DOI:
10.1039/C8RA08551J
(Paper)
RSC Adv., 2018,
8, 39307-39313
Novel far-red-emitting SrGdAlO4:Mn4+ phosphors with excellent responsiveness to phytochrome PFR for plant growth lighting
Received
16th October 2018
, Accepted 19th November 2018
First published on 26th November 2018
Abstract
In this work, we reported on novel far-red-emitting SrGdAlO4:Mn4+ (SGA:Mn4+) phosphors towards application in plant growth lighting. The crystal structure and luminescence properties were investigated on the basis of X-ray diffraction, excitation and emission spectra, luminescence decay curves and temperature-dependent photoluminescence spectra. When excited by 353 nm, the SGA:Mn4+ phosphors showed a far-red emission band in the 650–800 nm wavelength range with peaks at 709 and 725 nm, which was due to the 2Eg → 4A2g electron transition of Mn4+ ion. The optimal Mn4+ doping concentration was about 0.1 mol%. The CIE chromaticity coordinates of the SGA:0.1%Mn4+ sample were (0.7064, 0.2934). The luminescence decay lifetimes decreased gradually from 1.263 to 0.868 ms with the increasing Mn4+ concentrations. Notably, the emission band of SGA:0.1%Mn4+ sample was well-matched with the absorption spectrum of phytochrome PFR, which indicated the SGA:Mn4+ were potential far-red-emitting phosphors for plant growth applications.
1. Introduction
In agricultural production, the lighting conditions are directly related to the success of agricultural production.1,2 With the development of modern agriculture, the demand and energy consumption of plant lighting are expanding.3 Using light-emitting diodes (LEDs) as artificial light sources has become an inevitable choice for agricultural development.4 In recent years, compared with conventional light sources including fluorescent and incandescent lamps, LEDs are considered as next-generation lighting sources and thus have been receiving considerable attention owing to their tremendous merits such as short response time, long lifetimes, energy saving, low cost, and reliability.5–14 Especially, the wavelengths of LEDs can be adjusted by using various phosphors to match with the spectral range of plant photosynthesis and photomorphogenesis, which could affect the plant growth and development by regulating phytochrome.15,16 It is known that different wavelengths of light have different effects on plant photosynthesis, of which blue around 450 nm (410–500 nm), red light around 660 nm (610–700 nm) and far-red light around 730 nm (700–740 nm) have the greatest impact on photosynthesis.17,18 Far-red light can be absorbed by phytochrome PFR to regulate the time of flowering and the process of the plant germination.19,20 Therefore, it is imperative to search for novel far-red phosphors with preferable luminescence performance.
Currently, Eu2+-doped nitride-based red phosphors such as CaAlSiN3:Eu2+ have been widely reported.21–23 However, the preparation of nitrides requires high temperature and high pressure, which leads to high synthesis cost. It is worth noting that because of their low cost and desirable spectral features, Mn4+-activated red phosphors have the potential to replace Eu2+ based nitrides.24–35 Compared with the Mn4+-doped fluorides, Mn4+-activated oxides generally show intense far-red emissions in the 650–800 nm wavelength range, which are more suitable for plant growth lighting.36–38 The materials of Mn4+-doped aluminates phosphors are cheaper than Mn4+-doped germinates phosphors. Recently, Mn4+-doped aluminates phosphors with eco-friendly preparation process and high chemical stability have been extensively investigated, such as SrMgAl10O17:Mn4+, Ca14Al10Zn6O35:Mn4+, and CaMg2Al16O27:Mn4+.39–41 When Mn4+ ions substitute for Al3+ ions in the [AlO6] octahedral sites owing to the similar ionic radii of Al3+ ions and Mn4+ ions,42 the phosphors would give rise to red emissions.43,44 Up to date, the luminescence properties of Mn4+-doped SrGdAlO4 phosphors have never been reported. The SrGdAlO4 (SGA) compound consists of many [AlO6] octahedrons and can be synthesized easily with cheap materials. Thus SGA is selected as a host for investigating the luminescence properties of Mn4+ and red emissions would be expected in Mn4+-activated SGA phosphors.
In this paper, we reported on Mn4+-doped SGA far-red-emitting phosphors prepared by a conventional high-temperature solid-state reaction method. The crystal structure and luminescence properties of SGA:Mn4+ phosphors were characterized by X-ray diffraction, excitation and emission spectra, decay lifetimes, and the temperature-dependent PL spectra were analyzed in detail. These results indicated that the SGA:Mn4+ phosphors were very suitable to be used as far-red-emitting materials for applications in plant growth LEDs.
2. Experimental
A series of SrGdAl1-xO4:xMn4+ (SGA:xMn4+; x = 0.05, 0.1, 0.2, 0.4, 0.6, and 0.8 mol%) phosphors were prepared by using a high-temperature solid-state reaction method. The raw materials of SrCO3 (analytical reagent, AR), Gd2O3 (99.99%), Al2O3 (AR), and MnCO3 (AR) were weighed according to the stoichiometric ratio and ground in an agate mortar. After that, the mixtures were put into the crucibles and pre-heated at 600 °C for 3 h, then reground and sintered again at 1500 °C for 6 h. After cooling down to room temperature naturally, the final obtained products were ground again into fine powders for subsequent characterization.
The X-ray diffraction (XRD) patterns of the samples were recorded on a Bruker D8 X-ray diffractometer with Cu Kα radiation (λ = 1.5406 Å). The photoluminescence (PL) and PL excitation (PLE) spectra and decay curves of as-obtained phosphors were measured using an Edinburgh FS5 spectrometer equipped with a 150 W continued-wavelength xenon lamp and a pulsed xenon lamp, respectively. Furthermore, the temperature-dependent PL spectra of the phosphors were obtained from the same instrument attached a temperature controlling system. The internal quantum efficiency (IQE) was measured by an Edinburgh FS5 spectrometer equipped with an integrating sphere coated with BaSO4.
3. Results and discussion
Fig. 1 shows the XRD patterns of SGA:xMn4+ (x = 0.1%, 0.4%, and 0.8%) phosphors. It was found that all the observed diffraction peaks were consistent with the standard JCPDS card of SGA (no. 24-1185), demonstrating that Mn4+ ions were well-doped into the host and there was no significant influence on the structure of SGA.
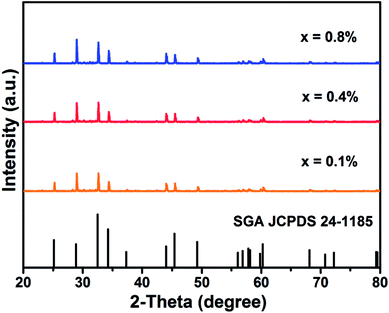 |
| Fig. 1 XRD patterns of SGA:xMn4+ (x = 0.1%, 0.4%, and 0.8%) phosphors and the standard profile of SGA (JCPDS no. 24-1185). | |
Fig. 2 shows the typical crystal structure of SGA. The SGA compound belonged to tetragonal crystal system with space group I4/mmm. The lattice constants were determined to be a = b = 3.697 Å, c = 12.360 Å, V = 168.9 Å3, and α = β = γ = 90°. It was obvious that Gd3+ and Sr2+ ions were coordinated with nine oxygen atoms around them, and the Al3+ ions were coordinated by six oxygen atoms to form [AlO6] octahedrons. Because of the similar ionic radii of Al3+ (r = 0.535 Å, coordination number (CN) = 6) and Mn4+ (r = 0.530 Å, CN = 6) ions, Mn4+ ions tended to replace Al3+ ions sites in SGA:Mn4+ phosphors.45
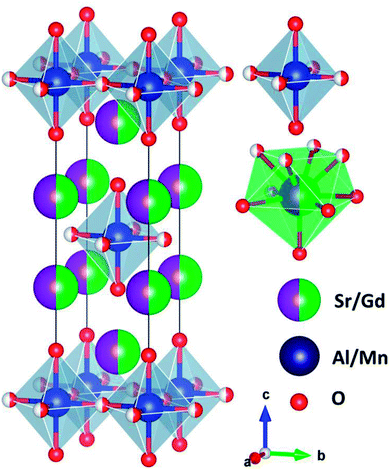 |
| Fig. 2 Typical crystal structure of SGA and the Al3+ and Sr2+/Gd3+ sites are coordinated by six and nine oxygen atoms, respectively. | |
Fig. 3(a) shows the PLE and PL spectra of SGA:0.1%Mn4+ sample at room temperature. When monitored at 709 nm, the obtained PLE spectrum of SGA:0.1%Mn4+ sample included two wide excitation bands peaking at 353 and 490 nm in the wavelength range from 250 to 600 nm, which were assigned to the Mn4+ spin-allowed transitions of 4A2g → 4T1g and 4A2g → 4T2g, respectively.46–48 The two broad bands in the PLE spectrum indicated that the SGA:Mn4+ phosphors could be excited by both ultraviolet (UV) and blue LED chips. When excited at 353 nm, the SGA:0.1%Mn4+ sample emitted bright far-red light. The PL spectrum consisted of a far-red emission band in the wavelength range of 650–800 nm with two sharp peaks at 709 and 725 nm, which was due to the spin-forbidden 2Eg → 4A2g transition of Mn4+.49–52 Fig. 3(b) compares the PL spectrum of SGA:0.1%Mn4+ phosphors and the absorption spectrum of phytochrome PFR. Obviously, there was an significant spectral overlap between the emission band of SGA:0.1%Mn4+ phosphors and the absorption of phytochrome PFR, implying that the SGA:Mn4+ phosphors could be potential far-red-emitting phosphors used in plant growth LEDs applications to promote plant development.53
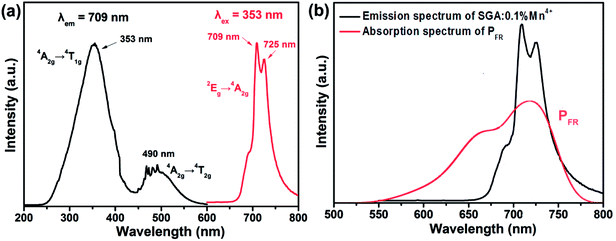 |
| Fig. 3 (a) PLE and PL spectra of SGA:0.1%Mn4+ phosphors. (b) PL spectrum of SGA:0.1%Mn4+ phosphors and the absorption spectrum of phytochrome PFR. | |
To determine the optimal Mn4+ doping concentration, the PL spectra of SGA:Mn4+ phosphors with different Mn4+ doping concentrations under 353 nm excitation were measured, as displayed in Fig. 4(a). The shapes and positions of the PL spectra of SGA:Mn4+ doped with various Mn4+ contents were very similar, but the emission intensities varied. As depicted in Fig. 4(b), when the Mn4+ doping concentration increased, the emission intensity of SGA:xMn4+ phosphors increased first and reached a maximum value at x = 0.1%, then decreased gradually with further increase in the Mn4+ concentrations. This phenomenon was attributed to concentration quenching effect, which was caused by the energy migration between neighboring Mn4+ activators.54,55 Because no spectral overlap was observed between PLE and PL spectra of SGA:Mn4+, so the radiation reabsorption was not the mechanism responsible for concentration quenching effect. If the distance between Mn4+ ions is higher than 5 Å, electric multipolar interaction is the dominant mechanism for concentration quenching effect; otherwise, exchange interaction becomes main mechanism for the concentration quenching.56,57 In order to investigate which mechanism was primary for the concentration quenching among the nearest Mn4+ ions of the SGA host, the critical distance (Rc) was roughly calculated using the following equation:58
|
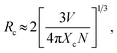 | (1) |
where
V is the volume of the unit cell,
Xc stands for the critical doping concentration of Mn
4+ ions, and
N represents the number of available sites for the dopant in the unit cell. For the SGA host, the
Xc = 0.1%;
V = 168.9 Å
3; and
N = 2. The calculated
Rc value was 54.43 Å, which was larger than 5 Å. Thus, the concentration quenching effect in the SGA:Mn
4+ phosphors was mainly caused
via electric multipolar interactions. Moreover, we can use the following equation to confirm the type of interaction mechanism among Mn
4+ ions:
58 |
I/x = K[1 + β(x)θ/3]−1,
| (2) |
where
I and
x are the PL intensity and doping concentration of Mn
4+ ions in SGA host, respectively;
β and
K are constants; and
θ represents an index of the electric multipolar character, where
θ = 6, 8 and 10 corresponding to dipole–dipole, dipole–quadrupole and quadrupole–quadrupole interactions, respectively.
59,60 Fig. 4(c) shows the plot of log(
I/
x)
versus log(
x). The slope (−
θ/3) of the fitted line was found to be −1.487, and thus the value of
θ was 4.461, which was close to 3. Therefore, the concentration quenching mechanism was the non-radiative energy transfer among the adjacent Mn
4+ ions in SGA:Mn
4+ phosphors, which was similar to the previous research.
53,61–63
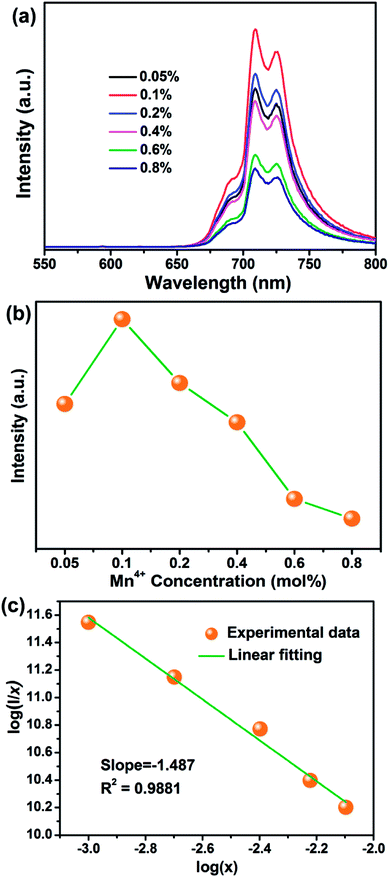 |
| Fig. 4 (a) PL spectra of SGA:xMn4+ (x = 0.05%, 0.1%, 0.2%, 0.4%, 0.6%, and 0.8%) phosphors under 353 nm excitation. (b) PL intensity of SGA:xMn4+ as a function of Mn4+ doping concentrations. (c) Linear fitting of log(x) versus log(I/x) in SGA:xMn4+ phosphors excited at 353 nm. | |
Fig. 5 illustrates the CIE chromaticity diagram of SGA:0.1%Mn4+ sample. According to its PL spectrum, the CIE chromaticity coordinates were calculated to be (0.7064, 0.2934), which were located in the far-red region. The color purity of SGA:0.1%Mn4+ sample was calculated to be 93%. As shown in the inset of Fig. 5, the SGA:0.1%Mn4+ sample emitted bright red light under 365 nm UV lamp. Therefore, the SGA:Mn4+ phosphors can be developed as potential far-red-emitting phosphors for applications in plant growth.
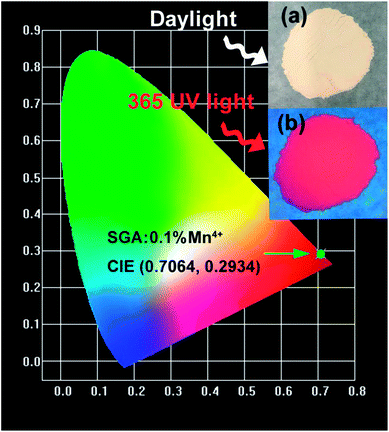 |
| Fig. 5 CIE chromaticity coordinates of SGA:0.1%Mn4+ phosphors under 353 nm excitation. The inset shows the digital photographs of SGA:0.1%Mn4+ phosphors in daylight and under a 365 nm UV lamp. | |
Fig. 6 shows PL decay curves of SGA:xMn4+ (x = 0.05%, 0.1%, 0.2%, 0.4%, 0.6%, and 0.8%) samples monitored at 709 nm and excited at 353 nm. All the decay curves could be well-fitted by the following equation:48
|
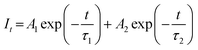 | (3) |
where
It is the luminescence intensities of SGA:
xMn
4+ phosphors at time
t,
A1 and
A2 represent constants, and
τ1 and
τ2 correspond to the short and long lifetimes for the exponential components, respectively. Furthermore, the average lifetime
τs could be calculated by the following equation:
48 |
τs = (A1τ12 + A2τ22)/(A1τ1 + A2τ2)
| (4) |
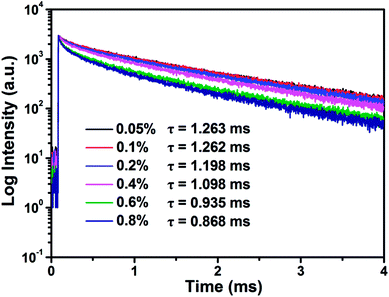 |
| Fig. 6 Decay curves and calculated lifetimes of SGA:xMn4+ (x = 0.05%, 0.1%, 0.2%, 0.4%, 0.6%, and 0.8%) under 353 nm excitation and monitored at 709 nm. | |
The decay times values were calculated and shown in Fig. 6. It was worth noting that the decay times of SGA:xMn4+ samples gradually decreased with increasing Mn4+ doping concentrations. The results was caused by the reduced distance between Mn4+–Mn4+ pairs in the host as high Mn4+ concentrations were doped, and then non-radiative transition rate increased among Mn4+ ions and finally the lifetimes of samples were decreased.64
Fig. 7(a) shows the temperature-dependent emission spectra of SGA:0.1%Mn4+ sample measured in the temperature range of 303 K to 463 K. With the excitation at 353 nm, it was obvious that there was no significant distinction among the emission spectra. However, the PL intensity of SGA:0.1%Mn4+ sample gradually decreased with increasing temperature, which was due to thermal quenching.65 Fig. 7(b) shows the normalized PL intensity as a function of temperature. It was found that the intensity of the sample decreased to ∼50% of the initial emission intensity when the temperature at 383 K (110 °C). The value of activation energy Ea was crucial for thermal quenching effect, which could be evaluated using the equation as follows:66
|
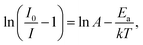 | (5) |
where
I0 and
I are the emission intensity of the SGA:0.1%Mn
4+ phosphor at initial temperature and at different given temperatures
T, respectively;
k stands for the Boltzmann constant;
A is the constant; and
Ea represents activation energy. According to the equation, the fitted linear relationship between ln(
I0/
I − 1) and 1/
kT was given in
Fig. 7(c). Thus, the value of
Ea was determined to be 0.367 eV. Furthermore, the IQE value of SGA:0.1%Mn
4+ phosphors was 23%, which was higher than several other Mn
4+ doped oxides, such as Ca
14Al
10Zn
6O
35:Mn
4+ (IQE: 19.4%),
2 and Na
2MgAl
10O
17:Mn
4+ (IQE: 22.7%).
67
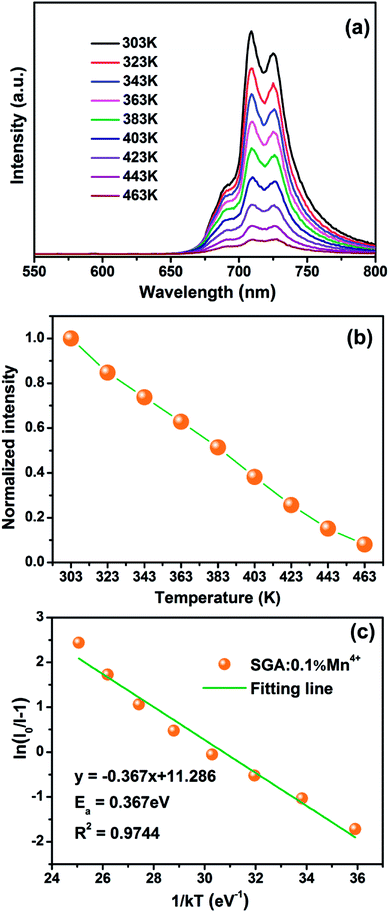 |
| Fig. 7 (a) Temperature-dependent PL spectra of SGA:0.1%Mn4+ phosphors under 353 nm excitation. (b) The normalized PL intensities of SGA:0.1%Mn4+ phosphors at different temperature from 303 to 463 K. (c) The plot of ln(I0/I − 1) versus 1/kT. | |
4. Conclusions
In conclusion, a novel series of far-red-emitting SGA:Mn4+ phosphors were synthesized by a conventional high-temperature solid-state reaction method. The phosphors could be efficiently excited by both UV and blue LED chips and showed far-red emission between 650 and 800 nm peaking at 709 nm and 725 nm. The emission band of the SGA:Mn4+ phosphors was matched well with the absorption spectrum of phytochrome PFR. The optimal Mn4+ doping concentration was about 0.1 mol% and the corresponding CIE chromaticity coordinates were (0.7064, 0.2934). The concentration quenching mechanism was the non-radiative energy transfer among the adjacent Mn4+ ions in SGA:Mn4+ phosphors. Moreover, the value of Ea was determined to be 0.367 eV. All above results proved that the SGA:Mn4+ phosphors could emit brilliant far-red light for applications in plant growth.
Conflicts of interest
There are no conflicts to declare.
Acknowledgements
This work was supported by the National Natural Science Foundation of China (No. 51502190), the Program for the Outstanding Innovative Teams of Higher Learning Institutions of Shanxi, and the Open Fund of the State Key Laboratory of Luminescent Materials and Devices (South China University of Technology, No. 2017-skllmd-01).
References
- Z. Zhou, M. Xia, Y. Zhong, S. Gai, S. Huang, Y. Tian, X. Lu and N. Zhou, J. Mater. Chem. C, 2017, 5, 8201–8210 RSC.
- L. Li, Y. Pan, Z. Chen, S. Huang and M. Wu, RSC Adv., 2017, 7, 14868–14875 RSC.
- J. Deng, H. Zhang, X. Zhang, Y. Zheng, J. Yuan, H. Liu, Y. Liu, B. Lei and J. Qiu, J. Mater. Chem. C, 2018, 6, 1738–1745 RSC.
- J. Long, X. Yuan, C. Ma, M. Du, X. Ma, Z. Wen, R. Ma, Y. Wang and Y. Cao, RSC Adv., 2018, 8, 1469–1476 RSC.
- X. Huang, J. Alloys Compd., 2017, 690, 356–359 CrossRef CAS.
- X. Huang, H. Guo and B. Li, J. Alloys Compd., 2017, 720, 29–38 CrossRef CAS.
- X. Huang, B. Li and H. Guo, Ceram. Int., 2017, 43, 10566–10571 CrossRef CAS.
- X. Huang, B. Li and H. Guo, J. Alloys Compd., 2017, 695, 2773–2780 CrossRef CAS.
- X. Huang, B. Li, H. Guo and D. Chen, Dyes Pigm., 2017, 143, 86–94 CrossRef CAS.
- X. Huang, S. Wang, B. Li, Q. Sun and H. Guo, Opt. Lett., 2018, 43, 1307–1310 CrossRef PubMed.
- B. Li, X. Huang, H. Guo and Y. Zeng, Dyes Pigm., 2018, 150, 67–72 CrossRef CAS.
- X. Huang and H. Guo, Dyes Pigm., 2018, 154, 82–86 CrossRef CAS.
- B. Li, G. Annadurai, L. Sun, J. Liang, S. Wang, Q. Sun and X. Huang, Opt. Lett., 2018, 43, 5138–5141 CrossRef PubMed.
- H. Guo, B. Devakumar, B. Li and X. Huang, Dyes Pigm., 2018, 151, 81–88 CrossRef CAS.
- J. Xiang, J. Chen, N. Zhang, H. Yao and C. Guo, Dyes Pigm., 2018, 154, 257–262 CrossRef CAS.
- R. Cao, Z. Shi, G. Quan, T. Chen, S. Guo, Z. Hu and P. Liu, J. Lumin., 2017, 188, 577–581 CrossRef CAS.
- Q. Sun, S. Wang, B. Li, H. Guo and X. Huang, J. Lumin., 2018, 203, 371–375 CrossRef CAS.
- Z. Zhou, J. Zheng, R. Shi, N. Zhang, J. Chen, R. Zhang, H. Suo, E. M. Goldys and C. Guo, ACS Appl. Mater. Interfaces, 2017, 9, 6177–6185 CrossRef CAS PubMed.
- X. Huang, J. Liang, B. Li, L. Sun and J. Lin, Opt. Lett., 2018, 43, 3305–3308 CrossRef PubMed.
- Q. Sun, S. Wang, B. Devakumar, B. Li, L. Sun, J. Liang and X. Huang, RSC Adv., 2018, 8, 28538–28545 RSC.
- K. Uheda, N. Hirosaki, Y. Yamamoto, A. Naito, T. Nakajima and H. Yamamoto, Electrochem. Solid-State Lett., 2006, 9, H22–H25 CrossRef CAS.
- X. Huang, Nat. Photonics, 2014, 8, 748–749 CrossRef CAS.
- P. Du, X. Huang and J. S. Yu, Chem. Eng. J., 2018, 337, 91–100 CrossRef CAS.
- R. Cao, X. Ceng, J. Huang, H. Ao, G. Zheng, X. Yu and X. Zhang, Opt. Mater., 2016, 62, 706–710 CrossRef CAS.
- R. Cao, X. Ceng, J. Huang, X. Xia, S. Guo and J. Fu, Ceram. Int., 2016, 42, 16817–16821 CrossRef CAS.
- R. Cao, X. Liu, K. Bai, T. Chen, S. Guo, Z. Hu, F. Xiao and Z. Luo, J. Lumin., 2018, 197, 169–174 CrossRef CAS.
- R. Cao, Z. Shi, G. Quan, Z. Luo, P. Tang, H. Ao and X. Yu, Opt. Mater., 2016, 57, 212–216 CrossRef CAS.
- R. Cao, W. Wang, J. Zhang, S. Jiang, Z. Chen, W. Li and X. Yu, J. Alloys Compd., 2017, 704, 124–130 CrossRef CAS.
- R. Cao, J. Zhang, W. Wang, T. Chen, Q. Gou, Y. Wen, F. Xiao and Z. Luo, Opt. Mater., 2017, 66, 293–296 CrossRef CAS.
- R. Cao, J. Zhang, W. Wang, Z. Hu, T. Chen, Y. Ye and X. Yu, Mater. Res. Bull., 2017, 87, 109–113 CrossRef CAS.
- Y. Zhu, L. Huang, R. Zou, J. Zhang, J. Yu, M. Wu, J. Wang and Q. Su, J. Mater. Chem. C, 2016, 4, 5690–5695 RSC.
- Y. Zhu, L. Cao, M. G. Brik, X. Zhang, L. Huang, T. Xuan and J. Wang, J. Mater. Chem. C, 2017, 5, 6420–6426 RSC.
- M. Zhu, Y. Pan, Y. Huang, H. Lian and J. Lin, J. Mater. Chem. C, 2018, 6, 491–499 RSC.
- S. Zhang, Y. Hu, H. Duan, L. Chen, Y. Fu, G. Ju, T. Wang and M. He, RSC Adv., 2015, 5, 90499–90507 RSC.
- L. Qin, S. Bi, P. Cai, C. Chen, J. Wang, S. I. Kim, Y. Huang and H. J. Seo, J. Alloys Compd., 2018, 755, 61–66 CrossRef CAS.
- X. Huang and H. Guo, Dyes Pigm., 2018, 152, 36–42 CrossRef CAS.
- J. Liang, L. Sun, B. Devakumar, S. Wang, Q. Sun, H. Guo, B. Li and X. Huang, RSC Adv., 2018, 8, 27144–27151 RSC.
- G. Jiang, B. Yang, G. Zhao, Y. Liu, J. Zou, H. Sun, H. Ou, Y. Fang and J. Hou, Opt. Mater., 2018, 83, 93–98 CrossRef CAS.
- L. Meng, L. Liang and Y. Wen, J. Mater. Sci.: Mater. Electron., 2014, 25, 2676–2681 CrossRef CAS.
- W. Lu, W. Lv, Q. Zhao, M. Jiao, B. Shao and H. You, Inorg. Chem., 2014, 53, 11985–11990 CrossRef CAS PubMed.
- B. Wang, H. Lin, J. Xu, H. Chen and Y. Wang, ACS Appl. Mater. Interfaces, 2014, 6, 22905–22913 CrossRef CAS PubMed.
- D. Chen, Y. Zhou, W. Xu, J. Zhong, Z. Ji and W. Xiang, J. Mater. Chem. C, 2016, 4, 1704–1712 RSC.
- B. Wang, H. Lin, F. Huang, J. Xu, H. Chen, Z. Lin and Y. Wang, Chem. Mater., 2016, 28, 3515–3524 CrossRef CAS.
- M. Zhu, Y. Pan, L. Xi, H. Lian and J. Lin, J. Mater. Chem. C, 2017, 5, 10241–10250 RSC.
- Y. Chen, K. Wu, J. He, Z. Tang, J. Shi, Y. Xu and Z. Liu, J. Mater. Chem. C, 2017, 5, 8828–8835 RSC.
- K. Li, H. Lian and R. V. Deun, J. Lumin., 2018, 198, 155–162 CrossRef CAS.
- J. Zhong, S. Zhou, D. Chen, J. Li, Y. Zhu, X. Li, L. Chen and Z. Ji, Dalton Trans., 2018, 47, 8248–8256 RSC.
- J. Zhong, D. Chen, X. Chen, K. Wang, X. Li, Y. Zhu and Z. Ji, Dalton Trans., 2018, 47, 6528–6537 RSC.
- C. Yang, Z. Zhang, G. Hu, R. Cao, X. Liang and W. Xiang, J. Alloys Compd., 2017, 694, 1201–1208 CrossRef CAS.
- F. Xue, Y. Hu, L. Chen, H. Wu, G. Ju, T. Wang and L. Yang, Ceram. Int., 2017, 43, 15141–15145 CrossRef CAS.
- S. Liang, M. Shang, H. Lian, K. Li, Y. Zhang and J. Lin, J. Mater. Chem. C, 2016, 4, 6409–6416 RSC.
- S. Liang, M. Shang, H. Lian, K. Li, Y. Zhang and J. Lin, J. Mater. Chem. C, 2017, 5, 2927–2935 RSC.
- S. Wang, Q. Sun, B. Devakumar, L. Sun, J. Liang and X. Huang, RSC Adv., 2018, 8, 30191–30200 RSC.
- S. Zhang and Y. Hu, J. Lumin., 2016, 177, 394–401 CrossRef CAS.
- X. Ding, Q. Wang and Y. Wang, Phys. Chem. Chem. Phys., 2016, 18, 8088–8097 RSC.
- H. Guo and X. Huang, J. Alloys Compd., 2018, 764, 809–814 CrossRef CAS.
- H. Guo, X. Huang and Y. Zeng, J. Alloys Compd., 2018, 741, 300–306 CrossRef CAS.
- Y. Jin, Y. Hu, H. Wu, H. Duan, L. Chen, Y. Fu, G. Ju, Z. Mu and M. He, Chem. Eng. J., 2016, 288, 596–607 CrossRef CAS.
- A. Fu, Q. Pang, H. Yang and L. Zhou, Opt. Mater., 2017, 70, 144–152 CrossRef CAS.
- H. Chen, H. Lin, Q. Huang, F. Huang, J. Xu, B. Wang, Z. Lin, J. Zhou and Y. Wang, J. Mater. Chem. C, 2016, 4, 2374–2381 RSC.
- X. Huang and H. Guo, RSC Adv., 2018, 8, 17132–17138 RSC.
- H. Deng, Z. Gao, N. Xue, J. H. Jeong and R. Yu, J. Lumin., 2017, 192, 684–689 CrossRef CAS.
- R. Yu, H. M. Noh, B. K. Moon, B. C. Choi, J. H. Jeong, K. Jang, S. S. Yi and J. K. Jang, J. Alloys Compd., 2013, 576, 236–241 CrossRef CAS.
- W. Chen, Y. Cheng, L. Shen, C. Shen, X. Liang and W. Xiang, J. Alloys Compd., 2018, 762, 688–696 CrossRef CAS.
- Z. Lu, A. Fu, F. Gao, X. Zhang and L. Zhou, J. Lumin., 2018, 203, 420–426 CrossRef CAS.
- Z. Liu, G. Shao, W. Chen, G. Hu, L. Shen, Y. Cheng, X. Liang and W. Xiang, Opt. Mater. Express, 2018, 8, 2532–2541 CrossRef.
- Q. Peng, R. Cao, Y. Ye, S. Guo, Z. Hu, T. Chen and G. Zheng, J. Alloys Compd., 2017, 725, 139–144 CrossRef CAS.
|
This journal is © The Royal Society of Chemistry 2018 |
Click here to see how this site uses Cookies. View our privacy policy here.