DOI:
10.1039/C8RA07919F
(Paper)
RSC Adv., 2018,
8, 38648-38655
Simultaneous adsorption of SO2 and CO2 in an Ni(bdc)(ted)0.5 metal–organic framework†
Received
24th September 2018
, Accepted 5th November 2018
First published on 16th November 2018
Abstract
The metal–organic framework Ni(bdc)(ted)0.5 is a promising material for simultaneous capture of harmful gases such as SO2 and CO2. We found that SO2 performs much better than CO2 during adsorption, and the lack of physical insight was clarified through detailed analyses of the electronic structures obtained from density functional theory calculations. Our results showed that strong interactions of the d band of Ni atoms with the valence states (2n, 3n, and 4n) of SO2 but almost not with those of CO2 are the main reasons. Our finding is useful for the rational design of new metal–organic frameworks with suitable interactions for the simultaneous capture of not only SO2 and CO2 but also other gases.
I. Introduction
CO2 is a greenhouse gas leading to global warming, and SO2 is the primary source of acid rain.1,2 With increasing consumption of fossil fuel energies, emissions of SO2 and CO2 are becoming more serious. The removal of these gases is, therefore, an active research area attracting much attention from scientists. Among the proposed materials and techniques,3–6 metal–organic frameworks (MOFs) are the most promising candidates that satisfy the practical requirements of high stability, high gas uptake capacity, low energy of gas desorption, and low cost.
Due to exceptionally high surface area, tunable porous crystals, and structural versatility, MOFs exhibit excellent ability for the capture and selective separation of a wide range of gases based on adsorption.7 Particularly, MOF-200 and MOF-210 have shown the highest CO2 uptake so far up to more than 70 wt%.8 Several studies have also been devoted to the study of SO2 capture using MOFs.6,9–14 Because of the co-existence of SO2 and CO2 in the same practical environment and the need for the removal of these gases to mitigate their influences on the climate, the simultaneous capture of the gases within the same MOF is particularly important.6,15–18 Recently, Tan and coworkers found that Ni(bdc)(ted)0.5 (bdc = benzenedicarboxylate, ted = triethylenediamine) can adsorb a significant amount of SO2 (9.97 mmol g−1) and simultaneously exhibit remarkable uptake of CO2 at low pressure and ambient temperature.13,19,20 Remarkably, SO2 performs much better than CO2 during co-adsorption by Ni(bdc)(ted)0.5 as SO2 can replace pre-adsorbed CO2;13 however, the physical insights into this phenomenon remain unexplained.
Here, we used van der Waals-corrected density functional theory,21–24 which has been successfully applied in previous studies,25–27 to reveal the electronic nature of MOF-gas interactions. Thus, our study is necessary, and the current findings are useful for the design of new MOFs for simultaneous adsorption of other gases.
II. Computational details
Density functional theory calculations were performed using the Vienna Ab Initio Simulation Package. The exchange and correlation functionals21,28 including the van der Waals dispersion corrections, vdW-DF,22,29 were used in this study. The electron-ion interactions were described by the projector augmented-wave method23,24 with a plane wave cut-off of 700 eV. The integration over the Brillouin zone was calculated using the Monkhorst–Pack scheme30 with k-point sampling of 5 × 5 × 5 for geometry optimization and electronic structure calculations. The size and shape of the unit cell of Ni(bdc)(ted)0.5 were fully optimized; it exhibited a tetragonal structure with cell parameters of a = b = 11.15 Å and c = 9.53 Å (Fig. 1).13 Spin polarization was performed for geometry optimization and total energy calculation.
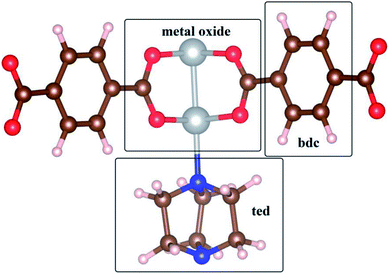 |
| Fig. 1 The unit cell of Ni(bdc)(ted)0.5; C (brown), O (red), H (white), N (blue), and Ni (silver). | |
The adsorption energy Ead is defined as follows:
|
Ead = EMOF+SO2/CO2 − EMOF − ESO2/CO2.
| (1) |
Here,
EMOF+SO2/CO2,
EMOF,
ESO2, and
ECO2 are the total energies of the MOF + gas system, MOF Ni(bdc)(ted)
0.5, isolated SO
2, and isolated CO
2, respectively.
The charge density difference31–33 was calculated for the most stable configurations of the adsorbed SO2 and CO2 using the following formula:
|
Δρ = ρMOF+SO2/CO2 − ρMOF − ρSO2/CO2.
| (2) |
Here,
ρMOF+SO2/CO2 and
ρMOF are the charge densities of the MOF + gas system and MOF Ni(bdc)(ted)
0.5, respectively;
ρSO2 and
ρCO2 are the charge densities of the single molecule SO
2 and CO
2, respectively. Here, the charge density of the MOF and the gas was calculated from the corresponding structures obtained from the MOF + gas system by removing either the gas or the MOF, respectively.
The partial point charges for each atom of SO2 and CO2 and MOF were estimated using the Bader charge partition method.34,35
III. Results and discussion
We label the unit cell surface of Ni(bdc)(ted)0.5 by three main regions: bdc, ted, and metal oxide (Fig. 1). For single gas adsorption, we load each molecule of SO2 and CO2 to various positions on the surface of Ni(bdc)(ted)0.5. We then optimize the geometry and calculate the adsorption energy of SO2 and CO2. We select the most stable configuration of SO2 and CO2 in each surface region based on the obtained adsorption energy, as presented in Fig. 2, for further analyses. This figure also exhibits the bond distances between SO2 and CO2 with the nearest atoms of Ni(bdc)(ted)0.5. To roughly understand the nature of MOF-gas interaction, we analyze the bond distance between the gas and the MOF. In this study, the atomic radii are defined through the Wigner–Seitz radius, which are 0.37, 0.741, 0.783, 0.863, 0.953, and 1.286 Å for H, O, N, C, S, and Ni, respectively. Generally, the length of a covalent bond between two atoms equals the sum of the radii of the two atoms. If there are covalent bonds, the standard bond lengths of C–S, O–H, S–O, C–C, and C–O between the gas and MOF, as shown in Fig. 2, are expected to be 1.816, 1.111, 1.694, 1.726, and 1.602 Å, respectively. However, the nearest bond distances (Fig. 2) are much longer than these standard covalent bond lengths. Therefore, there is physical interaction rather than a covalent bond between the gases and MOF.
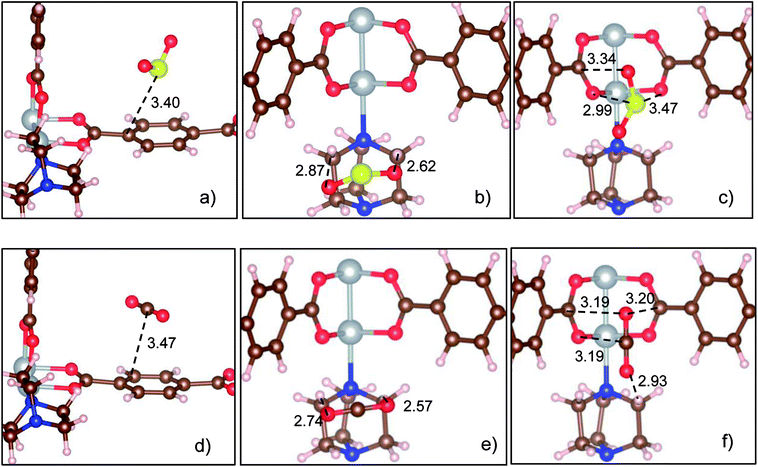 |
| Fig. 2 Stable adsorption configurations of SO2 (upper panel) and CO2 (lower panel) on bdc (a and d), ted (b and e), and metal oxide (c and f) of Ni(bdc)(ted)0.5; C (brown), O (red), H (white), S (yellow), N (blue), and Ni (silver). The bond distance is in angstroms. | |
Table 1 shows that the adsorption strengths of both gases follow the order bdc < ted < metal oxide, which agrees with the results for SO2 in a previous study.13 The adsorption energy of SO2 is significantly lower than that of CO2 for each adsorption site, indicating that SO2 adsorbs more strongly than CO2. Furthermore, while the adsorption energies of CO2 on the sites are not much different, those of SO2 vary largely, especially on metal oxide, which implies that the metal center of Ni(bdc)(ted)0.5 has a more significant influence on SO2 than on CO2.13 A stronger binding energy of SO2 compared with that of CO2 at the metal site is also observed for other MOFs;20,36,37 the binding energy of SO2 and CO2 at the metal oxide of Ni(bdc)(ted)0.5 is found to be in the same order with that of Mg, Ni, and Co-MOF-74.20 However, no comprehensive explanations based on the analysis of electronic structures have been provided for the current MOF system. Besides, vibrational energy also influences the stability of the adsorbed gases. Therefore, we calculate the vibrational frequency (ω) and the vibrational energy (1/2 hω) for the adsorbed SO2 and CO2. The frequencies of the three strongest vibrational modes on the metal oxide are found to be 1240, 1071, and 505 cm−1 for SO2 and 2432, 1366, and 610 cm−1 for CO2. For the other adsorption sites, the frequencies fluctuate around these values by less than 50 cm−1. The obtained frequencies agree well with the experimental data.13,20,38 Sequentially, the calculated vibrational energies are 0.154, 0.133, and 0.063 eV for SO2 and 0.301, 0.169, and 0.076 eV for CO2. The vibrational energy of the strongest SO2 mode (0.154 eV) is significantly smaller than the adsorption energy difference (0.541 eV) between SO2 on metal oxide and ted, whereas that of CO2 (0.301 eV) is much higher than its adsorption energy difference (0.073 eV) between these sites. Therefore, fluctuation cannot cause transition between the most favorable adsorption sites of SO2, but it exhibits effects on CO2. This result implies that SO2 is much more stable compared to CO2 toward fluctuation.
Table 1 Adsorption Energy (eV) of SO2 and CO2 in Ni(bdc)(ted)0.5
Adsorption site |
bdc |
ted |
Metal oxide |
SO2 |
−0.351 |
−0.469 |
−1.010 |
CO2 |
−0.213 |
−0.307 |
−0.380 |
Next, we systematically clarify the electronic structure of MOF – gas interaction. The charge density difference between SO2 and CO2 on the metal oxide is presented in Fig. 3a and b, respectively. The charge density difference is calculated by using eqn (2). The charge donation from SO2 and CO2 to MOF is depicted by the charge depletion cloud, whereas the back-donation from MOF to SO2 and CO2 is indicated by the charge accumulation on SO2 and CO2. A large extent of charge redistribution is found on Ni atoms in the case of SO2 but not for CO2, which is consistent with the observations from adsorption energy. The positive sign of the total point charge for the adsorbed SO2 and CO2 (Table 2) implies that back-donation is stronger than donation. The charge gains of the gases are mainly distributed on the oxygen atoms, and the charge gain of SO2 is greater than that of CO2 for all adsorption sites.
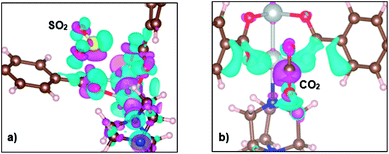 |
| Fig. 3 The charge density difference of the most stable configuration of SO2 (a) and CO2 (b) found on the metal oxide. Pink and cyan clouds denote the charge loss and gain, respectively. | |
Table 2 Bader charges (e−) of the adsorbed SO2 and CO2 and the MOF compared to those of the isolated ones, where (−) represents the charge loss and (+) represents the charge gain
Adsorption site |
bdc |
ted |
Metal oxide |
S |
−0.013 |
+0.002 |
−0.032 |
2O |
+0.055 |
+0.108 |
+0.102 |
SO2 |
+0.042 |
+0.110 |
+0.070 |
20H |
−0.032 |
−0.094 |
−0.005 |
22C |
−0.005 |
+0.011 |
−0.064 |
2N |
0.000 |
+0.010 |
−0.010 |
8O |
−0.005 |
−0.032 |
+ 0.068 |
2Ni |
0.000 |
−0.005 |
−0.058 |
MOF |
−0.042 |
−0.110 |
−0.070 |
C |
−0.019 |
−0.048 |
−0.066 |
2O |
+0.036 |
+0.055 |
+0.087 |
CO2 |
+0.017 |
+0.008 |
+0.021 |
20H |
−0.019 |
−0.022 |
−0.030 |
22C |
+0.008 |
+0.009 |
+0.005 |
2N |
−0.002 |
0.000 |
0.000 |
8O |
−0.003 |
+0.004 |
+0.001 |
2Ni |
0.000 |
0.000 |
+0.003 |
MOF |
−0.017 |
−0.008 |
−0.021 |
The donation of charge occurs at MOF hydrogen atoms for both SO2 and CO2 adsorptions and also at MOF C atoms for CO2 adsorption for all the adsorption sites. The other MOF atoms gain or donate charge or remain neutral depending on the local sites.
Fig. 4a shows substantial expansion in the width and significant variation in the peak height of all the valence states (2σ/1π/3σ, 2n, 3n, and 4n) of SO2. Scheme S1 and S2 in the ESI† depict the molecular orbital diagrams of SO2 and CO2. On the basis of these schemes, we located the peaks of electronic density of the states of SO2 and CO2. The modification of 2σ/1π/3σ states is mainly ascribed to (Fig. 5a) the overlap between DOS of SO2 and that of MOF oxygen atoms, whereas the change in the 2n/3n/4n states is ascribed to the interaction of DOS of SO2 with that of MOF oxygen atoms and the d band of Ni atoms.
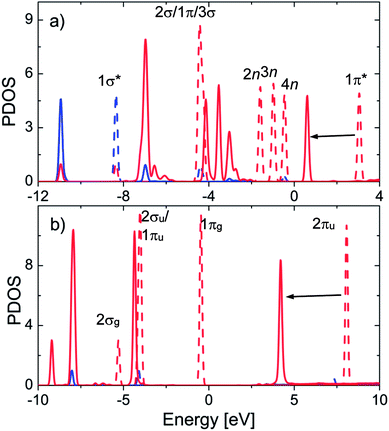 |
| Fig. 4 The partial DOS of the isolated (dashed line) and adsorbed (solid line) gases SO2 (a) and CO2 (b) on the metal oxide. The s and p orbitals are presented in blue and red, respectively. | |
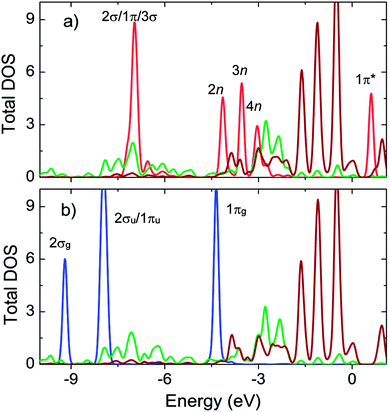 |
| Fig. 5 The total DOS of the adsorbed SO2 (red) and the adsorbed CO2 (blue) on the metal oxide. The total DOS of the MOF's oxygen atoms and the d band of the Ni atoms are presented in green and brown, respectively. | |
Fig. 4b shows that the DOS peaks of CO2 shifted to a lower energy level and the intensity of 2σu/1πu/1πg/2πu states decreased upon adsorption, which was closely correlated to the overlap of these orbitals with those of the MOF oxygen atoms (Fig. 5b). The shift in the value of the energy level is about 2.5 eV for SO2 and 4.0 eV for CO2. Noticeably, there is no significant interaction between the states of CO2 and the d band of the Ni atoms, which is entirely different from the case of SO2. We also provide a detailed analysis of the interaction between SO2 and CO2 with bdc and ted in the following section.
For SO2 on bdc, Fig. 6a shows that the peaks of DOS of SO2 exhibit the same shape as that of isolated SO2 with weaker interactions between SO2 and bdc compared with those observed on other adsorption sites. The height of the peak of 2σ/1π/3σ states is enhanced, whereas that of the 4n state is slightly reduced because of the overlap of these states with DOS of the MOF atoms (Fig. 7a). For SO2 on ted, as presented in Fig. 6b, the width and height of 2σ/1π/3σ and 2n/3n states are modified owing to the interactions with the hydrogen and carbon atoms of ted (Fig. 7b). In particular, overlaps occur between the 2σ/1π/3σ/2n states and the states of the oxygen atoms and between the 3n state and the states of the MOF carbon atoms and the d band of Ni atoms. Moreover, the 1π* peak shifts to the Fermi level due to the overlap with the d band of Ni atoms; therefore, SO2 gains a significantly greater charge on ted than on bdc and metal oxide (Table 2).
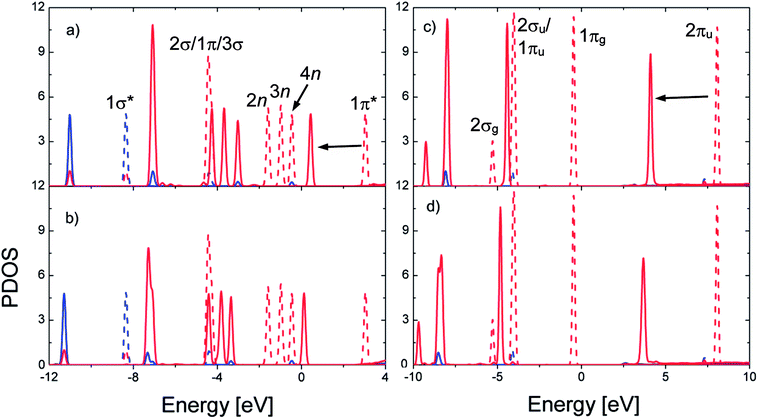 |
| Fig. 6 The partial DOS of the adsorbed gases (solid line) and the isolated gases (dotted line) for SO2 (left side) and CO2 (right side): on bdc (a and c), on ted (b and d). The s and p states are presented in blue and red, respectively. | |
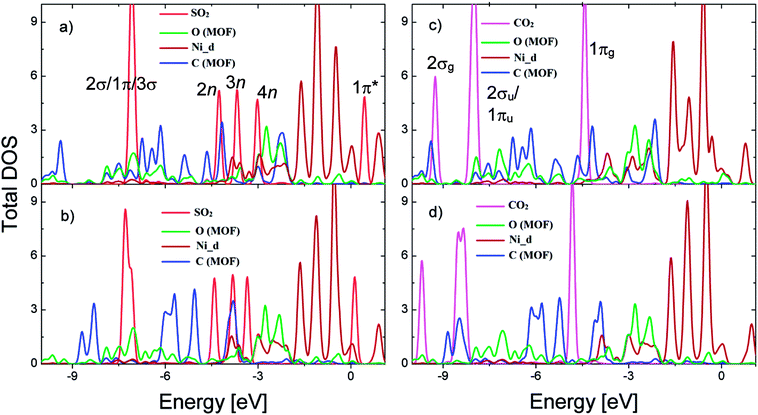 |
| Fig. 7 The total DOS of adsorbed SO2 (left side), adsorbed CO2 (right side), and atoms of Ni(bdc)(ted)0.5: on bdc (a and c), ted (b and d). The d band of the Ni atoms strongly interacts with the valence states of SO2 but almost does not interact with the valence states of CO2 for all the adsorption sites. | |
For CO2, the position of the peaks shifts to a lower energy, and the height of the peaks is reduced upon adsorption (Fig. 6c and d). However, the shape of the peaks is almost unchanged except for the modification at the 2σu/1πu states for the adsorption on ted (Fig. 6d) due to the overlap of these states with the states of ted's carbon atoms (Fig. 7d). For CO2 on all the adsorption sites, the interaction with MOF is accomplished through the overlaps of DOS of CO2 with DOS of the MOF C and O atoms. Noticeably, there is no significant interaction between CO2 and the Ni atoms for all the adsorption sites, which is different from that observed for SO2.
For the simultaneous adsorption of SO2 and CO2 in Ni(bdc)(ted)0.5, we found their most favorable adsorption configurations (Fig. 8). The adsorption energies are −0.33, −0.65, and −0.41 eV for configurations (a), (b), and (c), respectively. Therefore, the configuration (b) is the most favorable one. Fig. 9 shows that the DOS of SO2 and CO2 show similar characteristics to those of single gas adsorption. From the above analyses, we can see that while both SO2 and CO2 interact with the MOF C and O atoms, only SO2 significantly interacts with Ni atoms. Thus, we can state that the participation of the d band to different extents can be the reason for competition of SO2 and CO2 in Ni(bdc)(ted)0.5. Our findings support the previous experimental results about the crucial role of metal centers.13,25 This manuscript is devoted to providing an explanation for the experimental observation regarding the co-adsorption of SO2 and CO2 in Ni(bdc)(ted)0.5. Therefore, the study with different organic linkers, metal centers, and gas mixtures is beyond the scope of the present research and will be addressed39–41 in upcoming studies.
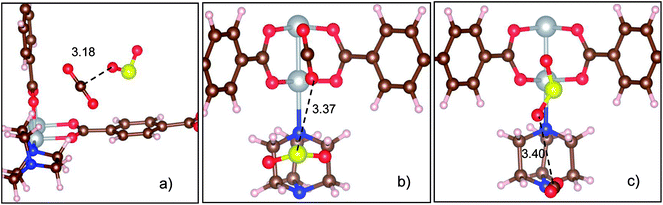 |
| Fig. 8 The most stable configurations for co-adsorption of SO2 and CO2. The co-adsorption energies are −0.33, −0.65, and −0.41 eV for (a), (b), and (c), respectively; C (brown), O (red), H (white), S (yellow), N (blue), and Ni (silver). The bond distance is in angstroms. | |
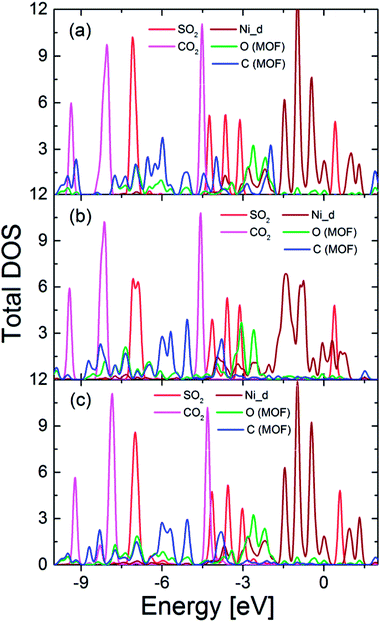 |
| Fig. 9 The total DOS of adsorbed SO2, adsorbed CO2, and atoms of Ni(bdc)(ted)0.5 for co-adsorption of SO2 and CO2; (a), (b), and (c) correspond to the configurations presented in Fig. 8. | |
IV. Conclusion
In conclusion, DOS of both gases homogeneously shift to lower energy levels upon adsorption. The deformations are more profound in the DOS of SO2 than that of CO2, including the downshifting of the anti-bonding 1π* state of SO2 to the Fermi level. The charge gain of SO2 is higher than that of CO2, which is consistent with the adsorption energy trend. Notably, the adsorption energy of SO2 is significantly greater on metal oxide than on other adsorption sites, which is not observed in the case of CO2, implying that the effect of Ni atoms on the adsorption of CO2 is not much significant. This is because the d band of Ni atoms strongly interacts with the electronic states of SO2 but does not interact with the electronic states of CO2. The critical role of the metal center of MOFs has been addressed in the literature. Nevertheless, this study shows that this role cannot be fulfilled if the d band of the metal center does not interact with the electronic states of the adsorbed gases. We also found that increased overlap in DOS causes more charge sharing and thus stronger binding of the gas to MOF. The specific interaction between SO2 and Ni2+ is unexpected since the metal ions in secondary building units of MOFs such as M(bdc)(ted)0.5 (M is a transition metal) usually do not interact strongly with guest molecules because they are fully saturated and screened. Thus, this study presents an interesting finding, which explains the experimental result.
Conflicts of interest
There are no conflicts of interest to declare.
Acknowledgements
This research was funded by Ho Chi Minh City University of Technology – VNU-HCM under grant number T-KHUD-2017-34. We acknowledge the usage of the computer time and software granted by the Institute of Physical Chemistry of Romanian Academy, Bucharest (HPC infrastructure developed under the projects Capacities 84 Cp/I of 15.09.2007 and INFRANANOCHEM 19/01.03.2009).
References
- T. R. Karl and K. E. Trenberth, Modern Global Climate Change, Science, 2003, 302, 1719–1723 CrossRef CAS PubMed.
- G. E. Likens and F. H. Bormann, Acid Rain: A Serious Regional Environmental Problem, Science, 1974, 184, 1176–1179 CrossRef CAS PubMed.
- D. Y. C. Leung, G. Caramanna and M. M. Maroto-Valer, An Overview of Current Status of Carbon Dioxide Capture and Storage Technologies, Renewable Sustainable Energy Rev., 2014, 39, 426–443 CrossRef CAS.
- K. Sumida, D. L. Rogow, J. A. Mason, T. M. McDonald, E. D. Bloch, Z. R. Herm, T.-H. Bae and J. R. Long, Carbon Dioxide Capture in Metal-Organic Frameworks, Chem. Rev., 2012, 112, 724–781 CrossRef CAS PubMed.
- D. Britt, D. Tranchemontagne and O. M. Yaghi, Metal-Organic Frameworks with High Capacity and Selectivity for Harmful Gases, PNAS, 2008, 105, 11623–11627 CrossRef CAS PubMed.
- S. Yang, J. Sun, A. J. Ramirez-Cuesta, S. K. Callear, W. I. F. David, D. P. Anderson, R. Newby, A. J. Blake, J. E. Parker, C. C. Tang and M. Schröder, Selectivity and Direct Visualization of Carbon Dioxide and Sulfur Dioxide in a Decorated Porous Host, Nature Chem., 2012, 4, 887–894 CrossRef CAS PubMed.
- J.-R. Li, R. J. Kuppler and H.-C. Zhou, Selective Gas Adsorption and Seperation in Metal-Organic Frameworks, Chem. Soc. Rev., 2009, 38, 1477–1504 RSC.
- H. Furukawa, N. Ko, Y. B. Go, N. Aratani, S. B. Choi, E. Choi, A. O. Yazaydin, R. Q. Snurr, M. O'Keeffe, J. Kim and O. M. Yaghi, Ultrahigh Porosity in Metal-Organic Frameworks, Science, 2010, 329, 424–428 CrossRef CAS PubMed.
- C. Wuang, H. Liu, X. Z. Li, J. Shi, G. Ouyang, M. Peng, C. Jiang and H. N. Cui, A new concept of Desulfurization: The electrochemically Driven and Green conversion of SO2 to NaHSO4 in aqueous solution, Environ. Sci. Technol., 2008, 42, 8585–8590 CrossRef.
- G. Hu, Z. Sun and H. Gao, Novel process of Simultaneous Removal of SO2 and NO2 by Sodium Humate Solution, Environ. Sci. Technol., 2010, 44, 6712–6717 CrossRef CAS PubMed.
- X. Y. Liu, J. M. Zhang, K. W. Xu and V. Ji, Improving SO2 gas sensing properties of graphene by introducing dopant and defect: A first-principles study, Appl. Surf. Sci., 2014, 313, 405–410 CrossRef CAS.
- X.-D. Song, S. Wang, C. Hao and J.-S. Qiu, Investigation of SO2 Gas Adsorption in Metal–Organic Frameworks by Molecular Simulation, Inorg. Chem. Commun., 2014, 46, 277–281 CrossRef CAS.
- K. Tan, P. Canepa, Q. Gong, J. Liu, D. H. Johnson, A. Dyevoich, P. K. Thallapally, T. Thonhauser, J. Li and Y. J. Chabal, Mechanism of Preferential Adsorption of SO2 into Two Microporous Paddle Wheel Frameworks M(bdc)(ted)0.5, Chem. Mater., 2013, 25, 4653–4662 CrossRef CAS.
- Z. Liang, M. Marshall and A. L. Chaffee, CO2 adsorption selectivity and water tolerance of pillared-layer metal organic frameworks, Microporous Mesoporous Mater., 2010, 132, 305–310 CrossRef CAS.
- L. Ding and A. O. Yazaydin, The Effect of SO2 and CO2 Capture in Zeolitic Imidazolate Frameworks, Phys. Chem. Chem. Phys., 2013, 15, 11856–11861 RSC.
- J. Yu, Y. Ma and P. B. Balbuena, Evaluation of the Impact of H2O, O2, and SO2 on Postcombustion CO2 Capture in Metal-Organic Frameworks, Langmuir, 2012, 28, 8064–8071 CrossRef CAS PubMed.
- H.-J. Ryu, J. R. Grace and C. J. Lim, Simultaneous CO2/SO2 Capture Characteristics of Three Limestones in a Fluidized-Bed Reactor, Energy Fuels, 2006, 20, 1621–1628 CrossRef CAS.
- T. T. T. Huong, P. N. Thanh, N. T. X. Huynh and D. N. Son, Metal – Organic Frameworks: State-of-the-art Material for Gas Capture and Storage, VNU J. Sci.: Math. Phys., 2016, 32, 67–85 Search PubMed.
- B. Arstad, H. Fjellvåg, K. O. Kongshaug, O. Swang and R. Blom, Amine Functionalised Metal Organic Frameworks (MOFs) as Adsorbents for Carbon Dioxide, Adsorption, 2008, 14, 755–762 CrossRef CAS.
- K. Tan, S. Zuluaga, Q. Gong, Y. Gao, N. Nijem, J. Li, T. Thonhauser and Y. J. Chabal, Competitive Coadsorption of CO2 with H2O, NH3, SO2, NO, NO2, N2, O2, and CH4 in M-MOF-74 (M = Mg, Co, Ni): The Role of Hydrogen Bonding, Chem. Mater., 2015, 27, 2203–2217 CrossRef CAS.
- J. P. Perdew, K. Burke and M. Ernzerhof, Generalized Gradient Approximation Made Simple, Phys. Rev. Lett., 1996, 77, 3865–3868 CrossRef CAS PubMed.
- M. Dion, H. Rydberg, E. Schroder, D. C. Langreth and B. I. Lundqvist, Van der Waals Density Functional for General Geometries, Phys. Rev. Lett., 2004, 92(1–4), 246401 CrossRef CAS PubMed.
- P. E. Blöchl, Projector Augmented-Wave Method, Phys. Rev. B: Condens. Matter Mater. Phys., 1994, 50, 17953–17979 CrossRef.
- G. Kresse and J. Joubert, From Ultrasoft Pseudopotentials to the Projector Augmented-Wave Method, Phys. Rev. B: Condens. Matter Mater. Phys., 1999, 59, 1758–1775 CrossRef CAS.
- J. Park, H. Kim, S. S. Han and Y. Jung, Tuning Metal−Organic Frameworks with Open-Metal Sites and Its Origin for Enhancing CO2 Affinity by Metal Substitution, J. Phys. Chem. Lett., 2012, 3, 826–829 CrossRef CAS PubMed.
- H. Wu, J. M. Simmons, G. Srinivas, W. Zhou and T. Yildirim, Adsorption Sites and Binding Nature of CO2 in Prototypical Metal−Organic Frameworks: A Combined Neutron Diffraction and First-Principles Study, J. Phys. Chem. Lett., 2010, 1, 1946–1951 CrossRef CAS.
- H. S. Koh, M. K. Rana, J. Hwang and D. J. Siegel, Thermodynamic screening of metal-substituted MOFs for carbon capture, Phys. Chem. Chem. Phys., 2013, 15, 4573–4581 RSC.
- J. P. Perdew, J. A. Chevary, S. H. Vosko, K. A. Jackson, M. R. Pederson, D. J. Singh and C. Fiolhais, Atoms, Molecules, Solids, and Surfaces: Applications of the Generalized Gradient Approximation for Exchange and Correlation, Phys. Rev. B: Condens. Matter Mater. Phys., 1992, 46, 6671–6687 CrossRef CAS.
- D. C. Langreth, B. I. Lundqvist, S. D. Chakarova-Käck, V. R. Cooper, M. Dion, P. Hyldgaard, A. Kelkkanen, J. Kleis, L. Kong, S. Li, P. G. Moses, E. Murray, A. Puzder, H. Rydberg, E. Schröder and T. Thonhauser, A Density Functional for Sparse Matter, J. Phys.: Condens. Matter, 2009, 21(1–15), 084201 Search PubMed.
- H. J. Monkhorst and J. D. Pack, Special Points for Brillouin-Zone Integrations, Phys. Rev. B: Condens. Matter Mater. Phys., 1976, 13, 5188–5192 CrossRef.
- G. Rojas, S. Simpson, X. Chen, D. A. Kunkel, J. Nitz, J. Xiao, P. A. Dowben, E. Zurek and A. Enders, Surface State Engineering of Molecule–molecule Interactions, Phys. Chem. Chem. Phys., 2012, 14, 4971–4976 RSC.
- W. G. Schmidt, K. Seino, M. Preuss, A. Hermann, F. Ortmann and F. Bechstedt, Organic Molecule Adsorption on Solid Surfaces: Chemical Bonding, Mutual Polarisation and Dispersion Interaction, Appl. Phys. A, 2006, 85, 387–397 CrossRef CAS.
- D. N. Son, O. K. Le, M. T. Hiep and V. Chihaia, Magnetic
anisotropy of ultrathin Pd4Co(111) film by first-principles calculations, J. Sci.: Adv. Mat. Dev., 2018, 3, 243–253 Search PubMed.
- G. Henkelman, A. Arnaldsson and H. Jonsson, A Fast and Robust Algorithm for Bader Decomposition of Charge Density, Comput. Mater. Sci., 2006, 36, 354–360 CrossRef.
- N. T. X. Huynh, O. M. Na, V. Chihaia and D. N. Son, A computational approach towards understanding hydrogen gas adsorption in Co-MIL-88A, RSC Adv., 2017, 7, 39583–39593 RSC.
- K. Yu, K. Kiesling and J. R. Schmidt, Trace Flue Gas Contaminants Poison Coordinatively Unsaturated Metal- Organic Frameworks: Implications for CO2 Adsorption and Separation, J. Phys. Chem. C, 2012, 116, 20480–20488 CrossRef CAS.
- L. Ding and A. Ö. Yazaydin, How Well Do Metal–Organic Frameworks Tolerate Flue Gas Impurities?, J. Phys. Chem. C, 2012, 116, 22987–22991 CrossRef CAS.
- V. Wathelet, B. Champagne, D. H. Mosley, E. A. Perpète and J. M. André, Vibrational Frequencies of H2O and CO2 from Car-Parrinello Molecular Dynamics, J. Mol. Struct.: THEOCHEM, 1998, 425, 95–100 CrossRef CAS.
- L. Du, Z. Lu, L. Xu and J. Zhang, A new mfj-type metal–organic framework constructed from a methoxyl derived V-shaped ligand and its H2, CO2 and CH4 adsorption properties, RSC Adv., 2017, 7, 21268–21272 RSC.
- C. Altintas and S. Keskin, Molecular simulations of MOF membranes for separation of ethane/ethene and ethane/methane mixtures, RSC Adv., 2017, 7, 52283–52295 RSC.
- F. Zhou, J. Zhou, X. Gao, C. Kong and L. Chen, Facile synthesis of MOFs with uncoordinated carboxyl groups for selective CO2 capture via postsynthetic covalent modification, RSC Adv., 2017, 7, 3713–3719 RSC.
Footnote |
† Electronic supplementary information (ESI) available: Molecular orbital diagrams for SO2 and CO2. See DOI: 10.1039/c8ra07919f |
|
This journal is © The Royal Society of Chemistry 2018 |