DOI:
10.1039/C8RA08134D
(Paper)
RSC Adv., 2018,
8, 38765-38772
Sulfate radical oxidation combined with iron flocculation for upgrading biological effluent of coking wastewater†
Received
30th September 2018
, Accepted 3rd November 2018
First published on 19th November 2018
Abstract
Because the components of the coking wastewater was biologically toxic and hence inhibit the actions of microorganisms in conventional biological treatment processes,the biological effluent of coking wastewater (BECW) still remains much recalcitrant pollutants. In the current work, we set out to explore the feasibility of using a proposed advanced oxidation method, involving the persulfate-activated zero-valent iron system (PS/ZVI), to realize a deep treatment of BECW. The efficiency levels at which sulfate radical oxidation combined with iron flocculation removed pollutants, specifically TOC, phenolic compounds (PCs), cyanide, and suspended solids (SSs), as well as removing colour were investigated in batch tests. Increasing the persulfate concentration generally resulted in improved pollutant removal, with maximum removal efficiency levels of 58.5%, 68.4%, 61% 99.9% and 91.04% for TOC, PCs, SS, cyanide and colour, respectively. Note that the coexisting inorganic ions CO32− and HCO3− were strong competitors of the radical consumption of TOC, but this interference was eliminated by adjusting the pH to 4.5. Also, flocculation of the generated Fe3+ ions from the radical reaction significantly enhanced SS removal. GC-MS analysis showed that the compositional diversity of the BECW decreased after oxidation. Meanwhile its biodegradability increased, indicating less bio-toxicity reaching the natural water body. This study suggests that the PS/ZVI system may be an alternative safer and more efficient method than Fenton's method for carrying out an advanced treatment of coking wastewater.
1. Introduction
Industrial pollutants, due to their serious effects on the atmosphere, water, and soil, have attracted increasing attention worldwide in industrial wastewater treatment especially for their toxicity, stench, and refractory characteristics.1,2 Coking wastewater is one kind of the typical wastewater with high bio-toxicity, which makes it difficult to be degraded by microorganism metabolism. For example, though its chemical oxygen demand (COD) can be significantly decreased (with 200–300 mg L−1 COD3,4) during biological processes, the effluent still contains large complex pollutants including polycyclic aromatic hydrocarbons (PAHs), cyanide, phenolic compounds (PCs), and nitrogen heterocyclic compounds (NHCs), which may seriously pollute the air, surface water, groundwater, and soil around the source factory, and may even endanger the safety of human beings.5,6 PAHs, which have been identified as highly carcinogenic chemicals by the International Agency for Research on Cancer (IARC), can induce human oxidative stress and consequent metabolic and neuropsychological disorders.6 NHCs that exist in the aquatic environment have a higher eco-toxic effect on microorganisms.7 The half lethal dose (LD50) of pyridine, indole, and quinoline have been measured to be 891, 1000, and 460 mg kg−1, respectively.8 Cyanide, a constituent of acute toxic inorganics9 such as potassium cyanide (with a lethal dose (LD) of 506.4 mg kg−1), can enter the air rapidly as a result of volatilization.10 Consequently, to avoid diffusion and accumulation of the mentioned pollutants, advanced treatment of effluent from biological processes of coking wastewater to achieve minimal impact on the receiving ecosystems has become an important goal.
Advanced oxidation processes (AOPs) form an effective method to further reduce the concentration of bio-toxic organics in BECW for preventing damage to aquatic ecosystems. Studies on the removal characteristics of the AOPs have been reported in the literature, including Fenton's oxidation,11,12 ozone oxidation,13,14 absorption,15 and a combination of these methods. However, ozone oxidation consumes a lot of energy,16 and the Fenton and Fenton-like methods can produce a large amount of chemical sludge.13,17,18 Hence, the development of an alternative advanced treatment of coking wastewater has been urgently sought. In recent years, a promising oxidation approach using the sulfate radical (SO4−˙) with an oxidation potential of 2.5–3.1 eV has been proposed.19 And this approach has outperformed other approaches due to its extensive applicability to most organics, diversified activation (e.g., transition metals, alkali, and heat), and moderate reaction conditions.19,20
Since performance of SO4−˙ on BECW is relatively unexplored, especially for actual wastewater, we set out to investigate the feasibility of using the sulfate radical for the advanced treatment of BECW. Given the quenching effect by Fe2+, the zero-valent iron (ZVI) in the solid state was used as an activator to promote radical release. Also, special attention was focused on the effect of the concentration of Na2S2O8 on the change of total organic carbon (TOC), phenol compounds (PCs), cyanide and colour in the BECW. Due to the generated Fe3+ in flocculation and precipitation, the removal of the suspended solids (SS) was also investigated. In addition, to gain deep insight into the oxidation process, the diversity of the composition of the BECW before and after the oxidation reaction was evaluated by using a gas chromatograph-mass spectrometer (GC/MS) and its biodegradability was assessed by determining the ratio of the biological oxygen demand (BOD) to the chemical oxygen demand (COD). This research is expected to provide a new practical method for the comprehensive treatment of actual industrial wastewater.
2. Materials and methods
2.1. Raw wastewater
Raw wastewater was obtained from the effluent of the secondary sedimentation tank of the coking wastewater treatment plant in Jiaocheng City, Shanxi Province, China. The wastewater was subjected to centrifugation at 8000 rpm for 1 h, and the resulting supernatant was packed and stored at 4 °C in a refrigerator until radical oxidation tests were performed. The values of various characteristics of the raw water are listed in Table 1.
Table 1 Characteristics of the sample
Parameter |
COD mg L−1 |
BOD mg L−1 |
SS mg L−1 |
Phenol compounds mg L−1 |
Cyanide mg L−1 |
Organic carbon mg L−1 |
Inorganic carbon mg L−1 |
Color |
Average |
290 |
46.1 |
115 |
1.56 |
4.837 |
71.62 |
153.8 |
230 |
2.2. Materials
The basic chemical reagents used, including zero-valent iron (ZVI, Fe0), sodium persulfate (Na2S2O8), barium chloride (BaCl2), sodium hydroxide (NaOH), and sodium sulfide (Na2S), were of analytic grade. The chromatographically pure reagents used were methylene chloride (CH2Cl2) and methanol (CH4O). All of these reagents were purchased from the Shanxi Xinhua Chemical Factory, China. Neodymium magnets were purchased from the Shanghai Yinghao Magnetic Industry Co. LTD., China. Water was deionized by using an Aquelix 5 water system (Millipore Corp., Bedford, MA, USA).
2.3. Batch experiments design
Special attention was focussed on the concentration of Na2S2O8 and the state of ZVI in the experimental design, since these two factors were of great importance to the oxidation process, and could affect the release of sulfate radicals that attack the organics. As for the Na2S2O8 concentration, four different mass concentration ratios of Na2S2O8 to COD, namely 1
:
2, 1
:
4, 1
:
6, and 1
:
10, respectively, were investigated. Fe2+ was usually used as a potent activator of Na2S2O8 to release sulfate radical and initiate the oxidation process. However, if there is an excess Fe2+ in the solution, the free radicals can be quenched, resulting in an inhibition of the oxidation.21 Thus, ZVI used in this study was in the solid state, which ensured a slow release of Fe2+ ions with a lower likelihood of quenching. The specific reaction process is given in eqn (1)–(3).22 |
S2O82− + Fe2+ → SO4˙− + SO42− + Fe3+
| (3) |
A volume of 500 mL of BECW was added to a 1 L beaker, and Na2S2O8 was added according to the mass concentration ratio of COD to Na2S2O8. Then the reaction was activated by the addition of a certain amount of ZVI subjected to stirring at a speed of 300 rpm using a magnetic water bath. The experiments were operated at room temperature, specifically at 25 ± 0.5 °C, and the pH was not adjusted but had an initial value of 6.84. To eliminate any interference of coexisting inorganic composition, namely CO32− and HCO3−, on the radical-driven consumption of the target pollutants, the pH was adjusted to 4.5.
2.4. Determination of parameters
Supernatant samples were taken periodically during the oxidation process and filtered with a 0.45 μm filter membrane, followed by determining for these filtered samples their colours, biological oxygen demand (BOD), chemical oxygen demand (COD), and the concentrations of various compounds including TOC, PCs, cyanide, and SS. The amounts of total organic carbon (TOC) and inorganic carbon (IC) were determined by using a TOC-VCPH analyzer (TOC-VCPH, Shimadzu Corporation, Kyoto, Japan). The concentration of PCs was measured by applying the Chinese national standard analysis method (the 4-aminoantipyrine spectrophotometric method, HJ825-2017). The concentration of cyanide was determined by applying the colorimetric method after distillation.23 The color was determinded by the method of dilution multiple, and the SSs was measured by gravimetric method. The pH was measured using a portable pH meter (Mettler Toledo FE-20, Shanghai Miqingke Industrial Co. Ltd., China). The concentration of SO42− was measured using the barium sulfate precipitation method. Here, TOC was used because it is more accurate than the COD for determining the change in the concentration of total organics. The TOC was determined using eqn (4).
In this equation, IC and TC are the concentrations of the compounds that can release CO2 at 150 °C and 900 °C, respectively.
At the end of the experiment, the excess ZVI was separated from the suspensions by using a magnet. Then the samples were collected and analysed by performing GC/MS after being extracted by CH2Cl2 three times and then concentrated to 1 mL. And another sample not subjected to the radical reaction was analysed as a control using the same method. All analytical measurements were taken in triplicate. A Trace 2000 gas chromatographer connected to a Thermo DSQ™ (dual-stage quadrupole) mass spectrometer (DSQII, Thermo, USA) with a DB-5 column (30 m × 0.25 mm × 0.25 μm) was used for identifying the intermediate products. For this analysis, the carrier gas used was ultrapure helium (flowing at a rate of 1.0 mL min−1) – and the column temperature was set to 100 °C, held at this temperature for 1 min, then increased at a rate of 10 °C min−1 until a temperature of 280 °C was reached, then increased at a rate of 5 °C min−1 until 310 °C was reached, and held at this temperature for 2 min. The injector and interface temperatures were set at 300 and 280 °C, respectively. Full scan mode (m/z = 50–600) was used.
3. Results and discussion
3.1. Dependence of the removal of organics on persulfate dosage
The removal efficiencies for various mass concentration ratios are shown in Fig. 1. A clear increase in TOC removal efficiency was observed with the increasement of the persulfate dosage (Fig. 1b). As depicted, a 49.6% TOC removal efficiency was obtained with a COD/S2O82− ratio of 1
:
4, almost two times higher than that with a ratio of 1
:
2. With further increases in the persulfate dosage, the rate of increase in the removal efficiency declined and eventually the efficiency leveled off, especially after a COD/S2O82− ratio of 1
:
6, though the maximum efficiency peaked at 58.5% when the COD/S2O82− ratio reached 1
:
10. When pollutant removal efficiency and dosage economy of persulfate were taken into account, the most suitable mass concentration ratio of COD/Na2S2O8 was considered to be 1
:
6. At this ratio, the final TOC concentration after 35 min was 35 mg L−1 (corresponding to a 51% removal efficiency). The decline in removal efficiency at high persulfate dosage can be attributed to the more complicated side reaction that involved the consumption of SO4−˙ and PS by the coexisting compounds in the BECW,24 which will be elaborated upon later.
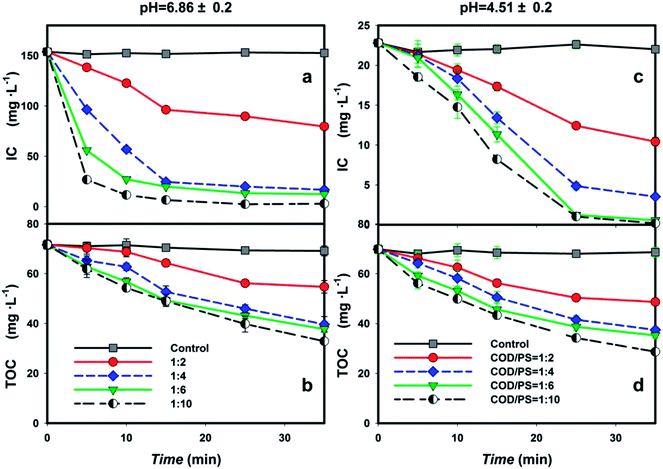 |
| Fig. 1 TOC values at various reaction time points and for various COD-to-PS mass concentration ratios for (a) and (b) for IC and TOC concentration for pHinitial = 6.8, (c) and (d) for IC and TOC concentration for pHinitial = 4.5. (Note: [COD]0 = 290 mg L−1, [TC]0 = 153.8 mg L−1, [TOC]0 = 71.62 mg L−1, temperature, 25 °C.) | |
Note that the IC removal rates for the above four ratios were 61.8%, 89.1%, 92%, and 98.1%, respectively, much higher than the TOC removal rates (Fig. 1a). The difference was due to the consumption of SO4−˙ by the chain reaction between the radicals and inorganic compounds such as HCO3−, CO32−, CN−, and SCN− in the BECW,21 which can occur immediately in the samples;24,25 this reaction generated another radical of CO3−˙ with a scarce capacity to remove organic matter.26 To eliminate any interference by coexisting inorganic components, namely CO32− and HCO3–, on the radical-driven consumption of target pollutants, the pH was adjusted to 4.5. When introducing this condition, the amount of IC removed by SO4−˙ in the acidic solution dramatically declined (Fig. 1c). Based on this, the targeting of the heterocyclic organics by the sulfate radical was improved as demonstrated by the higher removal of TOC. For COD/S2O82− ratios of 1
:
2, 1
:
4, 1
:
6, and 1
:
10, the TOC removal rates were 34.63%, 55.86%, 59.02%, and 63.08%, respectively, 2.73–9.00% higher than the TOC removal rates in the solution with a neutral pH value (Fig. 1d). In this sense, acidifying the solution before carrying out the radical reaction was essential for obtaining a high TOC removal efficiency.
Finally, the accelerated declines of both the TOC and IC concentrations after 10 minutes of reaction are worthy of attention. These declines may have resulted from a synergistic effect of the radical oxidation of the complex components in BECW by the addition of PS and flocculation sedimentation of suspended particles by the accumulation of generated Fe3+. While in the actual wastewater, the individual roles of the two factors were difficult to distinguish, the approximate time when iron began to function as a flocculant was noticed. As observed, small flocs appeared at five minutes of the reaction. Then these flocs grew slowly and began to settle from 10 minutes onwards. This change was in agreement with the bigger change in the concentration of the organics from 10 to 15 minutes than that within the first 5 minutes.
3.2. Phenol compound (PC) removal in BECW
Phenol compounds constitute an important component of coking wastewater, but are highly toxic and worth treating. Though a removal efficiency of 98% was obtained during biological treatment, the residual phenol concentration in the BECW was 1.56 mg L−1, still greater than the national standard of 0.3 mg L−1. As shown in Fig. 2, for each concentration set, the PC concentration decreased modestly, by less than 20%, within the first 10 minutes. These modest decreases can be explained by the SO4−˙ being relatively less activated in the solution as a result of the limited Fe2+ release by the slow reaction of solid ZVI.27 After the first ten minutes, a clear decrease of PC concentration was obtained in each case, and the rate of decrease was positively associated with the persulfate dosage. As a result, 35.6%, 48.3% and 62.1% of the PCs were removed when the mass ratios of the COD to PS were 1
:
2, 1
:
4 and 1
:
6, respectively. However, increasing the dosage further, to a mass ratio of 1
:
10, apparently did not yield an accelerated increase in the rate of PC removal, but an increase of only 6.3%, less than the former 12.7–13.8% increases for the lower dosages.
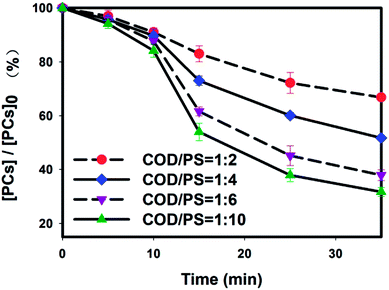 |
| Fig. 2 Time course profiles of PC concentration, resulting from PS/ZVI oxidation in EBCW, for various COD-to-PS mass concentration ratios. (Note: [COD]0 = 290 mg L−1, [PCs]0 = 1.56 mg L−1, temperature, 25 °C, pHinitial = 6.8.) | |
The moderate removal efficiency was attributed to the stable phenol structure with its good conjugation between the pi bond in the benzene ring and the lone pair electrons in the p orbitals of the hydroxyl, which made it more resistant to radical attack.28 In addition, the produced quinones, similar in having conjugated unsaturated ketones, were also stable structures and accumulated readily in the solution. This accumulation would also be expected to inhibit the PC oxidation process and lead to a low PC removal efficiency. Also, the rate constant of the PC reaction has been reported to be low (Table S1†).
3.3. Cyanide removal resulting from persulfate oxidation
As shown in Fig. 3, the cyanide removal rate was significantly affected by the PS/ZVI system. For each concentration set, the cyanide concentration sharply declined within 15 min, indicating the strong oxidation capacity of the persulfate system. As depicted, the higher persulfate concentration ensured a rapid and complete removal of cyanide. The mass ratio of 1
:
4 yielded a nearly 81% of the cyanide having been removed, 42% greater than that resulting from the 1
:
2 mass ratio. For the mass concentration ratios of 1
:
6 and 1
:
10, 99.9% of the cyanide was removed with within 15 minutes and 25 minutes, respectively. The rapid decline of cyanide has been reported to be due to not only the radical reaction, but also to oxidation by S2O82− ions, which have a high oxidation potential of 2.01 eV. And the reaction between S2O82− and cyanides has been confirmed in the literature by Guo.29
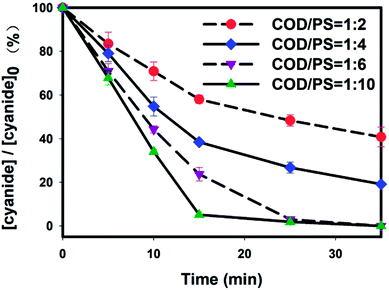 |
| Fig. 3 Time course profiles of cyanide concentration, resulting from PS/ZVI oxidation in EBCW, for various COD-to-PS mass concentration ratios. (Note: [COD]0 = 290 mg L−1, [cyanide]0 = 4.837 mg L−1, temperature, 25 °C, pHinitial = 4.5.) | |
Another radical reaction was more likely to have occurred during or even dominated the cyanide removal, since up to 4700 mg L−1 of Cl− was detected in the BECW (Table S2†). Lei recorded a chain reaction of Cl− with sulfate radical that produced another radical, namely Cl˙. Thus, given the high concentration of Cl− in the solution, the generated Cl˙ can make a great contribution to the cyanide removal.30 Furthermore, reaction between the sulfate radicals and the organics could generate H+ and further improve chlorate generation.24 And released H2OCl˙ and Cl˙ can then play a positive role in the cyanide oxidation reaction.
3.4. Change of colour in BECW
Fig. 4 shows the change in colour during the oxidation of the BECW by the PS/ZVI system. As depicted, a clear change from dark brown to light yellow was observed (Fig. 4a) after the radical reaction, and SS precipitation resulted from the application of persulfate. Clearly, the more sulfate radicals produced, the greater was the extent of color removal as confirmed by the colour changes under different mass ratios (Fig. 4b). As shown in the figure, the colour change during the first five minutes was almost independent of persulfate dosage. Then, after 5 minutes, the greater dosage resulted in greater declines in color intensity. A similar rapid decline in the intensity of the color of the solution was also observed for organics after 10 minutes. This decline can be attributed to the predominantly occurring iron flocculation. At the end of the 35 min reaction, 12.4%, 51.5%, 79.6%, and 91.0% of the colour was removed for mass ratios of 1
:
2, 1
:
4, 1
:
6, and 1
:
10, respectively.
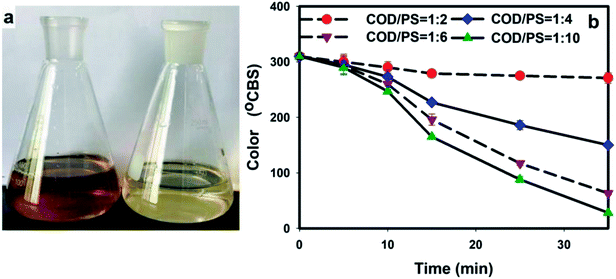 |
| Fig. 4 (a) Color change before and after the radical oxidation. (b) Time course profiles of the color of the BECW for various COD-to-PS mass concentration ratios. (Note: [PS]0 = 1740 mg L−1, colorinitial = 310 °CBS, pHinitial = 4.5, temperature, 25 °C.) | |
The colour of the coking wastewater can be attributed to large amounts of compounds including complex compounds formed by the residual ferric or cupric ion and cyanide or sulfur cyanide and chromogenic organics. The former can be eliminated by applying the cyanide removal method mentioned in Section 3.3, while removal of the latter was far more complicated. Preasphaltene, the primary product from dry distillation of coal during the coking process, was reported to be a typical chromogen and could be present in the BECW.31 Azo dyes can be formed during the coal processing.32 This formation may lead to an accumulation of chromogens in coking wastewater. Besides, many chromogenic groups such as the carboxyl (–COOH) group and those groups containing the C
C or C
O double bond were in the complicated compounds including butyronitrile, amine, azo-heterocyclic compounds, polycyclic aromatic hydrocarbon (PAH), ketones, etc.33 These groups can absorb ultraviolet and visible-light wavelengths with a high coefficient and complete transition between energy levels, which can darken the colour. Furthermore, the rich auxochromic groups (–NH2, –OH, –OR, –SR, –X, et al.) can also lead to increases in colour intensity.
And due to the low biodegradability of the mentioned compounds, large amounts of the chromogenic compounds were retained in the BECW and made it dark brown. Sulfate radicals can oxidize and decompose the chromogenic group of organics, further changing their structure and reducing the intensity of the colour.34,35 Huang36 confirmed the destruction of the azo bond (–N
N–) and the decomposition of the naphthalene ring to CO2 and H2O in orange G by sulfate radical attack. Methylene blue, with moderate reduction ability and strong polarity, was reported to be decolorized quickly by the sulfate radical.34 Another strong piece of evidence for the decolorization of the sulfate radical was the high efficiency of the removal of rhodamine B by sulfate radical oxidation, of note since rhodamine B includes C
O and –NH2 groups in its structure35 (Table S3†). Consequently, it was the strong oxidation by the sulfate radical that eliminated the chromogenic groups and led to the significant change in colour.
3.5. Suspended solid (SS) removal in BECW
Fig. 5 shows the SS removal characteristics in the solution. Due to the slow release rate of Fe2+ into the reaction system, the concentrations of sulfate radicals and Fe3+ ions were relatively low at the initial stage, before 5 minutes for instance. Then the SS concentration slightly changed. As shown, the SS concentration began to decrease rapidly as the solution became turbid and small floccules appeared after about 5 minutes. There was an approximately 13.3% reduction in the SS concentration over the first 10 minutes, which was most likely due to the ever-growing flocs with the greater Fe2+ release. This downward trend continued until the end of the test, when the SS concentration eventually fell below 150 mg L−1, with a 61% removal efficiency. Corresponding to the SS removal, the pH was observed to decline from the initial 7.0 value to 4.8.
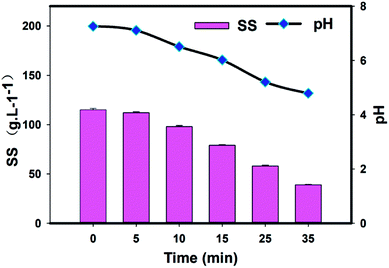 |
| Fig. 5 The time course profiles of SS and pH of the EBCW. (Note: [PS]0 = 1740 mg L−1, [SS]0 = 115 mg L−1, pHinitial = 6.8, temperature, 25 °C.) | |
The sudden increase in SS removal from 5 min onwards can be explained by the generation and accumulation of Fe3+ in the solution as the radical reaction proceeded. Further evidence for this explanation was the near simultaneous increase in the concentration of Fe3+ with the decrease in the SS concentration. The produced Fe3+ has been shown to be an active coagulant precursor with high binding affinity and is commonly used in drinking and wastewater treatment to precipitate organic and inorganic colloids.37–39 And it can bind tightly with negatively charged organic particles to remove the SS by flocculation and precipitation. It is noteworthy that accompanying the radical oxidation and ferric salt flocculation, a furl mechanism towards the large molecules was likely to occur, which would have further enhanced the SS removal efficiency.
3.6. Composition and biodegradability change in BECW
The change in the composition of BECW as a result of the persulfate oxidation was analyzed by acquiring GC-MS data of samples before and after the oxidation, as shown in Fig. 6. These spectra showed distinct differences between the diversities of these two samples. Most NHCs, including pyridine, quinoline, isoquinoline, indole, carbazole and their derivatives, were removed to one extent or another as a result of the oxidation. For example, the indole completely disappeared in 25 minutes of the reaction; in contrast carbazole, with its balanced and stable structure, remained in the solution. We also observed a partial removal of PCs, including phenol and naphthol, which was in accordance with the change in the PC characteristics as described above in Section 3.2. Note that the concentration of o-cresol increased, which can be explained by a partial conversion of phenol. While some organics (phenol, m-cresol, and carbazole) remained in the solution at the end of the 35 min reaction, the biodegradability of the solution was significantly enhanced.
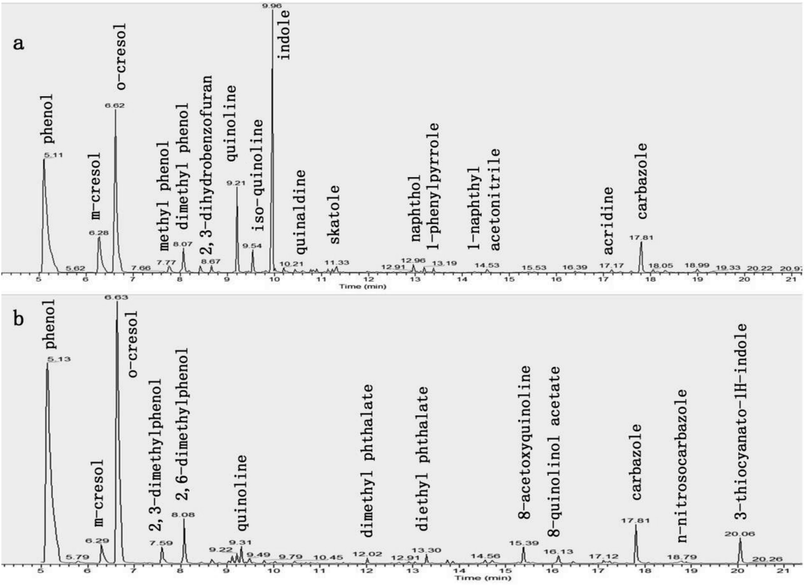 |
| Fig. 6 Compositions of the EBCW, (a) for the sample before radical oxidation, (b) for the sample after 35 min oxidation reaction. | |
Fig. 7 shows the time profile of the SO42− and BOD concentrations during the oxidation. The gradually increasing concentration of SO42− indicated that both the release of SO4−˙ and the radical reaction proceeded. And with the oxidation proceeding, the produced SO42− accumulated. Based on the initial concentration of SO42− (with 1130 mg L−1) and stoichiometric relationship of the conversion of S2O82− to SO42− (eqn (5)), more than 1260 mg L−1 SO42− was produced, indicating 90% of the oxidants were activated as SO4−˙, which ensured a high radical reaction rate.
|
[SO42−]increase = ([S2O82−]initial/238) × 64 × 2 − [SO42−]initial
| (5) |
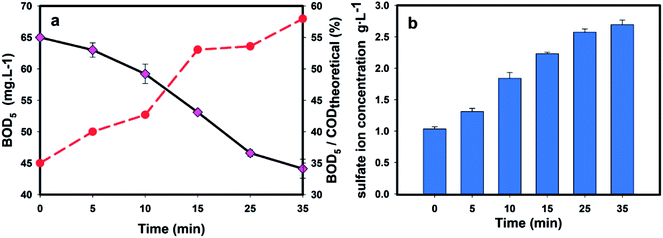 |
| Fig. 7 The time course profiles of (a) the biodegradability and (b) SO42− concentration of the EBCW solution. | |
And as a result of the radical oxidation, the BOD concentration sharply decreased, suggesting that the organics were removed. Conversely, a steady uptrend of the BOD/COD ratio was observed, indicating that more lower-toxicity structures were generated after the sulfate radical attack. As shown in Fig. 7b, the biodegradability increased by 29% from 0.45 to 0.58 after the oxidation, indicating its great potential in the decomposition of organic compounds as well as in pre-treatments before biological processes.
4. Conclusions
The proposed PS/ZVI system was proved to be feasible and effective at upgrading BECW, with a maximum removal efficiency of 58.5%, 68.4%, 61% 99.9% and 91.04% for TOC, PCs, SS, cyanide and colour, respectively. This significant removal was attributed to the combined effect of sulfate radical oxidation and iron flocculation. As demonstrated by the GC-MS and BOD/COD ratio analyses, the compositional diversity clearly declined and the biodegradability was significantly improved, further suggesting the great potential of the PS/ZVI system for actual wastewater with complex and refractory compounds. In conclusion, the persulfate-based oxidation process was safer than the Fenton reaction, whose pH needs to be adjusted to 3.0 and which has a danger of producing an explosion. Besides, the improved biodegradability by oxidation can enhance natural degradation of the residual organics in the discharged water.
Conflicts of interest
There are no conflicts to declare.
Acknowledgements
This research was supported by the National Natural Science Foundation of China (NSFC, No. 51608345), and by the Key Research and Development (R&D) Project of Shanxi Province (No. 201603D321012).
References
- W. Zhang, C. Wei, C. Feng, B. Yan, N. Li, P. Peng and J. Fu, Sci. Total Environ., 2012, 432, 396–403 CrossRef CAS PubMed.
- D. Park, D. S. Lee, Y. M. Kim and J. M. Park, Bioresour. Technol., 2008, 99, 2092–2096 CrossRef CAS PubMed.
- P. Ning, H. J. Bart, Y. Jiang, A. D. Haan and C. Tien, Sep. Purif. Technol., 2005, 41, 133–139 CrossRef CAS.
- C. A. Papadimitriou, P. Samaras and G. P. Sakellaropoulos, Bioresour. Technol., 2009, 99, 31–37 CrossRef PubMed.
- Z. Wang, J. Chen, F. Tian, P. Yang, X. Qiao and Z. Yao, Environ. Forensics, 2010, 11, 161–167 CrossRef CAS.
- J. Cheng, T. Yuan, Q. Wu, W. Zhao, H. Xie, Y. Ma, J. Ma and W. Wang, Water, Air, Soil Pollut., 2007, 183, 437–446 CrossRef CAS.
- X. Zhou, Y. Li and Y. Zhao, RSC Adv., 2014, 4, 15620–15629 RSC.
- B. Salehiforouz, A. A. Malekirad, M. Abdollahi, K. Rahzani and S. Mostafalou, Health, 2014, 6, 1230–1236 CrossRef CAS.
- A. Eisentraeger, B. H. Hollert, A. Sagner, A. Tiehm and J. Neuwoehner, Environmental Toxicology & Environ. Toxicol. Chem., 2010, 27, 1590–1596 Search PubMed.
- M. Jiang, Y. M. Li and G. W. Gu, Acta Sci. Circumstantiae, 2005, 25, 1253–1258 CAS.
- R. Kumar and P. Pal, Chem. Eng. J., 2012, 210, 33–44 CrossRef CAS.
- M. Dehua, L. Cong, Z. Xiaobiao, L. Rui and C. Lujun, Environ. Sci. Pollut. Res., 2016, 23, 1–10 CrossRef PubMed.
- Q. Wei, S. Qiao, B. Sun, H. Zou, J. F. Chen and L. Shao, RSC Adv., 2015, 5, 93386–93393 RSC.
- T. Lei, W. T. Zhao, X. Huang and X. C. Wang, China Water Wastewater, 2010, 26, 100–103 CAS.
- N. Zhang, G. F. Xue, P. Liu and L. N. Wang, Adv. Mater. Res., 2012, 573–574, 516–520 CAS.
- B. Mundy, B. Kuhnel, G. Hunter, R. Jarnis, D. Funk, S. Walker, N. Burns, J. Drago, W. Nezgod and J. Huang, Ozone: Sci. Eng., 2018, 40, 266–274 CrossRef CAS.
- K. Xiao, K. Pei, H. Wang, W. Yu, S. Liang, J. Hu, H. Hou, B. Liu and J. Yang, Water Res., 2018, 140, 232–242 CrossRef CAS PubMed.
- J. Bolobajev, E. Kattel, M. Viisimaa, A. Goi, M. Trapido, T. Tenno and N. Dulova, Chem. Eng. J., 2014, 255, 8–13 CrossRef CAS.
- Y. Feng, C. Liao, H. Li, C. Liu and K. Shih, Environ. Technol., 2018, 39, 1–11 CrossRef CAS PubMed.
- D. Zhang, L. Wu, J. Yao, H. Herrmann and H. H. Richnow, Chem. Eng. J., 2018, 374, 111–118 CrossRef.
- M. Nie, C. Yan, X. Xiong, X. Wen, X. Yang, Z. Lv and W. Dong, Chem. Eng. J., 2018, 348, 455–463 CrossRef CAS.
- Y.-d. Chen, S.-H. Ho, D. Wang, Z.-s. Wei, J.-S. Chang and N.-q. Ren, Bioresour. Technol., 2018, 247, 463–470 CrossRef CAS PubMed.
- APHA, AWWA, WEF, Standard Methods for the Examination of Water and Wastewater, APHA, Washington, DC, 22th edn, 2012 Search PubMed.
- H. V. Lutze, N. Kerlin and T. C. Schmidt, Water Res., 2015, 72, 349–360 CrossRef CAS PubMed.
- S. Wacławek, H. V. Lutze, K. Grübel, V. V. T. Padil, M. Černík and D. D. Dionysiou, Chem. Eng. J., 2017, 330, 44–62 CrossRef.
- S. Canonica, T. Kohn, M. Mac, F. J. Real, J. Wirz and U. Gunten, Environ. Sci. Technol., 2005, 39, 9182–9188 CrossRef CAS PubMed.
- X. Wei, N. Gao, C. Li, D. Yang, S. Zhou and L. Lei, Chem. Eng. J., 2016, 285, 660–670 CrossRef CAS.
- S. D. Brinkevich, N. I. Ostrovskaya, M. E. Parkhach, S. N. Samovich and O. I. Shadyro, Free Radical Res., 2012, 46, 295–302 CrossRef CAS PubMed.
- T. Guo, C. Dang, S. Tian, Y. Wang, D. Cao, Y. Gong, S. Zhao, R. Mao, B. Yang and X. Zhao, Chem. Eng. J., 2018, 347, 535–542 CrossRef CAS.
- Y. Lei, C. S. Chen, J. Ai, H. Lin, Y. H. Huang and H. Zhang, RSC Adv., 2015, 6, 866–871 RSC.
- Z. Wang, L. Li, H. Shui, Z. Wang, X. Cui, S. Ren, Z. Lei and S. Kang, Fuel, 2011, 90, 305–311 CrossRef CAS.
- S. Y. Yang, P. Wang, X. Yang, L. A. Shan, W. Y. Zhang, X. T. Shao and R. Niu, J. Hazard. Mater., 2010, 179, 552–558 CrossRef CAS PubMed.
- C. Lin, X. Zhuo, X. Yu, M. Yuan and C. H. Wei, RSC Adv., 2015, 5, 43786–43797 RSC.
- A. Ghauch and A. M. Tuqan, Chem. Eng. J., 2012, 183, 162–171 CrossRef CAS.
- Y. Leng, W. Guo, X. Shi, Y. Li and L. Xing, Ind. Eng. Chem. Res., 2013, 52, 13607–13612 CrossRef CAS.
- T. Huang, K. Zhang, Y. Qian, C. Fang and J. Chena, Environ. Sci. Pollut. Res., 2018, 25, 1–8 Search PubMed.
- H. Tang, J. P. Sha, Y. L. Ou and X. Zhao, Appl. Mech. Mater., 2015, 700, 426–430 Search PubMed.
- J. Y. Bottero and J. L. Bersillon, Adv. Chem., 1988, 425–442 Search PubMed.
- J. E. Greyor, C. J. Nokes and E. Fenton, Water Res., 1997, 31, 2949–2958 CrossRef.
Footnote |
† Electronic supplementary information (ESI) available. See DOI: 10.1039/c8ra08134d |
|
This journal is © The Royal Society of Chemistry 2018 |
Click here to see how this site uses Cookies. View our privacy policy here.