DOI:
10.1039/C8RA07294A
(Paper)
RSC Adv., 2018,
8, 36445-36452
Molecular keypad controlled circuit for Ce(III) and NO3− ions recognition by μw synthesized silicon-embedded organic luminescent sensor†
Received
1st September 2018
, Accepted 23rd October 2018
First published on 29th October 2018
Introduction
Ion recognition studies work upon the principle of fluctuation in absorption or emission maxima upon interactions of an ion(s) with an ionophore. The role of chemical sensing is the exploration of its applications in different disciplines of chemistry and are not limited to, clinical biology and environmental science. Among the numerous kinds of chemical sensors, luminescence-based chemosensors display a set of advantages, since they have high sensitivity (single ion detection), versatility (bio-compatibility), low cost, easy application and good resolution, which are typical of photoluminescence spectroscopy.1 A chemosensor has two basic units (a) the receptor unit for selective binding and (b) the signal unit for reporting output through change in the optical properties (Fig. 1). The detection of target analyte is achieved by the molecular recognition followed by signal transduction.2 Among all type of compounds used as fluorescent chemosensors, organic luminophores are most widely studied due to their rich chemical structures, easy chemical modification and highly fluorescent quantum yield.3,4 The creation of luminophores through clean reaction methodologies have generated immense scientific interest in exploring copper(I)-catalyzed alkyne-azide cycloaddition (CuAAC).
 |
| Fig. 1 Representation of a fluorescent ion luminescent sensor. | |
There have been numerous citations pertaining to the transition metal ion sensing by using 1,2,3-triazoles but this is the first report (to the best of our knowledge) citing the use of ‘Click Silylation’ product for Ce(III) ion analysis.5–7 ‘Click Silylation’ technique introduced a terminal siloxy group into the luminiphore bridged by 1,2,3-triazole linker.8–15 The naphthyl moiety (acting as active unit) linked via 1,2,3-triazole (spacer) has been explored as an ionic fluorophore can find potential application in bio-imaging technique owing to its specific excitation and emission UV values, unaltered by any interference from other metal ions.7,16,17 The triazole linker creates an effective link for host–guest binding yielding fluorescence quenching or enhancement, accompanied by batho- or hypso- chromic shift.5
Though cerium is classified as rare earth metal, it is still abundant in crust (66 μg g−1) and has been put into use in metallurgy, vehicle catalytic converters, ceramic materials and as heterogenous catalyst. In contrary, Ce(III) ions hampers biological functioning by altering the immune systems and functioning of many essential body organs. The prolonged exposure to the moist areas rich in Ce(III) ions can cause lung embolisms and liver damage.18–21 Thus, there is an urgent need of selective sensor for analysis and recovery of Ce(III) ions in water, soil and industrial samples.22 The developments in cation sensing is interlinked to anion sensing and are driven by their major role in biology, industry and environment pollution. Anion sensing works on the displacement module where an anion binds to receptor by replacing an indicator, causing perturbations in absorption spectral values.23–25 The Schiff base framework acts as the backbone to assists binding of anions that may result into the significant visual changes after complexation.26–29
The mimicking of sensing behaviour can be processed as ‘0’ and ‘1’ input values in case of logic gates that is instantly processed by specific output changes as signals of molecular gates.15,30–33 So, far no Ce(III) ion-ensemble sensing nitrate ions have been reported to the best of our knowledge. Thus, we herein report a new silicon capped 1,2,3-triazole linked naphthyl luminescent sensor (NTS) with regulated ON–OFF–ON switch module towards Ce(III) and NO3− ions. This type of behaviour has been mapped with the working of molecular switches that can work with an appropriate combination of chemical inputs.
Experiment
Materials and methods
All the syntheses were carried out under inert dry nitrogen atmosphere using vacuum glass line. The organic solvents were dried according to standard procedures.34 2-Hydroxy-1-naphthaldehyde (Aldrich), 4-chlorobenzenamine (Aldrich), bromotris (triphenylphosphine) copper(I) [CuBr(PPh3)3] (Aldrich), tetrahydrofuran (THF) (CDH), triethylamine (Et3N) (SDFCL), triethanolamine (CDH), KOH (CDH), toluene (SDFCL), DMF (FINAR) were used as received. 3-Azidopropyltriethoxysilane (AzPTES) was synthesized by known procedure from literature.35,36 Infrared spectrum was obtained neat on a Thermo Scientific Fischer spectrometer. CHN analysis was obtained on Perkin Elmer Model 2400 CHNS elemental analyzer and Thermo Scientific Flash 2000 organic elemental analyzer. Multinuclear NMR (1H, 13C) spectra were recorded on a Bruker advance II 400 and on a Jeol (AL 300 MHz) spectrometer using CDCl3 as internal reference and chemical shifts were reported relative to tetramethylsilane. Melting points were uncorrected and measured in a Mel Temp II device using sealed capillaries.
Synthesis of luminescent sensor 5
The entity 2 (Scheme 1) was synthesised from 2-hydroxy-1-naphthaldehyde 1 by a known procedure.36–39 The entity 3 was synthesized by microwave upon reacting equimolar quantity of 4-chloroaniline (0.18 g, 1.4 mmol) with 2 (0.3 g, 1.4 mmol) using 1 ml of ethanol as solvent at 60 °C. The yellow coloured solid product 3 was obtained within five minutes. The Schiff base alkyne 3 (0.38 g, 1.2 mmol) was treated with 3-azidopropyltriethoxysilane (0.29 g, 1.2 mmol) in equimolar ratio using THF
:
TEA 2
:
1 (v/v) (optimized as entry 2 in Table 1) as solvent and [CuBr(PPh3)3] as catalyst (0.01 mmol/alkyne function) in microwave at 60 °C for 15 minutes. The 1,4 triazole linked naphthyl terminated triethoxysilane 4 was extracted by solvent evaporation after catalyst filtration as stereospecific product.40 The silane 4 (0.63 g, 1.1 mmol) was processed by trans-esterification reaction with triethanolamine (0.18 g, 1.2 mmol) using toluene as solvent and anhydrous powdered KOH as catalyst to yield silatrane 5.
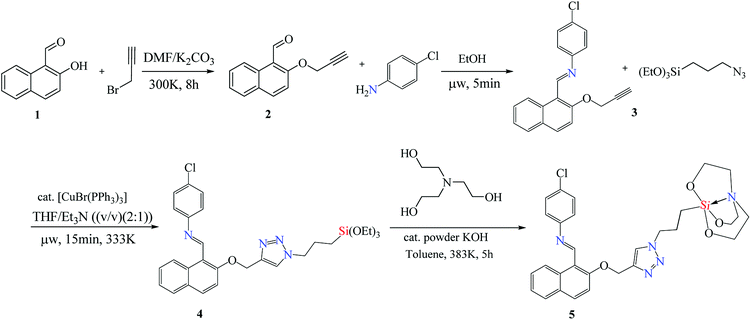 |
| Scheme 1 Reaction sequence followed for the synthesis of luminescent sensor NTS (5). | |
Table 1 Optimization of reaction conditions for μw assisted synthesis of triazole
Entry |
Solvent ratio |
Reaction duration (μw) |
Yielda (%) |
Determined by 1H NMR analysis of isolation of crude sample. |
1 |
THF : TEA 1 : 1 (v/v) |
15 min |
81 |
2 |
THF : TEA 2 : 1 (v/v) |
15 min |
93 |
3 |
THF : TEA 1 : 2 (v/v) |
15 min |
67 |
4 |
THF : TEA 3 : 1 (v/v) |
15 min |
74 |
5 |
THF : TEA 1 : 1 (v/v) |
30 min |
82 |
6 |
THF : TEA 2 : 1 (v/v) |
30 min |
93 |
7 |
THF : TEA 1 : 2 (v/v) |
30 min |
72 |
8 |
THF : TEA 3 : 1 (v/v) |
30 min |
79 |
Synthesis of 3. Yield: 84%; mp: 121–122 °C; anal. calcd for C20H14ClNO (%): C, 75.12; H, 4.41; N, 4.38; found (%): C, 74.68; H, 4.51; N, 4.02. IR (neat, cm−1): 2920, 2943, 2986, 2828, 2041, 1474, 1397, 1113, 947, 787; 1H NMR (300 MHz, CDCl3) δ = 9.48 (d, J = 8.7 Hz, 1H), 9.15 (s, 1H), 7.87 (d, J = 9.1 Hz, 1H), 7.71 (d, J = 8.0 Hz, 1H), 7.51 (t, J = 7.3 Hz, 1H), 7.36 (d, J = 7.5 Hz, 1H), 7.31 (d, J = 4.8 Hz, 2H), 7.16 (t, J = 6.4 Hz, 2H), 4.83 (d, J = 2.3 Hz, 2H), 3.64 (s, 1H), 2.42 (t, J = 4.1 Hz, 1H).
Synthesis of 4. Yield 93%; viscous brown oil; anal. calcd for C29H35ClN4O4Si (%): C, 61.41; H, 6.22; N, 9.88; found (%): C, 61.38; H, 6.41; N, 9.25; IR (neat, cm−1): 2931, 2867, 2831, 1645, 1606, 1576, 1361, 1301, 1165, 1077, 1023, 887, 831, 797, 691; 1H NMR (300 MHz, CDCl3) δ = 9.45 (d, J = 8.7 Hz, 1H), 9.10 (s, 1H), 7.80 (d, J = 9.0 Hz, 1H), 7.64 (d, J = 8.0 Hz, 1H), 7.49–7.40 (m, 2H), 7.36–7.22 (m, 4H), 7.09 (d, J = 8.6 Hz, 2H), 5.32 (s, 2H), 4.21 (t, J = 7.1 Hz, 2H), 3.66 (q, J = 7.0 Hz, 6H), 1.95–1.81 (m, 2H), 1.09 (t, J = 7.0 Hz, 9H), 0.50–0.33 (m, 2H); 13C NMR (101 MHz, CDCl3) δ = 158.79, 158.43, 151.66, 134.04, 132.26, 131.94, 131.81, 131.02, 129.42, 129.14, 128.53, 128.47, 128.33, 125.91, 124.60, 122.35, 117.59, 114.20, 67.91, 63.52, 58.50, 57.98, 52.47, 25.59, 24.17, 18.42, 18.29, 7.40.
Synthesis of 5. Yield 87%; mp: 231–233 °C (decom.); anal. calcd for C29H32ClN5O4Si (%): C, 60.25; H, 5.58; N, 12.1; found (%): C, 59.78; H, 5.82; N, 12.27; IR (neat, cm−1): 2858, 1683, 1370, 1323, 1058, 977, 764, 577; 1H NMR (300 MHz, CDCl3) δ = 9.46 (d, J = 8.9 Hz, 1H), 9.16 (s, 1H), 7.86 (d, J = 9.0 Hz, 1H), 7.70 (d, J = 7.9 Hz, 2H), 7.60 (s, 1H), 7.52–7.39 (m, 4H), 7.13 (d, J = 8.4 Hz, 2H), 5.34 (s, 2H), 4.22 (t, J = 7.2 Hz, 2H), 3.60 (t, J = 5.7 Hz, 6H), 2.69 (t, J = 5.7 Hz, 6H), 1.99–1.71 (m, 2H), 0.33–0.17 (m, 2H); 13C NMR (101 MHz, CDCl3) δ = 158.94, 158.59, 151.77, 142.93, 134.08, 131.85, 130.98, 129.34, 129.13, 128.61, 128.54, 128.35, 125.89, 124.55, 122.93, 122.46, 122.37, 117.58, 114.27, 63.75, 57.49, 56.79, 53.46, 50.94, 26.31, 13.20; MS (ES+) calcd for [M + H]+ 578.19; found 578.18.
Results and discussion
Synthesis
The base catalysed nucleophilic substitution of 2-hydroxy-1-naphthaldehyde (1) with propargyl bromide resulted into an alkyne terminated product 2. This alkyne product was separated and was subjected to the microwave promoted condensation reaction with 4-chloroaniline yielding a Schiff base product 3 with terminal alkyne fragment. The Schiff base alkyne 3 with irradiated with microwaves in the presence of Cu(I) that catalysed cycloaddition reaction with 3-azidopropylsilane. The THF
:
TEA 2
:
1 (v/v) acted as solvent media and the reaction was complete within 15 minutes at the temperature of 60 °C. The resulting cyclo-addition product obtained contains 1,2,3-triazole linker with terminal ethoxysilyl group 4 in good yield. This product is hydrolytically unstable which restricts its reactivity and application in water-based systems. Thus, ethoxysilyl group was replaced by an atrane ring 5 by trans-esterification reaction, making it stable for use in various aqueous mediums. This luminescent sensor 5 was further used for chemo-sensing studies (labelled as NTS).
UV-vis spectra
Cationic sensing. The absorbance spectral properties of NTS were examined solvatochromically using chloroform, dimethyl sulfoxide, dimethylformamide, and methanol, which demonstrated dimethyl sulfoxide to be the most suited solvent for photochromic analysis, owing to solubility and better detection studies. To record the metal-ion effect on absorption spectrum of NTS, 20 μM concentration of Ce(III), Cd(II), Fe(II), Cu(I), Zn(II), K(I), Ni(II), Ba(II), Hg(II), Mg(II) and Ag(I) ions were prepared and analysed in DMSO
:
H2O 4
:
1 (v/v). This demonstrated negligible quenching in absorption wavelength maxima, except for Ce(III) ions, where a large stimulated change was recorded as plotted in Fig. 2. The activity of Ce(III) ions in comparison to other metal ions owes to 6s24f15d1 electronic configuration wherein the high energy of 4f–5d configuration (6.3 eV) makes it more reactive. The quenching response of Ce(III) ions is proposed to proceed through electron or energy transfer due to presence of unpaired electrons.41,42
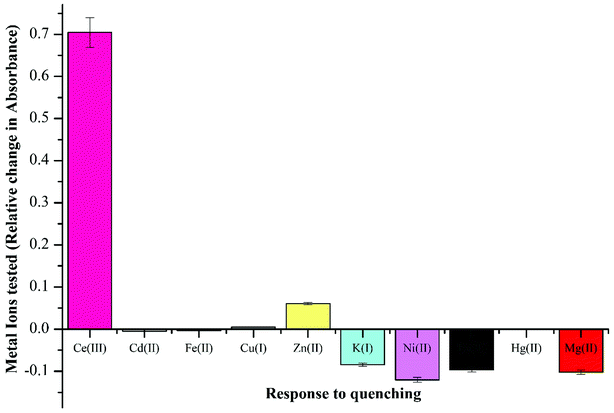 |
| Fig. 2 Competitive absorbance response recorded by luminescent sensor NTS with different cationic species using DMSO : H2O 4 : 1 (v/v) by addition of 5 equiv. of 20 μM metal ion solution with recorded change in maxima value at λ = 322 nm. | |
The ion sensing study was analysed in two different solvent media i.e. DMSO and DMSO
:
H2O 4
:
1 (v/v). The successive addition of 15 equivalent of 20 μM Ce(III) in DMSO yielded a hyperchromic shift in the absorption maxima, commonly referred to as “turn-on” output optical response. The comparative “turn-on” behaviour among each peak decreased upon successive addition of Ce(III) ions, which can be attributed to Internal Charge Transfer (ICT) interaction of NTS with Ce(III) ions. The relative changes in absorption maxima with increasing Ce(III) ion concentration have been shown in Fig. 3.
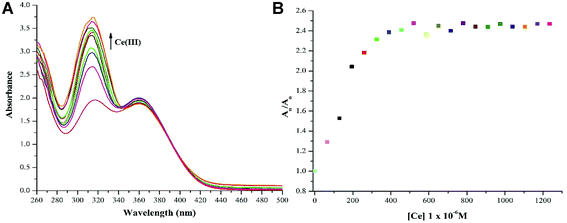 |
| Fig. 3 (a) ‘Turn-on’ response (λmax = 322 nm) recorded with successive addition of 60 μM solution of Ce(III) ions using DMSO as solvent owing to NTS–Ce(III) ion interactions; (b) relative change in absorption maxima (An/Ao) with addition of 15 equiv. of 20 μM of Ce(III) ions; (Ao = absorption maxima of NTS; An = absorption maxima with successive addition of Ce(III) ions). | |
To explore the utility of NTS in water based systems, the absorption maxima values were recorded and plotted for NTS with solvent mixture of DMSO
:
H2O 4
:
1 (v/v) as shown in Fig. 4. The studies performed with DMSO
:
H2O 4
:
1 (v/v) exhibit “turn-off” for absorption maxima at 319 nm accompanied by a blue shift to 307 nm. This titration process creates an isosbestic point at 322 nm accompanied by “turn-on” response for absorption maxima with λmax of 359 nm, indicating the production of single component in response to NTS and Ce(III) ion interactions. This observation can be attributed to the role of water interfering with NTS–Ce(III)–DMSO interactions in the solvent mixture. The lowest detection limit of analysis of Ce(III) ions using NTS probe is 60 μM.
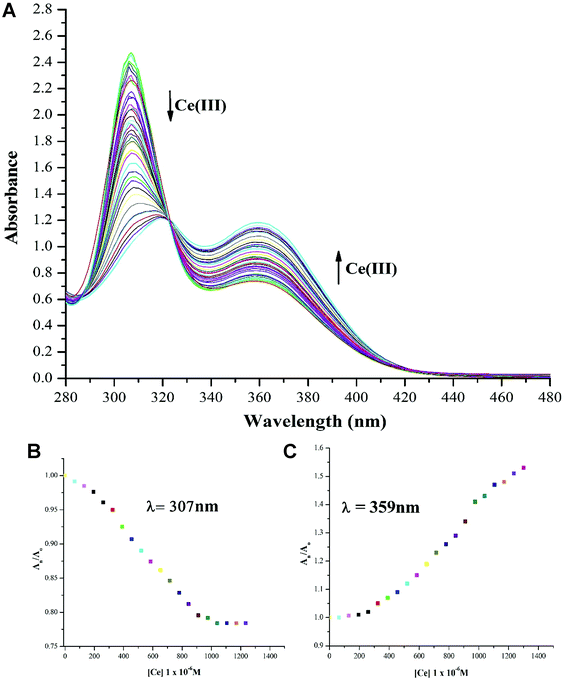 |
| Fig. 4 (a) ‘Turn-off’ response (λmax = 307 nm) associated with red shift (to λmax = 319 nm) recorded with successive addition of 20 μM solution of Ce(III) ions using DMSO : H2O 4 : 1 (v/v) as solvent owing to NTS–Ce(III) ion interaction which is associated with “turn-on” response (λmax = 359 nm) creating an isosbestic point at 322 nm; (b) relative change in absorption maxima (An/Ao) with (λmax = 307 nm) upon addition of 30 equiv. of 20 μM of Ce(III) ions; (c) relative change in absorption maxima (An/Ao) with (λmax = 359 nm) upon addition of 30 equiv. 20 μM of Ce(III) ions; (Ao = absorption maxima of NTS; An = absorption maxima with successive addition of Ce(III) ions). | |
Anionic sensing. NTS–Ce(III) ensemble was tested for anion sensitivity using 13 μM solutions of different anions: NO3−, OCl−, C2O42−, CH3COO−, Cl−, Br−, I− in DMSO
:
H2O 4
:
1 (v/v) as shown in Fig. 5. The change in absorbance was observed with equimolar additions of NO3− ions whereas insignificant changes were observed with OCl− ions and no change was recoded with rest of the anions. The high selectivity displayed by rise in absorption maxima corresponds to “turn-on” response module for NTS–Ce(III)–NO3− system. There was recovery of original absorption maxima upon addition of 5 equiv. (13 μM) solution of NO3− ions solution (Fig. 6). This chemo-sensing of anions using metals have been credited to photo electron transfer (PET) from receptor to luminescent sensor. The metal ion pre-organizes the complex to have a geometry compatible with host–guest binding. The lanthanide ensembles provide a platform for effective anion sensing in water, since aqua molecules being present in vacant coordination site can be displaced by anions. Thus, leading to spectral variations in metal-ensemble-anion system.23–25 On this basis, an expected binding mode was sketched (Fig. 7) with NTS
:
Ce(III) ion binding in 1
:
1 fashion.
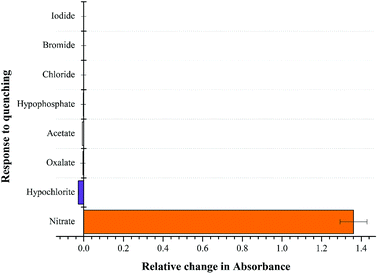 |
| Fig. 5 Competitive absorbance response recorded by excimer NTS–Ce(III) complex with different anionic species using DMSO : H2O 4 : 1 (v/v) by addition of 5 equiv. of 13 μM anionic solutions with recorded change in maxima value at λ = 319 nm. | |
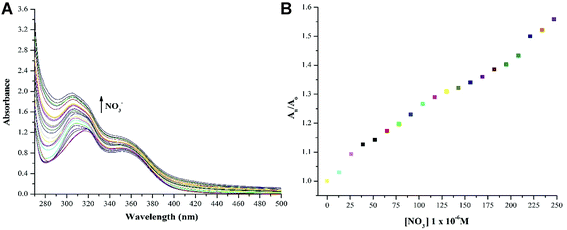 |
| Fig. 6 (a) “Turn-on” response (λmax = 322 nm) associated with blue shift (to λmax = 305 nm) was recorded with the successive addition of 10 μM solution of NO3− ions using DMSO : H2O 4 : 1 (v/v) as solvent which is a result of [NTS–Ce(III)] – NO3− ion interactions; (b) relative change in absorption maxima (An/Ao) with addition of 13 μM of NO3− ions; (Ao = absorption maxima of NTS–Ce(III) ions; An = absorption maxima with successive addition of NO3− ions). | |
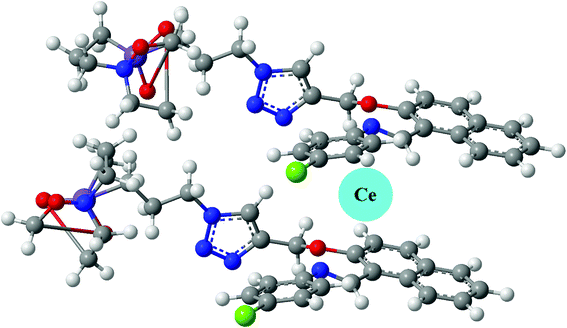 |
| Fig. 7 The binding mode of Ce3+ ions with NTS luminescent sensor. | |
Expected binding mode
NTS have been used as cationic chemo-sensor for cerium ion recognition, followed by nitrate ion assimilation. In order to render insight into the structural features, the geometry of ligand was optimised at DFT/B3LYP/6-31G level of theory. The optimized geometry had all frequencies indicating that obtained structure is a global minimum. Since, the binding of cerium as Ce(III) depends upon the size of supramolecular cavity, it must held together by two molecules in a layered structure as shown in Fig. 7. This is supported by a fact that Ce(III) is a larger ion, it cannot fit into a smaller cavity created by a single NTS molecule.7 Thus, the expected binding stoichiometric ratio for NTS
:
Ce(III) is 2
:
1.
Molecular keypad mimicking
The plotting of ionic response as Boolean function by performing logical operation produces a response that can be implemented as an electronic switch control. These binding studies inspire us to design a [(receptor)–(Ce3+)–(NO3−)] binding model using molecular keypad. The simplification of input signal and output response as molecular keypad was designed as shown in Fig. 8. An alternate way of sensing behaviour for NTS was performed using NTS–NO3− followed by successive addition of 20 μM of Ce(III). The rise in signal intensity was observed with a pattern similar to that observed for NTS–Ce(III) ensemble. These results evidently highlight the two-way sensing of both NO3− ions.
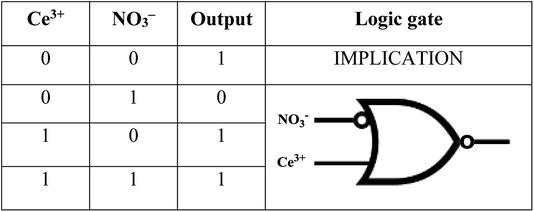 |
| Fig. 8 The relative absorbance intensity change observed with NTS upon addition of Ce3+ and NO3− ions with ‘0’ and ‘1’ signifying ‘no change in λmax’ and ‘turn-off/turn-on’ response, respectively; along with the mimicking of response as IMPLICATION logic gate. | |
Circuit diagram
The developments in electrical circuits to simplify the ion sensing behaviour will attract considerable scientific interest. The response obtained can be drafted as circuit board diagram (as shown in Fig. 9) with NTS as sensing unit and Ce(III) and NO3− ions as keys for circuit completion. The addition of Ce(III) to NTS results into a change in absorption maxima whereas NO3− ions do not alter the λmax value. Further, the addition of NO3− ions into NTS–Ce(III) ensemble or Ce3+ ions to NTS–NO3− ensemble depicts significant change in absorption maxima.
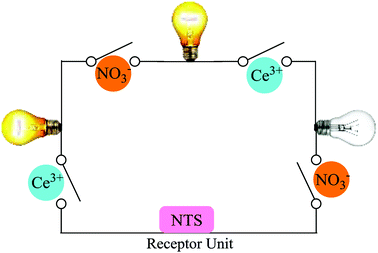 |
| Fig. 9 Ions controlled circuit diagram as a response with NTS. | |
Conclusion
The use of microwave radiations to create NTS luminescent sensor paved an effective pathway for clean, quick and efficient synthesis. The chemo-sensing of Ce(III) ions in DMSO and DMSO
:
H2O 4
:
1 (v/v) solvent system resulted into the unique ‘turn-on’ and ‘turn-off’ responses, respectively. This ensemble was further utilised for NO3− ion detection with a ‘turn-off’ response. The N, O, N cornered caged is generated that can efficiently trap Ce(III) ions till the lower concentration limit of 60 μM. This behaviour of NTS–Ce(III)–NO3− system is mimicked as IMPLICATION logic gate that can be simplified as a circuit diagram.
Conflicts of interest
There are no conflicts to declare.
Acknowledgements
We are thankful to Mrs Renki and Mr Manoj for helping us with UV-vis data collection.
References
- L. Prodi, F. Bolletta, M. Montalti and N. Zaccheroni, Coord. Chem. Rev., 2000, 205(1), 59 CrossRef CAS
. - C. Bargossi, M. C. Fiorini, M. Montalti, L. Prodi and N. Zaccheroni, Coord. Chem. Rev., 2000, 208(1), 17 CrossRef CAS
. - G. Kaur, G. Singh and J. Singh, Mater. Today Chem., 2018, 8, 56 CrossRef
. - Q. Zhao, F. Li and C. Huan, Chem. Soc. Rev., 2010, 39(8), 3007 RSC
. - Y. H. Lau, P. J. Rutledge, M. Watkinson and M. H. Todd, Chem. Soc. Rev., 2011, 40(5), 2848 RSC
. - L. B. Desmonts, D. N. Reinhoudt and M. C. Calama, Chem. Soc. Rev., 2007, 36(6), 993 RSC
. - M. Panchal, A. Kongor, M. Athar, V. Mehta, P. C. Jha and V. K. Jain, New J. Chem., 2018, 42(1), 311 RSC
. - G. Singh, S. S. Mangat, J. Singh, A. Arora and M. Garg, J. Organomet. Chem., 2014, 769, 124 CrossRef CAS
. - G. Singh, S. S. Mangat, J. Singh, A. Arora and R. K. Sharma, Tetrahedron Lett., 2014, 55(4), 903 CrossRef CAS
. - G. Singh, S. S. Mangat, H. Sharma, J. Singh, A. Arora, A. P. S. Pannu and N. Singh, RSC Adv., 2014, 4(69), 36834 RSC
. - G. Singh, A. Arora, S. S. Mangat, J. Singh, S. Chaudhary, N. Kaur and D. C. Lazarte, J. Mol. Struct., 2014, 1079, 173 CrossRef
. - G. Singh, J. Singh, S. S. Mangat and A. Arora, Tetrahedron Lett., 2014, 55(15), 2551 CrossRef CAS
. - G. Singh, J. Singh, S. S. Mangat, J. Singh and S. Rani, RSC Adv., 2015, 5(17), 12644 RSC
. - G. Singh, J. Singh, S. S. Mangat and A. Arora, RSC Adv., 2014, 4, 60853 RSC
. - G. Singh, J. Singh, J. Singh and S. S. Mangat, J. Lumin., 2015, 165, 123 CrossRef CAS
. - J. Hatai, M. Samanta, V. S. R. Krishna, S. Pal and S. Bandyopadhyay, RSC Adv., 2013, 3(44), 22572 RSC
. - M. Liu, Z. Xu, Y. Song, H. Li and C. Xian, J. Lumin., 2018, 198(1), 337 CrossRef CAS
. - H. X. Mai, Y. W. Zhang, R. Si, Z. G. Yan, L. D. Sun, L. P. You and C. H. Yan, J. Am. Chem. Soc., 2006, 128(19), 6426 CrossRef CAS PubMed
. - A. Afkhami, T. Madrakian, A. Shirzadmehr, M. Tabatabaee and H. Bagheri, Sens. Actuators, B, 2012, 174, 237 CrossRef CAS
. - M. R. Awual, M. M. Hasan, A. Shahat, M. Naushad, H. Shiwaku and T. Yaita, Chem. Eng. J., 2015, 265, 210 CrossRef CAS
. - G. Tyler, Plant Soil, 2004, 267(1), 191 CrossRef CAS
. - M. Shamsipur, M. Yousefi and M. R. Ganjali, Anal. Chem., 2000, 72(11), 2391 CrossRef CAS PubMed
. - P. A. Gale and C. Caltagirone, Chem. Soc. Rev., 2015, 44(13), 4212 RSC
. - S. Shinoda and H. Tsukube, Analyst, 2011, 136(3), 431 RSC
. - V. Borse, P. Jain, M. Sadawana and R. Srivastava, Sens. Actuators, B, 2016, 225, 340 CrossRef CAS
. - N. Mishra, K. Poonia, S. K. Soni and D. Kumar, Polyhedron, 2016, 120, 60 CrossRef CAS
. - Y. M. Hijji, B. Barare, A. P. Kennedy and R. Butcher, Sens. Actuators, B, 2009, 136(2), 297 CrossRef CAS
. - C. G. Neochoritis, T. Z. Tzitzikas, C. A. Tsoleridis, J. S. Stephanatou, C. A. Kontogiorgis, D. J. H. Litina and T. C. Papadopoulou, Eur. J. Med. Chem., 2011, 46(1), 297 CrossRef CAS PubMed
. - O. A. Bozdemir, R. Guliyev, O. Buyukcakir, S. Selcuk, S. Kolemen, G. Gulseren and E. U. Akkaya, J. Am. Chem. Soc., 2010, 132(23), 8029 CrossRef CAS PubMed
. - Y. Wang, Y. Huang, B. Li, L. Zhang, H. Song, H. Jiang and J. Gao, RSC Adv., 2011, 1(7), 1294 RSC
. - V. Bhalla and M. Kumar, Org. Lett., 2012, 14(11), 2802 CrossRef CAS PubMed
. - Q. Li, Y. Yue, Y. Guo and S. Shao, Sens. Actuators, B, 2012, 173, 797 CrossRef CAS
. - B. Chen, L. Wang, F. Zapata, G. Qian and E. B. Lobkovsky, J. Am. Chem. Soc., 2008, 130, 6718 CrossRef CAS PubMed
. - A. I. A. Vogel, A Text Book of Practical Organic Chemistry, Longman, London, 4th edn, 1978 Search PubMed
. - A. Bianco, M. Maggini, M. Nogarole and G. Scorrano, Eur. J. Org. Chem., 2006, 13, 2934 CrossRef
. - L. X. Cheng, J. J. Tang, H. Luo, X. L. Jin, F. Dai, J. Yang and B. Zhou, Bioorg. Med. Chem. Lett., 2010, 20(8), 2417 CrossRef CAS PubMed
. - G. A. Harrison, S. Zhou, J. V. Hines and S. C. Bergmeier, J. Comb. Chem., 2010, 12(4), 491 CrossRef PubMed
. - M. J. Khoshkholgh, S. Balalaie, R. Gleiter and F. Rominger, Tetrahedron, 2008, 64(48), 10924 CrossRef CAS
. - G. Singh, P. Kalra, A. Arora, Sanchita, G. Sharma, A. Singh and V. Verma, Inorg. Chim. Acta, 2018, 473, 186 CrossRef CAS
. - H. C. Kolb, M. G. Finn and K. B. Sharpless, Angew. Chem., Int. Ed., 2001, 40, 2004 CrossRef CAS PubMed
. - E. Priyadarshini, N. Pradhan, P. Panda and B. Mishra, Biosens. Bioelectron., 2015, 68, 598 CrossRef CAS PubMed
. - C. Duran, A. Gundogdu, V. N. Bulut, M. Soylak, L. Elci, H. B. Sentürk and M. Tüfekci, J. Hazard. Mater., 2007, 146, 347 CrossRef CAS PubMed
.
Footnote |
† Electronic supplementary information (ESI) available. See DOI: 10.1039/c8ra07294a |
|
This journal is © The Royal Society of Chemistry 2018 |