DOI:
10.1039/C8RA08142E
(Paper)
RSC Adv., 2018,
8, 40984-40988
Double-ratiometric fluorescence imaging of H2Se and O2˙− under hypoxia for exploring Na2SeO3-induced HepG2 cells' apoptosis†
Received
1st October 2018
, Accepted 16th November 2018
First published on 7th December 2018
Abstract
Sodium selenite (Na2SeO3), as an anti-tumor drug for inducing tumor cells' apoptosis, has been widely studied under normoxic conditions and has been shown to exhibit oxidative stress process-induced apoptosis. However, since the real tumor environment is hypoxic, the actual mechanism is still unclear. Hence, considering the main metabolite of Na2SeO3 in the metabolic process to be hydrogen selenide (H2Se) and also that it can be converted to superoxide anion (O2˙−) instantaneously in the presence of oxygen, a dual-ratiometric fluorescence imaging system for simultaneous monitoring of the changes of H2Se and O2˙− induced by Na2SeO3-guided tumor cell apoptosis under hypoxic conditions was constructed. Two molecular probes NIR-H2Se and dihydroethidium were used to detect H2Se and O2˙−, respectively, whereas Rhodamine 110 was used as the reference fluorophore. Notably, H2Se levels significantly increased under hypoxic conditions, but there was no change in the level of O2˙−, which is inconsistent with the results of the previous researches. Therefore, we hypothesize that the mechanism of Na2SeO3-induced apoptosis for tumor cells is caused by reductive stress; also, this method can be applied for the future study of other anti-cancer selenium compounds.
Introduction
Selenium,1,2 as a common protective agent against cancer, can effectively reduce the morbidity and mortality of tumors including lung cancer, colorectal cancer, lymphoma, and prostate cancer.3–5 The evidence from latent periods, clinical stages and epidemiology supports the chemopreventive role of selenium.6–8 Therefore, sodium selenite (Na2SeO3) has recently become the research hotspot as an anti-cancer medicine due to its high efficiency, low toxicity and cost-effectiveness. However, the precise anti-cancer mechanism of how Na2SeO3 can induce tumor cell apoptosis remains poorly understood. To date, research has been done on tumor cell cultures with Na2SeO3 under normoxic conditions. It shows that the most generally accepted mechanism for Na2SeO3 treatment is by cell apoptosis induced by elevating the reactive oxygen species (ROS) levels and the involvement of oxidative stress.9–12 Specifically, hydrogen selenide (H2Se) is the key small molecule metabolite of Na2SeO3 in vivo, and it reacts with O2 to produce and accumulate a large amount of ROS (mainly the superoxide anion, O2˙−), resulting in tumor cell oxidative stress, which leads to apoptosis.13–16 Nevertheless, since the tumor microenvironment is hypoxic (0.7–1.8% O2)17 due to the rapid proliferation of cancer cells and imbalance of new blood vessel formation,18–20 it is still a great challenge to understand the anti-cancer mechanism in hypoxic conditions, which needs to be solved urgently.
To clarify the mechanism, fluorescent probes, which are promising detection tools21 due to their non-invasiveness, high sensitivity, selectivity, rapid response, and high spatial resolution,22–28 are the primary option. Unfortunately, these fluorescent probes are limited by environmental conditions, probe distribution, and instrument performance, thereby only showing changes in emission intensities.29,30 In contrast, ratiometric probes, using the ratio of two different emission wavelengths as the detection signal, provide a built-in correction to the above factors for a more accurate analysis.31–34
In this regard, a double-ratiometric method for simultaneous fluorescence imaging analysis to monitor the changes in H2Se and O2˙− levels in tumor cells was developed. The method studies the apoptosis process induced by pharmacological doses of Na2SeO3 in hypoxic conditions. Herein, we chose the fluorescence probe (NIR-H2Se, λex/λem = 688/735 nm) designed by our group35 and the commercial fluorescence probe (dihydroethidium, λex/λem = 370/420 nm)36,37 to monitor the changes in intracellular H2Se and O2˙− concentrations in both normoxic conditions and hypoxic conditions, respectively. Meanwhile, Rhodamine 110 (λex/λem = 496/532 nm) was chosen as a reference fluorophore. This method could potentially avoid the influences of certain external factors on detection accuracy including environmental conditions, light scattering and probe distribution, therefore improving the detection accuracy of the target molecules in vivo.38,39
Experimental
Materials
All chemicals were analytical-reagent grade and all solvents were purified by a conventional method before use. Rhodamine 110 was purchased from J&K (China). The NIR-H2Se probe was synthesized in our laboratory following a previous protocol.35 Dihydroethidium (DHE) and KO2 were purchased from Aladdin (China). Stock solutions (1 mM) for probes were prepared by dissolving probe in DMSO (Sigma-Aldrich). Sartorius ultrapure water (18.2 MΩ cm) was used throughout the experiments. H2Se was prepared by reacting Al2Se3 with H2O under an N2 atmosphere for 30 min at room temperature before use every time.40,41 O2˙− was generated from KO2 in DMSO or xanthine oxidase. Na2SeO3 was purchased from Sigma-Aldrich. Dulbecco's Modified Eagle Medium (DMEM) was obtained from British Biotechnology (China). HepG2 cells were purchased from the Committee on Type Culture Collection of the Chinese Academy of Sciences.
Instruments
The fluorescence spectra measurements were performed with an FLS-920 Fluorescence Spectrometer (Edinburgh, UK). The fluorescence imaging of cells was recorded on a TCS SP5 confocal laser scanning microscope with an objective lens (×40) (Leica, Germany). The cells were cultured in a 5% CO2/95% air incubator MCO-15 AC (Sanyo, Japan).
Fluorescence spectra measurements
Ten μM probe NIR-H2Se was mixed with 5 μM probe DHE, 1 μM Rhodamine 110 and 10 mM PBS (pH = 7.4); then, various amounts of H2Se (0, 1, 2, 4, 6, 8, 9, 10, 11 μM) were added. The fluorescence intensities were measured at λex/λem = 496/532 nm and λex/λem = 688/735 nm. For a parallel study, 5 μM probe DHE was mixed with 10 μM probe NIR-H2Se, 1 μM Rhodamine 110 and 10 mM PBS (pH = 7.4) and then, various amounts of O2˙− (0, 1, 2, 3, 4, 5, 6, 7, 8 μM) were added for fluorescence measurements at λex/λem = 496/532 nm and λex/λem = 488/638 nm.
Cell culture
HepG2 cells in the exponential phase of growth were used for the cell experiments. The concentrations of the counted cells were adapted to 1 × 106 cells per mL for confocal fluorescence imaging with a culture medium. The cells were cultured with DMEM and supplemented with 1% antibiotics (100 U mL−1 penicillin and 100 mg mL−1 streptomycin) and 10% fetal bovine serum (FBS) at 37 °C under a humidified atmosphere of 5% CO2/95% air (20% O2), 75% CO2/25% air (5% O2) and 95% CO2/5% air (1% O2) for normoxic and hypoxic conditions in an MCO-15AC incubator, respectively.
Confocal fluorescence imaging
HepG2 cells were prepared in glass-bottom culture dishes and cultured for 24 h. One group of cells without treatment of Na2SeO3 served as the control group and the other groups were treated with various amounts of Na2SeO3 (2, 5, 10 μM) for 12 h under either normoxic or hypoxic conditions. All groups were further incubated in an FBS-free culture medium mixed with 10 μM probe NIR-H2Se, 5 μM probe DHE and 1 μM Rhodamine 110 at 37 °C for 15 min and then washed with PBS (pH = 7.4, 10 mM) three times. Afterwards, the cells were imaged immediately using a confocal microscope with an objective lens (×40) and examined by CLSM with different laser transmitters. The emitted light intensities were collected with an META detector from 490 to 550 nm, 590 to 670 nm and 690 to 760 nm.
Results and discussion
Spectroscopic properties of NIR-H2Se with H2Se, DHE with O2˙− and Rhodamine 110
The excitation and emission spectra of NIR-H2Se with H2Se, DHE with O2˙− and Rhodamine 110 were recorded (Fig. S1†). Interestingly, the emission spectra of the reaction products of NIR-H2Se with H2Se, DHE plus O2˙−, and Rhodamine 110 are located at 735, 638 and 532 nm, respectively. Therefore, the emission spectra of Rhodamine 110 and the two reaction products exhibit a fairly ideal separation effect with approximately 100 nm between each peak, making their simultaneous detection possible (Fig. 1).
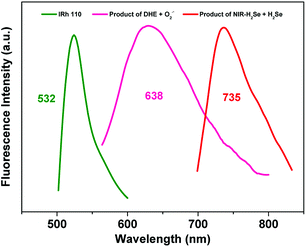 |
| Fig. 1 Fluorescence emission spectra of (red) NIR-H2Se with H2Se, (pink) DHE with O2˙− and (green) Rhodamine 110. | |
Ratiometric detection
The fluorescence responses of DHE to H2Se and NIR-H2Se to O2˙− were tested by using the mixture of NIR-H2Se, DHE and Rhodamine 110 to simultaneously monitor the levels of H2Se and O2˙−. As shown in Fig. 2a, O2˙− (5 μM) yielded a strong fluorescence signal with DHE (5 μM), but high concentrations of H2Se (15 μM) did not exhibit a fluorescence response. Similarly, the fluorescence response of NIR-H2Se (10 μM) to O2˙− (10 μM) was negligible, but a strong fluorescence signal was obtained with 10 μM H2Se (Fig. 2b). Then, the optical properties of the probe mixtures with Rhodamine 110 were recorded with various concentrations of H2Se in simulated physiological conditions (10 mM PBS, pH = 7.4). The fluorescence intensities increased with increasing concentrations of H2Se at 735 nm (Fig. 3a) but hardly fluctuated at 532 nm (Fig. 3b, black curve, right Y scale). Interestingly, there is a good linear correlation between the fluorescence intensity ratio F735/F532 and H2Se concentrations from 0 to 11.0 μM (Fig. 3b, red curve, left Y scale). The equation of linear regression is F735/F532 = 0.23 + 0.17 [H2Se] μM with the R value of 0.9937, indicating that the fluorescence intensity ratio F735/F532 increased with increasing concentrations of H2Se linearly. Simultaneously, similar fluorescence responses of the mixture towards O2˙− under various concentrations of O2˙− in simulated physiological conditions (10 mM PBS, pH = 7.4) were also obtained (Fig. 3c); the fluorescence intensity was significantly enhanced via increasing O2˙− concentrations at 638 nm but was maintained at 532 nm (Fig. 3d, black curve, right Y scale). Also, a linear correlation between the fluorescence intensity ratio F638/F532 and O2˙− concentrations from 0 to 8.0 μM (Fig. 3d, red circles, left Y scale) was observed. The equation of linear regression is F638/F532 = 0.47 + 0.20 [O2˙−] μM with the R value of 0.9923, indicating that the fluorescence intensity ratio of F638/F532 increased linearly with increasing concentrations of O2˙−. Overall, the results demonstrated that it is entirely feasible to use NIR-H2Se and DHE for simultaneously monitoring the changes in H2Se and O2˙− in a complex system.
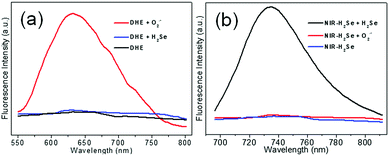 |
| Fig. 2 Interference measurement of (a) DHE to H2Se and (b) NIR-H2Se to O2˙−. All of the spectra were acquired at room temperature in 10 mM PBS (pH = 7.4) with (a) λex/λem = 488/638 nm or (b) λex/λem = 688/735 nm. | |
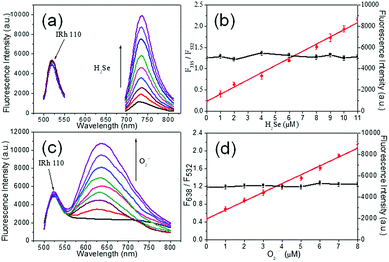 |
| Fig. 3 Ratiometric detection with the mixture of 10 μM NIR-H2Se, 5 μM DHE and 1 μM Rhodamine 110 toward H2Se and O2˙−. (a) Fluorescence response of the mixture to various concentrations (0 to 11.0 μM) of H2Se. (b) The linear correlation between the fluorescence intensity ratio F735/F532 and H2Se concentrations (red) and fluorescence intensities changes of Rhodamine 110 to H2Se concentrations (black). (c) Fluorescence response of the mixture to various concentrations (0 to 8.0 μM) of O2˙−. (d) The linear correlation between the fluorescence intensity ratio F638/F532 and O2˙− concentrations (red) and changes in the fluorescence intensity of Rhodamine 110 to O2˙− concentrations (black). The data shown are representative of repeating experiments (n = 3). | |
Co-staining imaging in living cells
To further test the simultaneous imaging of H2Se and O2˙− in vitro, co-staining experiments were carried out. Since Na2SeO3 metabolite H2Se is mainly accumulated in the liver, the cell model HepG2 was selected for the following study. HepG2 cells were first incubated in FBS-free culture medium mixed with 10 μM NIR-H2Se, 5 μM DHE and 1 μM Rhodamine 110 at 37 °C for 15 min and then, confocal fluorescence images were obtained. Fig. 4 displays the distribution of Rhodamine 110 (green), the product of DHE with native O2˙− of HepG2 cells (yellow), the product of NIR-H2Se with H2Se (red), and the merged image. The two probes and the reference achieved excellent spectral separation in the cell as well as great biocompatibility. The two probes' reaction products and the reference molecular structure all contain two amino groups, making the degree of impact by the intracellular complex environment almost the same, which further improved the sensitivity of the detection. Hence, the mixture of NIR-H2Se, DHE and Rhodamine 110 can be used to simultaneously recognize H2Se and O2˙− in living cells.
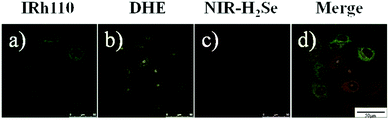 |
| Fig. 4 Co-staining images of HepG2 cells incubated with FBS-free culture medium mixed with 1 μM Rhodamine 110, 5 μM DHE and 10 μM NIR-H2Se at 37 °C for 15 min. The emitted light was collected with an META detector from 490 to 550 nm, 590 to 670 nm and 690 to 760 nm. (a) Green channel. (b) Yellow channel. (c) Red channel. (d) Merge of (a), (b) and (c). Scale bar = 50 μm. | |
Double-ratiometric simultaneous detection under normoxia and hypoxia
For the next step, the probe mixture was applied to explore its application for double-ratiometric, simultaneous imaging of endogenous H2Se and O2˙− in HepG2 cells pre-treated with Na2SeO3 under normoxic (20% O2) and hypoxic (5% O2, 1% O2) conditions. One group of HepG2 cells without treatment of Na2SeO3 served as the control group, and the other groups were treated with Na2SeO3 (2, 5, 10 μM) for 12 h under either normoxia (20% O2) or hypoxia (5% O2, 1% O2). Afterwards, the cells were incubated in an FBS-free culture medium mixed with 10 μM NIR-H2Se, 5 μM probe DHE and 1 μM Rhodamine 110 at 37 °C for 15 min. The ratiometric images of H2Se were achieved by combining the images obtained in the band path of 490–550 nm with the corresponding images in the band path of 690–760 nm. Meanwhile, the concentration of O2˙− was also obtained by combining the images obtained in the band path of 490–550 nm with the corresponding images in the band path of 590–670 nm (Fig. 5 and S2–S4†). Fig. 5a shows that under different oxygen concentrations, the concentration of H2Se in HepG2 cells increased by the Na2SeO3 concentration. From the quantitative map, it can be seen that with the decrease in oxygen concentration and increase in Na2SeO3 concentration, the H2Se content gradually increased. At 1% O2, the concentration of H2Se in the HepG2 cells was relatively high with 10 μM Na2SeO3 (Fig. 5b). Consequently, under different oxygen concentrations, the content of O2˙− in the HepG2 cells increased while increasing Na2SeO3 since the increase of oxygen and Na2SeO3 led to gradually rising O2˙− levels (Fig. 5c). At 20% O2, the concentration of O2˙− in the HepG2 cells was relatively high with 10 μM Na2SeO3 incubation, but the content of O2˙− in the HepG2 cells remained unchanged under the condition of 1% O2 (Fig. 5d).
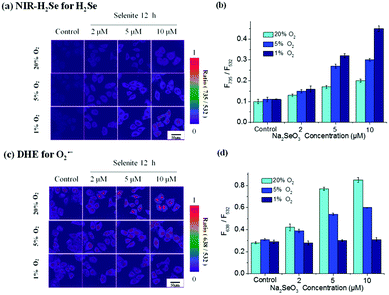 |
| Fig. 5 Confocal fluorescence double-ratiometric images of endogenous H2Se and O2˙− in living HepG2 cells treated with various concentrations of Na2SeO3 under normoxic and hypoxic conditions. (a) The fluorescence ratio image (F735/F532). (b) The corresponding statistic fluorescence ratio (F735/F532) for (a). (c) The fluorescence ratio image (F638/F532). (d) The corresponding statistic fluorescence ratio (F638/F532) for (c). The images shown are representative of repeated experiments (n = 3). Scale bar = 50 μm. | |
In this regard, higher H2Se contents were observed in hypoxic environments rather than in normoxic conditions in parallel experiments. Correspondingly, lower O2˙− contents were maintained and they hardly changed under hypoxic environments, whereas O2˙− contents gradually increased in the HepG2 cells with Na2SeO3 in a time- and dose-dependent manner under normoxic conditions. These results indicate that the Na2SeO3 anticancer mechanism is not an ROS-induced apoptosis process under hypoxic conditions. Thus, the anticancer effect of Na2SeO3 in solid tumors should be reductive stress to trigger cell death of cancer cells.
Conclusions
In summary, we developed a novel double-ratiometric fluorescence probe system for simultaneous fluorescence imaging analysis of the changes in H2Se and O2˙− levels in the tumor cell HepG2's apoptosis process induced by pharmacological doses of Na2SeO3. The system verifies that the content of O2˙− is clearly higher in the tumor cell apoptosis process induced by Na2SeO3 in normoxic conditions, and H2Se is almost completely oxidized by O2 to produce O2˙−. In contrast, under hypoxic conditions, the O2˙− content is extremely low, but the H2Se content increases dramatically. Therefore, in solid tumors, the anti-cancer mechanism of Na2SeO3 can be ascribed to reductive stress induced by H2Se but not oxidative stress. This finding will significantly improve the investigation of the anti-cancer mechanism of Na2SeO3, and the double-ratiometric fluorescence method can be used as a suitable tool for the study of other selenium compounds in the future.
Conflicts of interest
There are no conflicts to declare.
Acknowledgements
This work was supported by National Natural Science Foundation of China (21575081, 21775091, 21535004, 91753111 and 21705098) and the Key Research and Development Program of Shandong Province (2018YFJH0502).
References
- J. Chen, Y. Zhu and Y. Zhang, RSC Adv., 2016, 6, 62193–62199 RSC.
- Y. Nan, W. Zhao, X. Xu, C.-T. Au and R. Qiu, RSC Adv., 2015, 5, 69299–69306 RSC.
- Y. Cui, S. Vogt, N. Olson, A. G. Glass and T. E. Rohan, Cancer Epidemiol., Biomarkers Prev., 2007, 16, 1682–1685 CrossRef CAS PubMed.
- T. Chen and Y.-S. Wong, Int. J. Biochem. Cell Biol., 2009, 41, 666–676 CrossRef CAS PubMed.
- I. A. Asfour, M. M. El-Tehewi, M. H. Ahmed, M. A. Abdel-Sattar, N. N. Moustafa, H. M. Hegab and O. M. Fathey, Biol. Trace Elem. Res., 2009, 127, 200–210 CrossRef CAS PubMed.
- R. Sinha and k. El-Bayoumy, Curr. Cancer Drug Targets, 2004, 4, 13–28 CrossRef CAS PubMed.
- L. C. Clark, B. Dalkin, A. Krongrad, G. F. Combs Jr, B. W. Turnbull, E. H. Slate, R. Witherington, J. H. Herlong, E. Janosko, D. Carpenter, C. Borosso, S. Falk and J. Rounder, Br. J. Urol., 1998, 81, 730–734 CAS.
- Y. Xia, C. Wang, T. Xu, Y. Li, M. Guo, Z. Lin, M. Zhao and B. Zhu, RSC Adv., 2018, 8, 1917–1926 RSC.
- H.-M. Shen, C.-F. Yang and C.-N. Ong, Int. J. Cancer, 1999, 81, 820–828 CrossRef CAS.
- G. Nilsonne, X. Sun, C. Nyström, A.-K. Rundlöf, A. P. Fernandes, M. Björnstedt and K. Dobra, Free Radical Biol. Med., 2006, 41, 874–885 CrossRef CAS PubMed.
- L. Guan, B. Han, Z. Li, F. Hua, F. Huang, W. Wei, Y. Yang and C. Xu, Apoptosis, 2009, 14, 218–225 CrossRef CAS PubMed.
- J. J. An, K. J. Shi, W. Wei, F. Y. Hua, Y. L. Ci, Q. Jiang, F. Li, P. Wu, K. Y. Hui, Y. Yang and C. M. Xu, Cell Death Dis., 2013, 4, 973–982 CrossRef PubMed.
- H. Luo, Y. Yang, F. Huang, F. Li, Q. Jiang, K. Shi and C. Xu, Cancer Lett., 2012, 315, 78–85 CrossRef CAS PubMed.
- S.-H. Park, J.-H. Kim, G. Y. Chi, G.-Y. Kim, Y.-C. Chang, S.-K. Moon, S.-W. Nam, W.-J. Kim, Y. H. Yoo and Y. H. Choi, Toxicol. Lett., 2012, 212, 252–261 CrossRef CAS PubMed.
- X. Liu, B. Hu, R. Cheng, F. Kong, X. Pan, K. Xu and B. Tang, Chem. Commun., 2016, 52, 6693–6696 RSC.
- B. Hu, R. Cheng, X. Liu, X. Pan, F. Kong, W. Gao, K. Xu and B. Tang, Biomaterials, 2016, 92, 81–89 CrossRef CAS PubMed.
- S. Rey, L. Schito, M. Koritzinsky and B. G. Wouters, Adv. Drug Delivery Rev., 2017, 109, 45–62 CrossRef CAS PubMed.
- P. Vaupel, F. Kallinowski and P. Okunieff, Cancer Res., 1989, 49, 6449–6465 CAS.
- D. Hanahan and R. A. Weinberg, Cell, 2000, 100, 57–70 CrossRef CAS PubMed.
- M. C. Hung, G. B. Mills and D. Yu, Nat. Med., 2009, 15, 246–247 CrossRef CAS PubMed.
- E. Zhang, P. Ju, P. Guo, X. Hou, X. Hou, H. Lv, J.-j. Wang and Y. Zhang, RSC Adv., 2018, 8, 31658–31665 RSC.
- T. Ueno and T. Nagano, Nat. Methods, 2011, 8, 642–645 CrossRef CAS PubMed.
- L. L. Zhang, H. K. Zhu, C. C. Zhao and X. F. Gu, Chin. Chem. Lett., 2017, 28, 218–221 CrossRef CAS.
- F. Wang, Y. Zhu, L. Zhou, L. Pan, Z. Cui, Q. Fei, S. Luo, D. Pan, Q. Huang, R. Wang, C. Zhao, H. Tian and C. Fan, Angew. Chem., Int. Ed., 2015, 54, 7349–7353 CrossRef CAS PubMed.
- C. Zhao, X. Zhang, K. Li, S. Zhu, Z. Guo, L. Zhang, F. Wang, Q. Fei, S. Luo, P. Shi, H. Tian and W. H. Zhu, J. Am. Chem. Soc., 2015, 137, 8490–8498 CrossRef CAS PubMed.
- J. Xu, J. Pan, X. Jiang, C. Qin, L. Zeng, H. Zhang and J. F. Zhang, Biosens. Bioelectron., 2016, 77, 725–732 CrossRef CAS PubMed.
- X. Cheng, H. Jia, J. Feng, J. Qin and Z. Li, Sens. Actuators, B, 2013, 184, 274–280 CrossRef CAS.
- X. Gu, C. Liu, Y. C. Zhu and Y. Z. Zhu, J. Agric. Food Chem., 2011, 59, 11935–11939 CrossRef CAS PubMed.
- X. Liu, Q. Yang, W. Chen, L. Mo, S. Chen, J. Kang and X. Song, Org. Biomol. Chem., 2015, 13, 8663–8668 RSC.
- L. Yuan, W. Lin, Y. Xie, B. Chen and J. Song, Chem.–Eur. J., 2012, 18, 2700–2706 CrossRef CAS PubMed.
- L. Yuan, W. Lin, J. Song and Y. Yang, Chem. Commun., 2011, 47, 12691–12693 RSC.
- X. Jiang, J. Xu, Y. Zhang, H. Wang, L. Zeng and Y. Zhang, Anal. Methods, 2016, 8, 1572–1576 RSC.
- L. Zhu, J. Xu, Z. Sun, B. Fu, C. Qin, L. Zeng and X. Hu, Chem. Commun., 2015, 51, 1154–1156 RSC.
- Z. Ye, C. Duan, R. Sheng, J. Xu, H. Wang and L. Zeng, Talanta, 2018, 176, 389–396 CrossRef CAS PubMed.
- F. Kong, L. Ge, X. Pan, K. Xu, X. Liu and B. Tang, Chem. Sci., 2016, 7, 1051–1056 RSC.
- Z. Yu, Q. Sun, W. Pan, N. Li and B. Tang, ACS Nano, 2015, 9, 11064–11074 CrossRef CAS PubMed.
- R. M. Monaghan, R. G. Barnes, K. Fisher, T. Andreou, N. Rooney, G. B. Poulin and A. J. Whitmarsh, Nat. Cell Biol., 2015, 17, 782–792 CrossRef CAS PubMed.
- K. Komatsu, Y. Urano, H. Kojima and T. Nagano, J. Am. Chem. Soc., 2007, 129, 13447–13454 CrossRef CAS PubMed.
- D. Srikun, E. W. Miller, D. W. Domaille and C. J. Chang, J. Am. Chem. Soc., 2008, 130, 4596–4597 CrossRef CAS PubMed.
- C. Mealli, S. Midollini and L. Sacconi, Inorg. Chem., 1978, 17, 632–637 CrossRef CAS.
- V. V. Matylitsky, A. Shavel, N. Gaponik, A. Eychmüller and J. Wachtveitl, J. Phys. Chem. C, 2008, 112, 2703–2710 CrossRef CAS.
Footnotes |
† Electronic supplementary information (ESI) available. See DOI: 10.1039/c8ra08142e |
‡ X. G. and W. G contribute equally to this work. |
|
This journal is © The Royal Society of Chemistry 2018 |