Corporation (China). (+)-10-camphorsulfonic acid (CSA), triethylamine (TEA), m-phenylenediamine (MPD), sodium dodecyl sulfate (SDS), trimesoyl chloride (TMC) and dicyandiamide were bought from Aladdin Reagent Co. Ltd. (China). N-Heptane, n-hexane, hydrochloric acid (37%, wt%), sodium hydroxide (NaOH), potassium chloride (KCl) and sodium chloride (NaCl) were purchased from Jiangtian Chemical Technology Co. Ltd. (China). COS, with a weight-average molecular weight of 1.5 kDa and a deacetylation degree (DD) of 0.90, was purchased from Qingdao Medicine Institute, Shandong, China. BSA (96%) and lysozyme were purchased from Dingguo Changsheng Biotech Co. Ltd. (China). E. coli and B. subtilis were provided by Transgen Biotech Co. Ltd. (China). Pure water with a conductivity of less than 10 μS cm−1 was obtained from a RO system.
2.2. Synthesis and characterization of COSG
COSG was synthesized by the guanidination reaction of COS and dicyandiamide, according to the previous study.52 The synthetic route of COSG is presented in Fig. 1. Initially, COS (5 g) was dissolved in 50 mL of hydrochloric acid (0.2 mol L−1) under magnetic stirring to form a homogeneous solution. The desired amount of dicyandiamide (corresponding to a molar ratio of 1
:
1 compared with COS) was dissolved in 50 mL of pure water at 60 °C. Then, the COS solution and dicyandiamide solution were mixed in a 250 mL three-necked flask and adjusted the pH value to 1 by hydrochloride solution (5 mol L−1). As shown in Fig. 2, the working power of the microwave was set to 400 W. The flask was put in it and stirred at 50 °C for 15 min. After the guanidination reaction, the mixture was poured into a dialysis bag with molecular weight cut-off of 500. After it was dialyzed for three days, the solution was poured into anhydrous ethanol to precipitate. The white precipitates were filtered and washed several times and dried to constant weight at 60 °C. Finally, the dried product named as COSG was obtained.
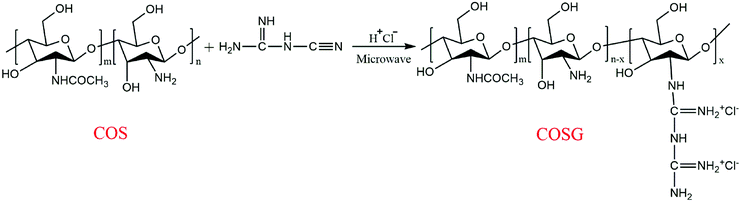 |
| Fig. 1 Synthetic route of COSG. | |
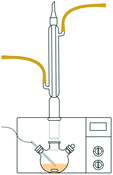 |
| Fig. 2 Schematic diagram of microwave synthesis. | |
The chemical structures of COS and COSG were measured by Fourier transform infrared (FTIR) spectroscopy (FTS-6000, Bio-Rad Inc., America). The 13C-NMR spectra of COS and COSG were measured by a NMR spectrometer (Varian Unity Inova-500 MHz, Varian Associates). Carbon and nitrogen contents of the synthesized COSG were determined by a Vario EL elemental analyzer (Germany).
2.3. Membrane preparation
The virgin membrane was prepared through the typical interfacial polymerization (IP) process which was the same as our previous works.54,55 Except that, the modification process was convenient and fast. Firstly, two different concentrations of the grafting solutions (0.1 mol L−1 COS, 0.5 mol L−1 COS, 0.1 mol L−1 COSG, 0.5 mol L−1 COSG) were prepared. As shown in Fig. 3, after the first IP reaction, 50 mL grafting solution was poured on the nascent membrane. It was allowed to the second interfacial polymerization for approximately 1 minute. Then the grafting solution was decanted and the membrane was annealed in the oven at 80 °C for 6 min. The resulting membranes modified with COS and COSG were named COS-modified membrane and COSG-modified membrane, respectively. Finally, the prepared membranes were rinsed with pure water at room temperature and stored in pure water at 4 °C before use.
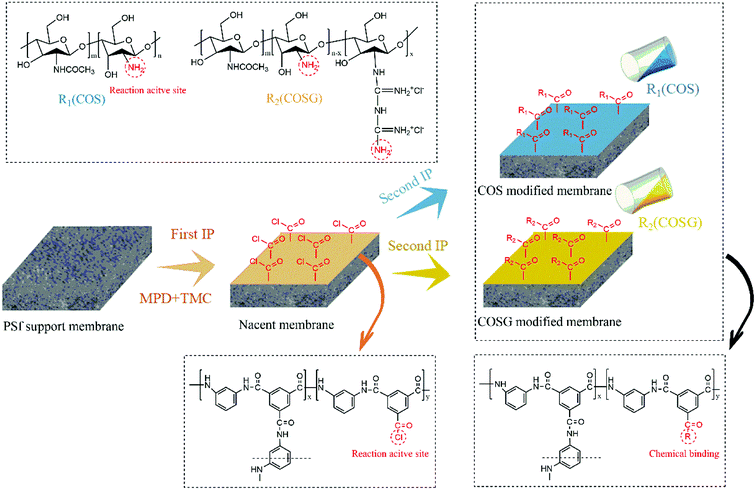 |
| Fig. 3 Schematic diagram of the COS-modified and COSG-modified membrane fabrication process. | |
2.4. Surface properties characterization
The prepared membranes were dried overnight under vacuum at 40 °C before test.
The elemental contents of the membrane surface were obtained by XPS (PHI5000VersaProbe, Japan). The morphology of the membrane surface was investigated by SEM (Nova NanoSEM430, USA) and AFM (Bruker Dimension Icon, Germany). The wettabilities of the membranes were evaluated by a contact angle analyser (Data Physics Instruments GmbH, Germany). The zeta potentials of the membranes were evaluated by an electrokinetic analyser (Anton Paar GmbH, Austria).56,57
2.5. Desalination performance evaluation
The desalination performances of the membranes were tested in a self-made RO test apparatus.54,55 The effective area of the membrane cell is 28.26 cm2. The filtration experiment was tested with 2000 mg L−1 NaCl aqueous solution and under the condition of 25.0 ± 0.1 °C, 1.55 MPa trans-membrane pressure (TMP), 1.5 L min−1 cross-flow velocity. The water flux (Jw) was obtained by directly weighing the permeate water. The salt rejection (R) were obtained according to the eqn (1): |
 | (1) |
where Cp and Cf represent the salt concentrations of the permeate and feed solution, respectively.
2.6. Anti-fouling property evaluation
Lysozyme and BSA were chosen as the model foulants, and the experiment was tested at the same TMP (1.55 MPa). Firstly, a 2000 mg L−1 NaCl aqueous solution was run for 40 minutes, and the water flux was measured. Then, the foulant with a concentration of 500 mg L−1 was added to the feed solution. The water flux of the membrane was recorded every one minute. After 6 h test, the feed solution was fully replaced by a 2000 mg L−1 NaCl solution, and the fouled membrane was rinsed under the condition of 0.62 MPa 4.0 L min−1 for 30 minutes to remove the foulants that loosely deposited on the membrane surface. Finally, the water flux was tested again. After the above test, the membranes were dried overnight under vacuum at 40 °C, and the surface morphology was observed by SEM. The flux decline (FD) and flux recovery (FR) were obtained according to the eqn (2) and (3), respectively: |
 | (2) |
|
 | (3) |
where J0, J1 and J2 represent the initial water flux after precompression for 40 minutes, water flux after fouling for 6 h and rinsing for 30 minutes, respectively.
2.7. Anti-bacterial property evaluation
The E. coli and B. subtilis were chosen to test the anti-bacterial property of the RO membranes by measuring the mortality rate.58 The E. coli and B. subtilis suspension were poured in the nutrient broth and incubated by shaking in a oscillator at 37 °C for 12 h. The prepared membrane samples were contacted with the bacterial suspensions (cell concentration: (1.0–1.5) × 106 CFU mL−1, inoculation quantity: (5.3–8.0) × 107 CFU m−2), respectively. The mortality rate at the certain contact time was obtained according to the eqn (4): |
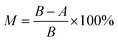 | (4) |
where M, A and B represent the mortality rate, the number of viable bacteria after 2 h of contact and the number of viable bacteria without contact (blank control).
2.8. Anti-biofouling property evaluation
The desalination performance and surface morphology of the membranes after biofouling were tested to determine the anti-biofouling property. The bacteria suspension (volume: 100 mL; cell concentration: (1.0–1.5) × 106 CFU mL−1) was poured on the membrane and incubated at 37 °C for 7 days, and the fresh nutrient broth was added every 12 h to remain bacteria active. Finally, the membranes were rinsed with pure water before the anti-biofouling property evaluation.
3. Results and discussion
3.1. COSG characterization
The FTIR spectra of the COS and COSG in the range of 500–3750 cm−1 are shown in Fig. 4. The band around 3373 cm−1 represents the stretching vibrations of O–H and N–H groups in the polymer chains, the band around 2890 cm−1 corresponds to the C–H stretching vibration, while the band at 1076 cm−1 in the spectrum of COS corresponds to the N–H bending vibration of secondary amines in the –NH–CO–CH3 group owing to the incomplete deacetylation of COS. Except that, the bands at 1527 cm−1 and 1378 cm−1 correspond to the N–H distortion vibration and C–N stretching vibration of the guanidine group, respectively. Moreover, the band at 1637 cm−1 corresponds to C
N stretching vibration. The three stronger bands at 1637 cm−1, 1527 cm−1, 1378 cm−1 indicated that the guanidination occurred on COS (as shown in Fig. 1). All these results confirmed that COSG was synthesized successfully.52,53,59
 |
| Fig. 4 FTIR spectra of COS and COSG. | |
The 13C-NMR spectra of the COS and COSG are shown in Fig. 5. The new chemical peaks of C7 (165.27 ppm) and C8 (155.30 ppm) which were corresponding to the carbons of biguanidine groups demonstrated the successful guanination of COS.52
 |
| Fig. 5 13C-NMR spectra of COS and COSG. | |
COS are generated by depolymerization of chitin or chitosan, hence the molecular formula of COS can be expressed as (C6H11NO4)DD·(C8H13NO5)1-DD.44 Assuming that substitution degree of guanidine is DS, the molecular formula of COSG can be expressed as (C6H11NO4)DD-DS·(C8H13NO5)1-DD·(C8H17N5O4)2+DS·Cl−2DS. Thus, the substitution degree of guanidine can be calculated by the eqn (5):
|
 | (5) |
The elemental contents (C and N) of the synthesized COSG were determined by elemental analyzer Vario EL (Germany). As a result, the contents of carbon and nitrogen were 30.12% and 9.29%, respectively. Based on the eqn (5), the substitution degree of guanidine was 18.45%. Thus, the molecular formula of the synthesized COSG is (C6H11NO4)0.7155·(C8H13NO5)0.1·(C8H17N5O4)2+0.1845·Cl0.369−.
3.2. Surface properties characterization
3.2.1. XPS analysis. The elemental compositions of membranes were characterized by XPS. As Table 1, the COS-modified membranes had higher oxygen (O) atom content than the virgin membrane, owing to the higher relative O content (–OH, C–O–C) of COS in comparison with the aromatic polyamide layer. In addition, the membranes modified by COSG had higher content of nitrogen (N) and lower content of carbon (C) compared with the COS-modified membranes due to the higher N element content of guanidyl. These results verified that COS and COSG had been successfully introduced to the membranes by second interfacial polymerization.
Table 1 The elemental contents and surface zeta potential of the virgin, COS-modified and COSG-modified membranes
Sample |
Atomic percent (mol%) |
Atomic ratio |
Zeta potential (mV) |
C |
N |
O |
C/N |
Virgin |
76.81 |
10.73 |
12.46 |
7.16 |
−51.37 ± 2.81 |
0.1% COS-modified |
75.57 |
10.59 |
13.84 |
7.14 |
−22.09 ± 1.74 |
0.5% COS-modified |
74.26 |
9.81 |
15.93 |
7.57 |
−10.62 ± 2.82 |
0.1% COSG-modified |
74.34 |
11.21 |
14.45 |
6.63 |
−12.35 ± 1.64 |
0.5% COSG-modified |
70.26 |
14.39 |
15.35 |
4.88 |
4.17 ± 1.93 |
3.2.2. Surface charge analysis. The surface charge of the virgin and modified membrane was investigated through a streaming potential measurement at pH 7 and 25 °C. The results are presented in Table 1. The virgin membrane was typical negatively charged attributing to the existence of the carboxylic acid groups.40 In comparison, the COS-modified and COSG-modified membranes had less negative charge. Typically, the zeta potential values of the membrane surfaces modified with 0.5% COS solution and 0.5% COSG solution were about −10.62 ± 2.82 mV and 4.17 ± 1.93 mV at pH 7.0, respectively. The almost neutral charged COSG-modified membrane was due to the protonation of primary amine groups on the COS and guanidine groups, which made the positive charge of the membranes increased.53 Meanwhile, the second IP process consumed the carboxylic acid groups on the selective layer.40,60 These changes further proved the successful guanidination of COS and the successful grafting of COS and COSG on the RO membrane surface.
3.2.3. Surface morphology analysis. The surface morphology of the membrane was observed by SEM and AFM. As Fig. 6, the typical ridge-and-valley structures were clearly observed and no significantly visual difference was detected. The membrane surface roughness is shown in Fig. 7 and Table 2. The roughness values of COS-modified and COSG-modified membrane surface declined with the increase of the modified solution concentration. For example, the Rp-v declined from 437.4 ± 16.1 nm of the virgin membrane to 366.6 ± 11.3 nm of 0.5% COSG-modified membrane. This was because the COS and COSG polymer chains filled the valley structure of RO membrane surfaces.
 |
| Fig. 6 SEM images of the virgin, COS-modified and COSG-modified membranes. | |
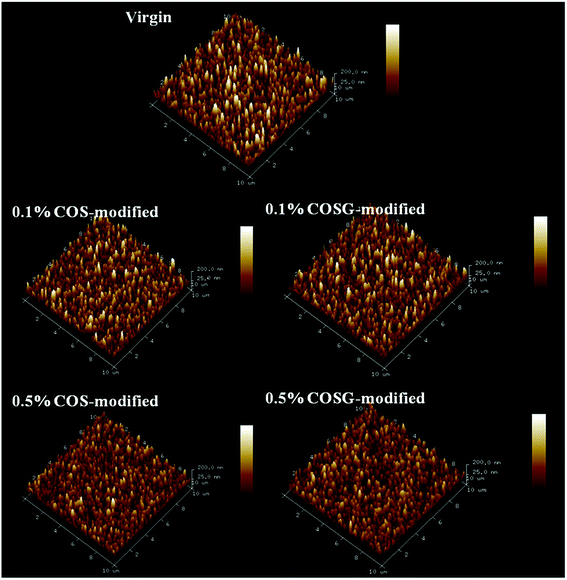 |
| Fig. 7 AFM images of the virgin, COS-modified and COSG-modified membranes. | |
Table 2 Roughness of the virgin, COS-modified and COSG-modified membranes
Sample |
Rrmsa (nm) |
Rab (nm) |
Rp-vc (nm) |
Root mean square roughness. Average roughness. Peak-to-valley distance. |
Virgin |
53.0 ± 1.1 |
42.2 ± 1.4 |
437.4 ± 16.1 |
0.1% COS-modified |
51.9 ± 1.6 |
41.5 ± 1.2 |
413.5 ± 13.8 |
0.5% COS-modified |
48.6 ± 1.3 |
39.0 ± 1.1 |
379.3 ± 12.6 |
0.1% COSG-modified |
51.9 ± 0.7 |
41.8 ± 0.9 |
409.5 ± 10.4 |
0.5% COSG-modified |
44.5 ± 1.2 |
35.8 ± 0.7 |
366.6 ± 11.3 |
SEM and AFM analysis results indicated that the influence of second interfacial polymerization on the surface morphology of membranes was negligible.
3.2.4. Wettability analysis. The membranes grafted with 0.5% COS and COSG solution were chosen to test the contact angle. As Fig. 8, the contact angle of the virgin membrane was 60.3 ± 4.8°. The contact angles of COS-modified and COSG-modified membrane were 11.2 ± 3.2° and 8.1 ± 3.6°, decreasing by 81.4% and 86.6% compared with the virgin membrane, respectively. It was due to the existence of polar groups (–OH, –NH2, C–O–C and guanidyl) in COS and COSG.61 The contact angle results might indicate the great increase of surface hydrophilicity after COS and COSG modification.
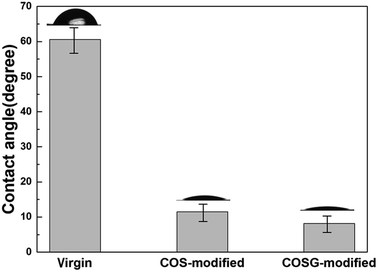 |
| Fig. 8 Contact angles of the virgin, COS-modified and COSG-modified membranes. | |
3.3. Desalination performance analysis
The desalination performance of the virgin, COS-modified and COSG-modified membranes were evaluated. As shown in Table 3, the water fluxes of COS-modified and COSG-modified membranes increased obviously. Especially, the water fluxes of 0.5% COS-modified and 0.5% COSG-modified membranes were 58.12 ± 2.10 L m−2 h−1 and 59.82 ± 1.63 L m−2 h−1, increasing by 13.29% and 16.61% compared with the virgin membrane, respectively. It was because the surface modified layer contained the hydrophilic amino, acylamide, hydroxy and guanidine. The enhanced surface hydrophilicity promoted the dissolution of water molecules in the membrane, which could increase the flux.62 Although the salt rejections of the modified membranes showed small decrease, the reductions were not significant. The small decrease may be due to the mild surface modification condition.
Table 3 Desalination performance of the virgin, COS-modified and COSG-modified membranes (test condition: 2000 mg L−1 NaCl, 1.55 MPa TMP, 1.5 L min−1, 25.0 ± 0.1 °C)
Sample |
Water flux (L m−2 h−1) |
Salt rejection (%) |
Virgin |
51.30 ± 1.52 |
99.03 ± 0.21 |
0.1% COS-modified |
54.57 ± 1.63 |
99.02 ± 0.18 |
0.5% COS-modified |
58.12 ± 2.10 |
98.75 ± 0.09 |
0.1% COSG-modified |
56.28 ± 0.94 |
98.92 ± 0.13 |
0.5% COSG-modified |
59.82 ± 1.63 |
98.91 ± 0.12 |
3.4. Anti-fouling property
In this work, the membranes grafted with 0.5% COS and COSG solution were chosen to conduct the fouling, anti-bacterial and biofouling experiments, because the membranes with this grafting concentration had a more hydrophilic, smoother, lower negatively charged surface, and better membrane permselectivities than the modified membranes with the other concentration. BSA and lysozyme were chosen to test the anti-fouling performance of the membranes and the experiment were tested for 450 min at equal trans-membrane pressure. As Fig. 9, the water fluxes declined rapidly at first and slowed down gradually. The FD and FR are presented in Table 4. After fouling, the water flux declines of the COS-modified and COSG-modified membrane were low. Meanwhile, the COS-modified and COSG-modified membranes could recover over 91% water flux after rinsing, which were much higher than the virgin membrane. The improved surface hydrophilicity, smoother surface morphology and lower negative charge of the modified membranes weakened the interaction between the foulants and the modified membrane, resulting in less adsorption and easier removal of foulants from the membrane surface.
 |
| Fig. 9 The effect of BSA (a) and lysozyme (b) fouling on the water fluxes of the RO membranes (fouling condition: 2000 mg L−1 NaCl, 500 mg L−1 foulant, 1.55 MPa TMP, 1.5 L min−1, 25.0 ± 0.1 °C; rinsing condition: 2000 mg L−1 NaCl, 0.62 MPa TMP, 4.0 L min−1, 25.0 ± 0.1 °C). | |
Table 4 The flux decline and flux recovery of the virgin, COS-modified and COSG-modified membranes (fouling condition: 2000 mg L−1 NaCl, 500 mg L−1 foulant, 1.55 MPa TMP, 1.5 L min−1, 25.0 ± 0.1 °C; rinsing condition: 2000 mg L−1 NaCl, 0.62 MPa TMP, 4.0 L min−1, 25.0 ± 0.1 °C)
Sample |
Virgin |
COS-modified |
COSG-modified |
BSA |
FD |
44.8% |
28.7% |
20.4% |
FR |
73.8% |
91.1% |
93.2% |
Lysozyme |
FD |
47.0% |
29.8% |
16.2% |
FR |
65.4% |
93.1% |
93.6% |
The surface morphology of membranes was characterized after fouling and rinsing. As shown in Fig. 10, the modified membranes had relatively less residual foulants and the ridge-and-valley structures were distinct.
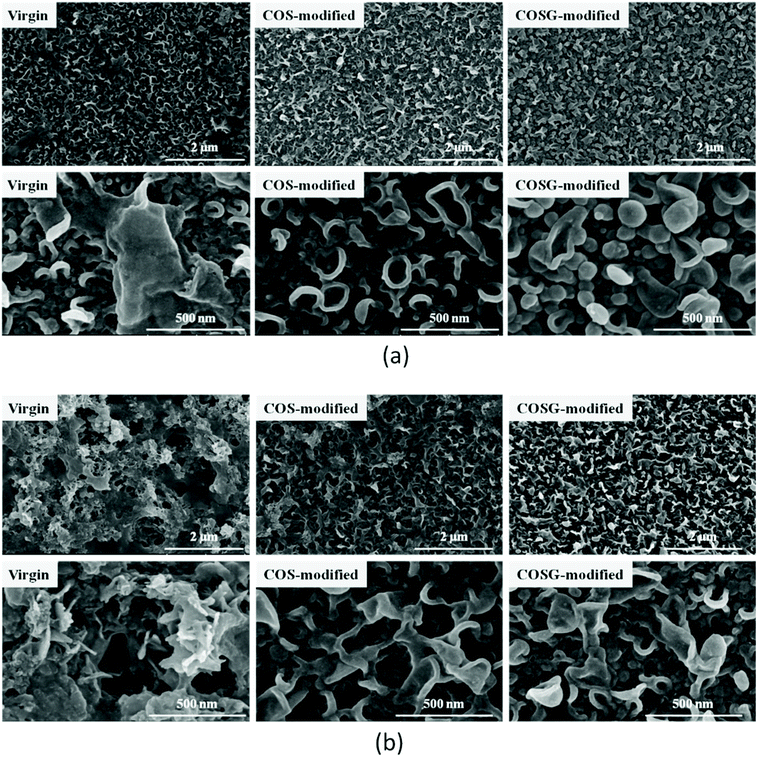 |
| Fig. 10 SEM images of the RO membranes after BSA (a) and lysozyme (b) fouling (fouling condition: 2000 mg L−1 NaCl, 500 mg L−1 foulant, 1.55 MPa TMP, 1.5 L min−1, 25.0 ± 0.1 °C; rinsing condition: 2000 mg L−1 NaCl, 0.62 MPa TMP, 4.0 L min−1, 25.0 ± 0.1 °C). | |
The above results indicated that the modified membranes were endowed with excellent anti-fouling property.
3.5. Anti-bacterial property
The sterilization property of the membranes were tested. As Fig. 11 and Table 5, the bacteria mortalities of the virgin membrane were about 25.0 ± 4.0% and 28.0 ± 2.2% for E. coli and B. subtilis, respectively. The bacteria mortalities of the COS-modified membrane were about 38.7 ± 2.9% and 41.5 ± 3.2% for E. coli and B. subtilis, respectively. The increased bacteria mortalities of COS-modified membranes were due to the protonated amine groups. It was supposed that the protonated amine groups might have the anti-bacterial property, because it could bind with the negatively charged macromolecules in bacteria cell leading to bacteria death.63,64
 |
| Fig. 11 The colonies of E. coli and B. subtilis suspensions after contacting with the RO membranes for 2 h (incubation level: (5.3–8.0) × 107 CFU m−2). | |
Table 5 The mortalities of E. coli and B. subtilis after contacting with the RO membranes (incubation level: (5.3–8.0) × 107 CFU m−2; contact time: 2 h)
Sample |
E. coli |
B. subtilis |
Virgin |
25.0 ± 4.0% |
28.0 ± 2.2% |
COS-modified |
38.7 ± 2.9% |
41.5 ± 3.2% |
COSG-modified |
99.9% |
99.9% |
As presented in Fig. 11, almost no colony could be observed for the COSG-modified membrane. The bacteria mortalities of the COSG-modified membrane were 99.9% for the two kinds of bacteria, demonstrating the strong anti-bacterial property of COSG-modified membranes against both the Gram-positive bacteria and Gram-negative bacteria. The excellent anti-bacterial property of the COSG-modified membrane was attributed to the high-activity guanidine groups on the membrane surface. The strong electrostatic interaction of COSG and the bacteria changed the permeability of the cell membrane, and resulted in intracellular material leakage.53 Finally, the bacteria lost biological activity.
3.6. Anti-biofouling property
The desalination performance of the membranes after biofouling are shown in Table 6. The water fluxes of the virgin membrane decreased about 29.4% and 20.2% of the initial flux for E. coli and B. subtilis, respectively. While the water fluxes of the COS-modified membrane decreased by 8.2% and 2.2%, respectively. In addition, the COSG-modified membrane reduced about 8.4% and 5.2% of the initial water flux, respectively. Meanwhile, the modified membranes exhibited higher salt rejection than the virgin membrane. As Fig. 12, the membrane surface of COS-modified and COSG-modified membrane could be observed less adhered bacteria than the virgin membrane. These results demonstrated the excellent anti-biofouling characteristics of the modified membranes.
Table 6 Flux and rejection of the membranes before and after biofouling test (inoculum level: (5.3–8.0) × 107 CFU m−2 for E. coli and B. subtilis, 37 °C, 7 d; test condition: 2000 mg L−1 NaCl, 1.55 MPa TMP, 1.5 L min−1, 25.0 ± 0.1 °C)
Sample |
Before biofouling |
E. coli |
B. subtilis |
Flux (L m−2 h−1) |
Rejection (%) |
Flux (L m−2 h−1) |
Rejection (%) |
Flux (L m−2 h−1) |
Rejection (%) |
Virgin |
51.30 ± 1.52 |
99.03 ± 0.21 |
36.2 ± 3.7 |
97.86 ± 0.13 |
40.9 ± 2.6 |
97.51 ± 0.43 |
COS-modified |
58.12 ± 2.10 |
98.75 ± 0.09 |
53.3 ± 1.8 |
99.14 ± 0.06 |
56.8 ± 1.3 |
99.02 ± 0.07 |
COSG-modified |
59.82 ± 1.63 |
98.91 ± 0.12 |
54.8 ± 1.1 |
99.08 ± 0.11 |
56.7 ± 0.9 |
99.05 ± 0.06 |
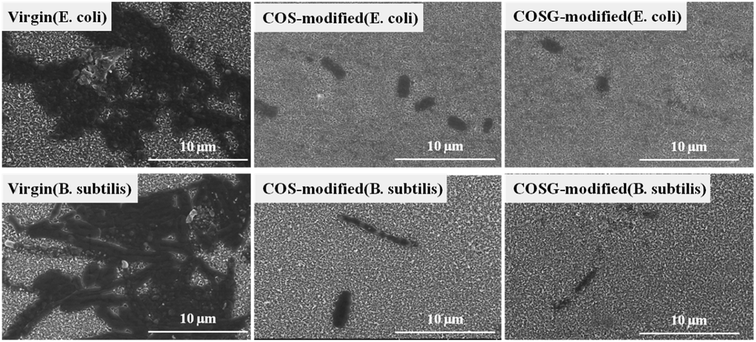 |
| Fig. 12 SEM images of the RO membranes after contacting with E. coli and B. subtilis suspensions for 7 days and rinsing with pure water (incubation level: (5.3–8.0) × 107 CFU m−2). | |
The bacterial colonies and the EPS, which were generated with the bacteria growing and reproduction could hinder the back diffusion of NaCl. Eventually, the osmotic pressure increased and the desalination performance of the virgin membrane decreased. The hydrated layer of the hydrophilic surface (COS-modified and COSG-modified) improved the anti-biofouling characteristics of the RO membrane by barricading against the adsorption of bacteria to the membrane surface. Meanwhile, the membrane modified with COSG exhibited a contact killing anti-bacterial property, which could prevent biofilm formation. Therefore, the modified membranes maintained high permselectivity.
4. Conclusions
In this work, a new anti-bacterial biguanidine functional chitooligosaccharide (COSG) was successfully synthesized and immobilized on the nascent RO membrane surface via second interfacial polymerization. The COSG fully combined the superiority of oligosaccharides and guanidine-based polymers, which possesses excellent hydrophilic and anti-bacterial properties. In addition, the modification method is simple, time-efficient and could be used for scale-up. The COSG-modified membrane showed neutral charge, significantly improved hydrophilicity and 16.61% increase of water flux. The membrane anti-fouling property was also improved with low flux decline ratios and high flux recovery ratios in organic fouling test. Moreover, the COSG-modified membrane exhibited killing ratio of 99.9% against E. coli and B. subtilis due to the high-activity guanidine groups. A small number of attached bacteria and the maintained permselectivity during severe biofouling tests confirmed the excellent anti-biofouling property of the COSG-modified membrane.
Conflicts of interest
There are no conflicts to declare.
Acknowledgements
This research was supported by Natural Science Foundation of China (No. 91534124), and Public Science and Technology Research Funds Projects of Ocean (No. 201405009).
References
- L. F. Greenlee, D. F. Lawler, B. D. Freeman, B. Marrot and P. Moulin, Water Res., 2009, 43, 2317–2348 CrossRef CAS PubMed.
- M. Elimelech and W. A. Phillip, Science, 2011, 333, 712–717 CrossRef CAS PubMed.
- A. G. Fane, R. Wang and M. X. Hu, Angew. Chem., Int. Ed., 2015, 54, 3368–3386 CrossRef CAS.
- D. M. Warsinger, S. Chakraborty, E. W. Tow, M. H. Plumlee, C. Bellona, S. Loutatidou, L. Karimi, A. M. Mikelonis, A. Achilli, A. Ghassemi, L. P. Padhye, S. A. Snyder, S. Curcio, C. D. Vecitis, H. A. Arafat and J. H. Lienhard, Prog. Polym. Sci., 2018, 81, 209–237 CrossRef CAS PubMed.
- M. Asadollahi, D. Bastani and S. A. Musavi, Desalination, 2017, 420, 330–383 CrossRef CAS.
- Q. Li and M. Elimelech, Environ. Sci. Technol., 2004, 38, 4683–4693 CrossRef CAS.
- G. Kang and Y. Cao, Water Res., 2012, 46, 584–600 CrossRef CAS PubMed.
- R. A. Al-Juboori and T. Yusaf, Desalination, 2012, 302, 1–23 CrossRef CAS.
- A. Ettori, E. Gaudichet-Maurin, J.-C. Schrotter, P. Aimar and C. Causserand, J. Membr. Sci., 2011, 375, 220–230 CrossRef CAS.
- T.-S. Kim and H.-D. Park, J. Membr. Sci., 2016, 507, 24–33 CrossRef CAS.
- X. Huang, K. L. Marsh, B. T. McVerry, E. M. V. Hoek and R. B. Kaner, ACS Appl. Mater. Interfaces, 2016, 8, 14334–14338 CrossRef PubMed.
- S.-H. Park, S. H. Kim, S.-J. Park, S. Ryoo, K. Woo, J. S. Lee, T.-S. Kim, H.-D. Park, H. Park, Y.-I. Park, J. Cho and J.-H. Lee, J. Membr. Sci., 2016, 513, 226–235 CrossRef CAS.
- Y. Wang, Z. Wang, X. Han, J. Wang and S. Wang, J. Membr. Sci., 2017, 539, 403–411 CrossRef CAS.
- S.-H. Park, S. O. Hwang, T.-S. Kim, A. Cho, S. J. Kwon, K. T. Kim, H.-D. Park and J.-H. Lee, Appl. Surf. Sci., 2018, 443, 458–466 CrossRef CAS.
- M. Piatkovsky, H. Acar, A. B. Marciel, M. Tirrell and M. Herzberg, J. Membr. Sci., 2018, 549, 507–514 CrossRef CAS.
- Z. Yang, D. Saeki and H. Matsuyama, J. Membr. Sci., 2018, 550, 332–339 CrossRef CAS.
- D. Rana and T. Matsuura, Chem. Rev., 2010, 110, 2448–2471 CrossRef CAS PubMed.
- K. Boussu, C. Vandecasteele and B. Van der Bruggen, J. Membr. Sci., 2008, 310, 51–65 CrossRef CAS.
- A. R. D. Verliefde, E. R. Cornelissen, S. G. J. Heijman, J. Q. J. C. Verberk, G. L. Amy, B. Van der Bruggen and J. C. van Dijk, J. Membr. Sci., 2008, 322, 52–66 CrossRef CAS.
- Y. Zhou, S. Yu, C. Gao and X. Feng, Sep. Purif. Technol., 2009, 66, 287–294 CrossRef CAS.
- L. Zhao, P. C.-Y. Chang, C. Yen and W. S. Winston Ho, J. Membr. Sci., 2013, 425, 1–10 Search PubMed.
- M. Liu, Q. Chen, L. Wang, S. Yu and C. Gao, Desalination, 2015, 367, 11–20 CrossRef CAS.
- J. Wu, Z. Wang, Y. Wang, W. Yan, J. Wang and S. Wang, J. Membr. Sci., 2015, 495, 1–13 CrossRef CAS.
- Y. Wang, Z. Wang and J. Wang, J. Membr. Sci., 2018, 554, 221–231 CrossRef CAS.
- F. Perreault, M. E. Tousley and M. Elimelech, Environ. Sci. Technol. Lett., 2014, 1, 71–76 CrossRef CAS.
- H. Isawi, M. H. El-Sayed, X. Feng, H. Shawky and M. S. Abdel Mottaleb, Appl. Surf. Sci., 2016, 385, 268–281 CrossRef CAS.
- C. Dong, Z. Wang, J. Wu, Y. Wang, J. Wang and S. Wang, Desalination, 2017, 401, 32–41 CrossRef CAS.
- Q. Yu, J. Cho, P. Shivapooja, L. K. Ista and G. P. Lopez, ACS Appl. Mater. Interfaces, 2013, 5, 9295–9304 CrossRef CAS PubMed.
- C. X. Liu, D. R. Zhang, Y. He, X. S. Zhao and R. Bai, J. Membr. Sci., 2010, 346, 121–130 CrossRef CAS.
- G. Ye, J. Lee, F. Perreault and M. Elimelech, ACS Appl. Mater. Interfaces, 2015, 7, 23069–23079 CrossRef CAS PubMed.
- P. Gilbert and L. E. Moore, J. Appl. Microbiol., 2005, 99, 703–715 CrossRef CAS PubMed.
- F. C. Krebs, S. R. Miller, M. Lee Ferguson, M. Labib, R. F. Rando and B. Wigdahl, Biomed. Pharmacother., 2005, 59, 438–445 CrossRef CAS PubMed.
- Y. Guan, H. Xiao, H. Sullivan and A. Zheng, Carbohydr. Polym., 2007, 69, 688–696 CrossRef CAS.
- Y. K. Mathurin, R. Koffi-Nevry, S. T. Guehi, K. Tano and M. K. Oule, J. Food Prot., 2012, 75, 1167–1171 CrossRef CAS PubMed.
- V. Perla and S. S. Jayanty, Food Chem., 2013, 138, 1574–1580 CrossRef CAS PubMed.
- A. M. Salgueiro, M. D. Santos, J. A. Saraiva, F. Almeida, I. Sousa, J. Tedim, H. I. S. Nogueira and D. V. Evtuguin, Carbohydr. Polym., 2017, 175, 303–310 CrossRef CAS PubMed.
- D. Wei, Q. Ma, Y. Guan, F. Hu, A. Zheng, X. Zhang, Z. Teng and H. Jiang, Mater. Sci. Eng., C, 2009, 29, 1776–1780 CrossRef CAS.
- J. Nikkola, X. Liu, Y. Li, M. Raulio, H.-L. Alakomi, J. Wei and C. Y. Tang, J. Membr. Sci., 2013, 444, 192–200 CrossRef CAS.
- X. Li, Y. Cao, H. Yu, G. Kang, X. Jie, Z. Liu and Q. Yuan, J. Membr. Sci., 2014, 466, 82–91 CrossRef CAS.
- Y. Gao, S. Zhao, Z. Qiao, Y. Zhou, B. Song, Z. Wang and J. Wang, Desalination, 2018, 430, 74–85 CrossRef CAS.
- H. Zhang, Y. Gao and J. Gai, J. Mater. Chem. A, 2018, 6, 6442–6454 RSC.
- M. Kong, X. G. Chen, K. Xing and H. J. Park, Int. J. Food Microbiol., 2010, 144, 51–63 CrossRef CAS PubMed.
- J. Dou, Q. Xu, C. Tan, W. Wang, Y. Du, X. Bai and X. Ma, Carbohydr. Polym., 2009, 75, 119–124 CrossRef CAS.
- S.-K. Kim and N. Rajapakse, Carbohydr. Polym., 2005, 62, 357–368 CrossRef CAS.
- K. V. Harish Prashanth and R. N. Tharanathan, Trends Food Sci. Technol., 2007, 18, 117–131 CrossRef.
- M. Shen, S. Keten and R. M. Lueptow, J. Membr. Sci., 2016, 506, 95–108 CrossRef CAS.
- M. Shen, S. Keten and R. M. Lueptow, J. Membr. Sci., 2016, 509, 36–47 CrossRef CAS.
- K. Li, L. Liu, H. Wu, S. Li, C. Yu, Y. Zhou, W. Huang and D. Yan, Phys. Chem. Chem. Phys., 2018, 20, 29996–30005 RSC.
- T. Wei, L. Zhang, H. Zhao, H. Ma, M. S. J. Sajib, H. Jiang and S. Murad, J. Phys. Chem. B, 2016, 120, 10311–10318 CrossRef CAS PubMed.
- N. Zhang, S. Chen, B. Yang, J. Huo, X. Zhang, J. Bao, X. Ruan and G. He, J. Phys. Chem. B, 2018, 122, 4719–4728 CrossRef CAS PubMed.
- X. Zhao, Z.-Z. Qiao, J.-X. He and S. Yiang, J. Eng. Fibers Fabr., 2010, 5, 16–24 CAS.
- L. Wang, Z. Liu, X. Liu and Y. Wu, RSC Adv., 2016, 6, 90777–90785 RSC.
- P. Sahariah, B. M. Óskarsson, M. Á. Hjálmarsdóttir and M. Másson, Carbohydr. Polym., 2015, 127, 407–417 CrossRef CAS PubMed.
- M. Shi, Z. Wang, S. Zhao, J. Wang, P. Zhang and X. Cao, J. Membr. Sci., 2018, 555, 157–168 CrossRef CAS.
- W. Yan, Z. Wang, J. Wu, S. Zhao, J. Wang and S. Wang, J. Membr. Sci., 2016, 498, 227–241 CrossRef CAS.
- P. Fievet, M. Sbaï, A. Szymczyk, C. Magnenet, C. Labbez and A. Vidonne, Sep. Sci. Technol., 2004, 39, 2931–2949 CrossRef CAS.
- S. Tardu, Superlattices Microstruct., 2004, 35, 513–529 CrossRef CAS.
- Y. Gao, L. Liang, S. Zhao, Y. Qi, W. Zhang, X. Sun, Z. Wang, J. Wang and B. Song, RSC Adv., 2018, 8, 24690–24700 RSC.
- Q. Liu, Y. Li, X. Jin, Q. Peng, X. Liu and Y. Wu, J. Appl. Polym. Sci., 2016, 25, 133 Search PubMed.
- Y. Wang, Z. Wang, J. Wang and S. Wang, J. Membr. Sci., 2018, 549, 495–506 CrossRef CAS.
- A. Shakeri, H. Salehi and M. Rastgar, Carbohydr. Polym., 2017, 174, 658–668 CrossRef CAS PubMed.
- J. Xu, X. Feng and C. Gao, J. Membr. Sci., 2011, 370, 116–123 CrossRef CAS.
- J. Y. Kim, J. K. Lee, T. S. Lee and W. H. Park, Int. J. Biol. Macromol., 2003, 32, 23–27 CrossRef CAS PubMed.
- Y.-X. Mei, X.-Y. Dai, W. Yang, X.-W. Xu and Y.-X. Liang, Int. J. Biol. Macromol., 2015, 77, 330–335 CrossRef CAS PubMed.
Footnote |
† These authors contributed equally to this work. |
|
This journal is © The Royal Society of Chemistry 2018 |
Click here to see how this site uses Cookies. View our privacy policy here.