DOI:
10.1039/C8RA07084A
(Paper)
RSC Adv., 2018,
8, 38157-38165
Biosorption of Cr(VI) from aqueous solution using dormant spores of Aspergillus niger
Received
24th August 2018
, Accepted 30th October 2018
First published on 14th November 2018
Abstract
Spores of Aspergillus niger (denoted as A. niger) were used as a novel biosorbent to remove hexavalent chromium from aqueous solution. The effects of biosorbent dosage, pH, contact time, temperature and initial concentration of Cr(VI) on its adsorption removal were examined in batch mode. The Cr(VI) uptake capacity increased with an increase in Cr(VI) concentration until saturation, which was found to be about 97.1 mg g−1 at pH 2.0, temperature of 40 °C, adsorbent dose of 2.0 g L−1 and initial concentration of 300 mg L−1. Scanning electron microscopy, energy dispersive X-ray spectroscopy, field-emission transmission electron microscopy (FETEM), XPS and Fourier-transform infrared spectroscopy were applied to study the microstructure, composition and chemical bonding states of the biomass adsorbent before and after spore adsorption. The mechanisms of chromate anion removal from aqueous solution by the spores of A. niger were proposed, which included adsorption of Cr(VI) onto the spores followed by its reduction to Cr(III). The reduced Cr(III) was rebound to the biomass mainly through complexation mechanisms, redox reaction and electrostatic attraction. The removal of Cr(VI) by spores of A. niger followed pseudo-second-order adsorption kinetics. Monolayer adsorption of Cr(VI) was revealed by the better fitting of the Langmuir model isotherm rather than multilayer adsorption for the Freundlich model. The results indicated that A. niger spores can be used as a highly efficient biosorbent to remove Cr(VI) from contaminated water.
1. Introduction
Chromium contamination (commonly existing as Cr(VI) and Cr(III)) is one of the most important environmental issues, which mainly comes from industry, including the dye and pigment, fertilizer, film and photography, metallurgical, leather and mining industries.1 Cr(III) and Cr(VI) exhibit very different mobilities and toxicities, with Cr(III) being relatively less soluble and toxic in aqueous systems. In contrast, Cr(VI) is a highly toxic substance with strong solubility, which can induce cancer and mutation.2 Cr(VI) has been recognized as a serious environmental problem due to its toxicity to living organisms and potential carcinogenicity to humans, thus it has attracted increasing concern for its environmental effects. Due to these differences, the discharge levels of Cr(VI) into surface water is strictly controlled to below 0.05 mg L−1 by the U.S. EPA, while total Cr (including Cr(III), Cr(VI) and its other forms) is controlled to below 2 mg L−1.3 Some physicochemical methods used for the removal of Cr(VI) include chemical reduction and precipitation, solvent extraction, ion exchange, adsorption and membrane separation.4 However, these methods have limitations, such as secondary pollution, use of expensive equipment and large quantities of chemical reagents, and incomplete metal removal.5 Thus, alternative biosorption methods are needed.
Metal biosorption is considered to be a more inexpensive and efficient Cr(VI) removal technology due to its simple operation and low energy consumption. Biosorbents that remove heavy metal from wastewater are often classified as algae, microbiological and agricultural waste.6 The fungal adsorbent in microbial adsorbents can adsorb metal ions efficiently due to the composition of its cell walls (dextran, cellulose, chitin, and protein).7 Studies on the biosorption of heavy metals on fungi have shown that the fungal cell wall has many functional groups composed of polysaccharides, proteins and lipids, which are responsible for the binding of metals. In the literature on metal biosorption, many studies have been carried out using mycelia of fungal biomass, such as Mucor, Rhizopus and A. niger.8,9
Removal of Cr(VI) using A. niger fungal biomass has been studied by some researchers.10,11 Park et al. revealed that the removal efficiency of four fungal biosorbents with respect to chromium are as follows: S. cerevisiae (44.2%) > P. chrysogenum (40.3%) > A. niger (29.3%) > R. oryzae (23.5%).12 From their results, A. niger fungus has a lower affinity for chromium adsorption than the other two fungi biomass. However, the use of the spores of A. niger biomass to improve biosorption capacity has not been investigated to date.
To the best of our knowledge, there has been scarce focus on the removal of metal ions by the spores of A. niger. The spores of A. niger have the following advantages over mycelium: (1) small diameter and rough surface; (2) strong survivability; and (3) anti-physical and chemical attack.13,14 Thus, these characteristics may lead to the feasibility of adsorption of heavy metal ions using A. niger spores. The objective of the present study was to investigate the use of spores of A. niger as a biosorbent for the removal of Cr(VI) from aqueous solution. The optimum biosorption conditions were determined as a function of dosage of biomass, initial pH, contact time, temperature and initial concentration. The biosorption data was analyzed via equilibrium and kinetic studies. The biosorption mechanisms were also investigated via SEM-EDS, XPS, FETFM and FTIR analysis.
2. Materials and methods
2.1 Biosorbent preparation
A. niger spores obtained as a reproductive propagule only in solid medium were produced in the late growth stage of A. niger.6 A. niger mycelium was acquired from liquid medium.
2.1.1 Preparation of spores of A. niger. The A. niger strain used in this study was derived from maize straw. The strain was smeared on solid modified Martin medium. A. niger spores scattered on the medium were collected, then kept at room temperature for subsequent experiments.
2.1.2 Preparation of A. niger mycelium. A. niger strain from maize straw was inoculated into liquid Martin medium. After 5 days of incubation, mycelium pellets were harvested and autoclaved for 15 min at 121 °C. Then they were washed with generous amounts of deionized water. After washing, the collected mycelium pellets were dehydrated at 50 °C for 24 h to a constant weight and crushed with a grinder. The dried mycelium powder was stored for further study.6
2.2 Chromium solution analysis and uptake capacities
The Cr(VI) stock solution (1000 mg L−1) was prepared by dissolving 2.828 g of K2Cr2O7 in 1 L double distilled water. The stock solution was appropriately diluted in distilled water to obtain the experimental solutions of the desired concentration. Inductively coupled plasma mass spectrometry was employed to determine the concentrations of the total Cr before and after biosorption. The amount of Cr(VI) adsorbed by the A. niger spores at equilibrium, q (mg g−1), which represents the metal uptake, was evaluated according to the following equation:
where, C0 and Ce are the initial and final concentration of Cr in solution (mg L−1), V is the solution volume and W is the weight of dry spores (g).
2.3 Batch biosorption experiment
In this experiment, the biosorption of Cr(VI) using A. niger spores from an aqueous solution was investigated. The optimum conditions (pH, contact time, initial metal ion concentration, temperature and biomass dosage) for the biosorption of Cr(VI) were elucidated. Biosorption experiments were conducted in batch in a 250 mL Erlenmeyer flask with 100 mL metal solution on a rotatory shaker at 120 rpm. After agitation for the desired time, the solutions were centrifuged at 4000 rpm for 5 min and Cr(VI) uptake capacity was calculated based on the supernatant solution.
2.3.1 Effect of dose of sorbent on Cr(VI) sorption. Dosages of A. niger spores ranging from 0.05 to 0.25 g, with increments of 0.05 g, were added to 100 mL Cr(VI) solution (100 mg L−1) at pH 2 and their adsorption capacity was calculated. Meanwhile, the A. niger mycelium in different dosages, which represents the traditional biosorption method, was compared.
2.3.2 Effect of pH of solution on Cr(VI) sorption. The pH of the solution ranging from 2 to 7, with increments of 1, was maintained at the required value using HCl and NaOH during the study. The experiment was conducted for biosorption at a concentration 100 mg L−1 of Cr(VI) and 0.2 g of spores in 100 mL solution.
2.3.3 Effect of contact time on Cr(VI) sorption. The effects of contact time on the biosorption capacity were investigated in the range of 0–300 min. A. niger spores (0.2 g) were added to 100 mL of a solution containing 100 mg L−1 Cr(VI) in a 250 mL Erlenmeyer flask at pH 2.0.
2.3.4 Effect of initial concentration of solution on Cr(VI) sorption. The effect of initial Cr(VI) concentration was investigated in the range of 25–200 mg L−1. Spore dosage of 0.2 g was added to 100 mL of solution containing varying Cr(VI) concentrations in 250 mL Erlenmeyer flasks at pH 2.0.
2.3.5 Effect of temperature on Cr(VI) sorption. Temperature studies were performed at 20 °C, 30 °C and 40 °C. The spore dosage of 0.2 g was added to 100 mL of Cr(VI) solution with concentrations in the range of 25 to 300 mg L−1.
2.3.6 Effect of other heavy metals on Cr(VI) sorption. Four pairs of competitive biosorption experiments for Cr6+ and Na+, Cr6+ and K+, Cr6+ and Ca2+, and Cr6+ and Mg2+ ions were performed to investigate the selectivity of the spores for Cr(VI) removal. The initial concentration of all the heavy metals was 100 mg L−1, and the biosorbent dosage was 0.2 g.
2.3.7 Effect of biosorption site on Cr(VI) sorption. The spores were mixed with 40 mL of formaldehyde (HCHO) and 80 mL of formic acid (HCOOH), and the reaction mixture was shaken on a rotary shaker for 8 h at 150 rpm. It has been shown that this treatment results in methylation of the amino groups on the biomass. The spores were suspended in 200 mL of anhydrous methanol, and 2 mL of concentrated hydrochloric acid added to the suspension. The reaction mixture was shaken on a rotary shaker for 8 h at 150 rpm. This treatment was expected to cause esterification of the carboxylic groups present on the biomass.15
2.3.8 Effect of real water matrices on Cr(VI) sorption. Songhua River water and groundwater were used as water matrices with 100 mg L−1 chromium solution. The spore dosage of 0.2 g was added to 100 mL of Cr(VI) solution in a 250 mL Erlenmeyer flask at pH 2.0.
2.4 Spore characterization
Scanning electron microscopy, energy-dispersive X-ray spectroscopy, field-emission transmission electron microscopy (FETEM), XPS and Fourier-transform infrared spectroscopy were applied to study the composition, microstructure, chemical status and chemical bonding states of the biomass adsorbent. Scanning electron microscopy (SEM) was used to study the surface morphology before and after spore biosorption. The dried spores were applied to a conductive paste and then sputtered with gold for better image contrast. The image was observed using an SU8010 scanning electron microscope from Japan. FETEM analyses were performed using a Talos F200x field-emission transmission electron microscope from America. In addition, XPS measurements were performed using a PHI 5400 ESCA system equipped with an Al Kα radiation source. Fourier transform infrared spectroscopy (FTIR) analyses were performed using a PerkinElmer instrument to compare the changes in the functional groups before and after spore biosorption.
3. Results and discussion
3.1 The effect of different factors (spore dosage, pH, contact time and initial concentration) on Cr(VI) biosorption
As shown in Fig. 1a, the uptake of the A. niger spores was always higher than that of the mycelium at different dosages. When the biosorbent dosage was 0.5 g L−1 and the initial concentration was 100 mg L−1, the maximum uptake of the A. niger spore biosorbent for Cr(VI) was 58.3 mg g−1, and that for the mycelium was only 12.6 mg g−1. In addition, Table 1 shows some collected data on the biosorption capacity of the pretreated or modified A. niger mycelium. The results indicate that the absorption by A. niger spores is more advantageous than the traditional mycelium. We also observed a decrease in adsorption capacity with an increase in the dosage of A. niger spores. This decrease may be explained as follows: although the total amount of chromium adsorbed by the spores increases, the adsorption capacity per unit of spores is reduced due to the fixed concentration.18 A similar trend was observed in the mycelium used in this study.
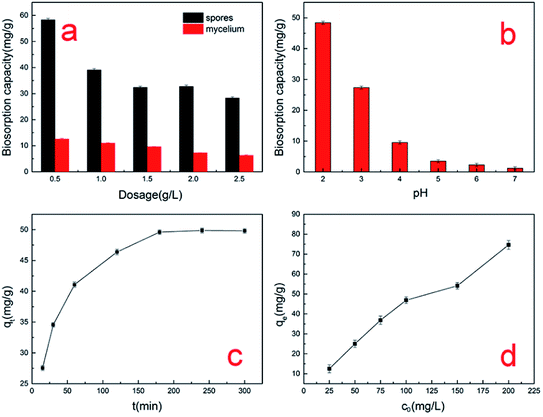 |
| Fig. 1 Effect of factors on the biosorption capacity for Cr(VI) by A. niger spores: (a) spore dosage, (b) pH level, (c) contact time and (d) initial concentration. | |
Table 1 Comparison of the biosorption capacity for Cr6+ by spores and mycelium of Aspergillus niger under different conditions
Biosorbent |
Initial concentration (mg L−1) |
pH |
Biomass dosage (g L−1) |
Cr6+ uptake (mg g−1) |
Reference |
Spores |
100 |
2 |
0.5 |
58.3 |
This study |
Mycelium |
100 |
2 |
0.5 |
12.6 |
This study |
Autoclaved mycelium |
10 |
2 |
2 |
1.5 |
9 |
CTAB pretreated mycelium |
10 |
2 |
2 |
3.1 |
9 |
Acid pretreated mycelium |
10 |
2 |
2 |
2.0 |
9 |
PEI pretreated mycelium |
10 |
2 |
2 |
2.5 |
9 |
Dead mycelium |
50 |
2 |
5 |
10 |
16 |
Mycelium |
30 |
2 |
4 |
5.41 |
17 |
As seen from Fig. 1b, the absorption capacity of A. niger spores for Cr(VI) increased with an increase in acidity, reaching a maximum at pH 2. The absorption capacity of Cr(VI) was 1.2 mg g−1 at pH 7, which increased to 48.4 mg g−1 at pH 2. Other authors have also observed similar results, where the adsorption of Cr(VI) on biomass increased with lowering of pH.19,20 As the pH decreases, the surface of the spores will be protonated to a higher extent, which results in a stronger attraction towards negatively charged chromate anions such as HCrO4−, Cr2O72−, CrO42−, Cr4O132− and Cr3O102− in solution. However, as the pH increases, the OH− ion concentration increases and the total charge on the spore surface becomes negative. This will hinder the biosorption of negatively charged chromate ions, resulting in a dramatic decrease in biosorption capacity at a higher pH.21 When the pH value is lower than 2, the A. niger spores will partially dissolve and their surface chemical nature will change. Therefore, it can be concluded that the adsorption of chromium ions by A. niger spores is obviously dependent on the pH of the solution. Electrostatic adsorption plays an important role in this biosorption process.
As can be seen from Fig. 1c, the data collected over 300 min shows that the biosorption of Cr(VI) consisted of two phases: a primary rapid phase and a secondary slow phase. Many studies observed similar results for biosorption by various biomasses.22,23 The initially rapid phase lasted for about 70 min and the majority of Cr(VI) was absorbed. This suggests that the removal of Cr(VI) was an efficient process. These results were possibly due to electrostatic attraction, extracellular bio-reduction, cellular affinity and microprecipitation. After 100 min, the chromium uptake process became relatively slow and reached saturation at about 200 min. This may be due to the reduction of available active sites of spores.24
The effect of initial Cr(VI) ion concentration was investigated in the range of 25–200 mg L−1. The results are presented in Fig. 1d. The Cr(VI) biosorption capacity of the spores increased with an increase in the initial concentration of Cr(VI) and then reached the maximum value of about 74.6 mg g−1 when the initial concentration was 200 mg L−1. It is worth noting that as the initial concentration increased, the adsorption amount of Cr(VI) increased, which is due to the fact that more active sites on the spore surface were not occupied.25 From Fig. 1d, we can see that the biosorption capacity rapidly increased in the range of 25–100 mg L−1. The biosorption capacity still increased in the range of 100–200 mg L−1, which indicates the particular suitability of spores for the treatment of effluents at concentrations greater than 200 mg L−1. Thus, we should increase the initial concentration in future studies to determine the maximum initial concentration.
3.2 The effect of temperature on Cr(VI) biosorption
Temperature has been reported to play an important role in biosorption. Therefore, batch experiments were carried out to investigate the temperature dependence of the A. niger spores for chromium ion removal. Fig. 2 shows the effect of temperature on the removal of chromium ions at different concentrations. When the concentration was high, an increase in temperature led to a marked increase in the adsorption capacity of the spores. This is mainly due to the sensitivity of the spores themselves to temperature. The Cr(VI) uptake capacity was found to be about 97.1 mg g−1 at pH 2.0 at the temperature of 40 °C with an initial concentration of 300 mg L−1.
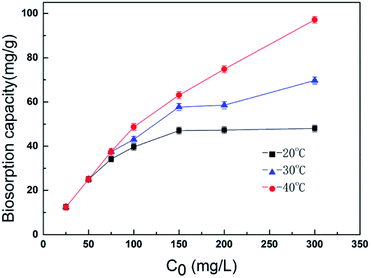 |
| Fig. 2 Effect of temperature on the biosorption capacity for Cr(VI) by A. niger spores. | |
3.3 The effect of other cations on Cr(VI) biosorption
To investigate the selectivity of the spores to remove Cr(VI), other heavy metals such as K+, Na+, Ca2+ and Mg2+ were added separately to the chromium solution. When the biosorbent dosage was 2 g L−1 and the initial Cr(VI) concentration was 100 mg L−1, the maximum biosorption value of the spore biosorbent for Cr(VI) was 44.6 mg g−1. When 100 mg L−1 ion solutions of K+, Na+, Mg2+ and Ca2+ were added, the biosorption capacity for Cr6+ changed from 44.6 mg g−1 to 44.24, 46.26, 39.65 and 37.8 mg g−1, respectively (Fig. 3). Thus, ion interference had little influence on the adsorption of Cr(VI) by A. niger spores.
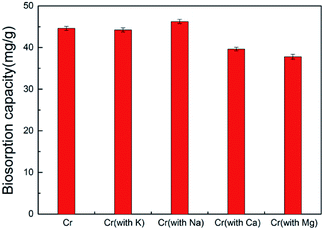 |
| Fig. 3 Biosorption capacity for Cr(VI) and metal ion mixtures with other heavy metals. | |
3.4 The effect of biosorption site
The functional groups of the spore biomass were modified via different chemical treatments to explore the role of different functional groups in the adsorption process.15 As shown in Fig. 4, the spores masking carboxyl and amino groups showed a significant reduction in adsorption. The adsorption capacity of the unmodified spores was 40.9 mg g−1, which was reduced to 28.2 mg g−1 and 7.1 mg g−1 when masking the carboxyl and amino groups, respectively. This indicates that amino and carboxyl groups play an important role in the adsorption of chromium ions by A. niger spores.
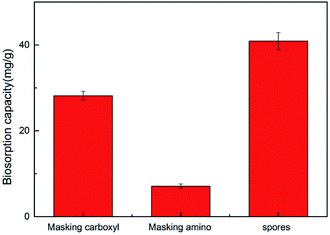 |
| Fig. 4 Amount of metal ions biosorbed by raw biomass and chemically modified biomass. | |
3.5 Effect of real water matrices
To explore the adsorption effect of the A. niger spores used in our experiments in the real environment, we designed two sets of controlled experiments. We used Songhua River water and groundwater as the water matrix to explore the adsorption effect of A. niger spores. As shown in Fig. 5, the same trend as Fig. 1c was observed, where the adsorption capacity increased rapidly first, and then slowly tended to dynamically balance. Compared to double distilled water as the water matrix, the overall decrease in the adsorption capacity of groundwater and surface water as the water matrix may be due to a decrease in the activity of the A. niger spores.
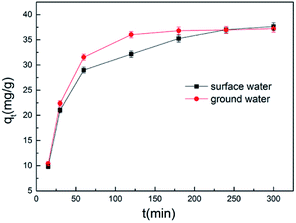 |
| Fig. 5 Biosorption of Cr(VI) using dormant spores of Aspergillus niger in aqueous solutions of ground water and surface water. | |
3.6 SEM-EDX analysis
Scanning electron microscopy equipped with energy dispersive spectroscopy (SEM-EDX) was used to investigate the changes in the A. niger spores as a result of binding with Cr(VI). Fig. 6a and b show the surface morphology of the spores before and after biosorption, respectively. As shown in Fig. 6a, the A. niger spores have a small diameter of about 2 μm and some wrinkles on their surface and a spherical and bead-like structure with a high surface/volume ratio. These features provide good conditions for spore adsorption. The SEM image of the A. niger spores after biosorption is shown in Fig. 6b, where the wrinkles on the surface of the spores disappear. The SEM image of the spores loaded with Cr obtained at 3 h shows that Cr was distributed on the surface of the A. niger spores.
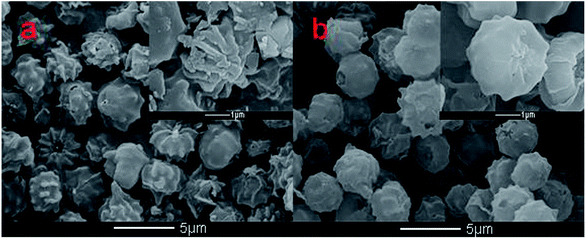 |
| Fig. 6 SEM images of the spores before (a) and after (b) Cr(VI) adsorption. | |
The EDX spectra of the A. niger spores before and after the adsorption of Cr(VI) are shown in Fig. 7a and b, respectively. The EDX analysis of the spores after biosorption confirmed the presence of Cr on the surface of the A. niger spores with the characteristic EDX peak pattern of Cr in Fig. 7b. According to the EDX results, the chromium content (atomic percentage, at%) on the spore surface (at% of 0 to 7.89) increased, while the C (at% of 80.35 to 76.82) and O (at% of 19.65 to 15.29) contents decreased after Cr(VI) biosorption. There is no doubt that this relative content of C, O and Cr is related to the release of C and O and the adsorption of Cr(VI) on the spore surface during Cr(VI) biosorption, respectively. The O- and C-containing functional groups on the spores possibly contributed to Cr binding on the spores.26
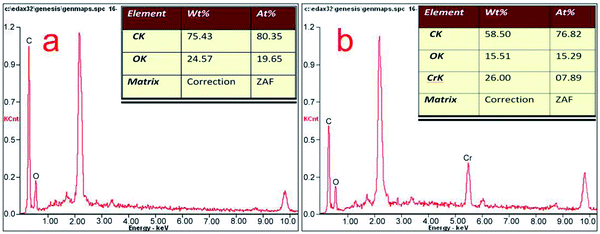 |
| Fig. 7 EDX analysis of the spores before (a) and after (b) Cr(VI) adsorption. | |
3.7 FETEM analysis
FETEM mapping can show the composition and the distribution of elements in the spores. As shown in Fig. 8a, we performed elemental analysis on the micro-domains in the box. Fig. 8b–d show the elements of Cr, C and O on the spores after biosorption, respectively. Cr element was present on the surface of the A. niger spores, again indicating that Cr(VI) was adsorbed on the surface of the biomass.
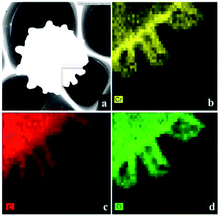 |
| Fig. 8 FETEM images after adsorption by the A. niger spores. | |
3.8 XPS analysis
XPS was employed to provide the valence state of the chromium bound to the spores. The Cr, O, C, and N peaks can be seen in the low-resolution XPS spectra of the Cr-loaded spores (Fig. 9a). Fig. 9b shows the deconvolution of the high-resolution Cr2p XPS spectrum of the spores. As documented, the characteristic binding energy peaks at 576.8–577.7 and 586.0–588.0 eV correspond to Cr(III), and Cr(VI) contributes a higher binding energy.27,28 The binding energy peaks in the Cr 2p spectrum were located at 576.95 and 586.45 eV, indicating that the Cr adsorbed on the surface of spores was in the form of Cr(III). No Cr(VI) was detected in the Cr2p XPS spectrum, indicating that the Cr(VI) adsorbed on the adsorbent was completely reduced to Cr(III). Other researches also reached the same conclusion.16,29,30
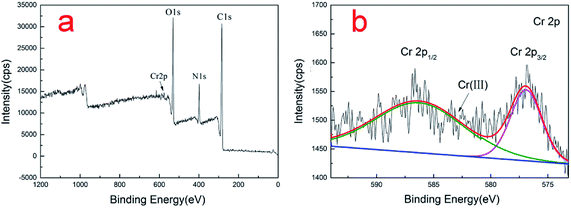 |
| Fig. 9 XPS spectra of (a) spores after Cr(VI) adsorption and (b) Cr 2p1/2 and 2p2/3 orbitals. | |
3.9 FTIR analysis
The FTIR spectra of the A. niger spores within the wavenumber range of 400–4000 cm−1 before and after biosorption were utilized to analyze their functional groups. As shown in Fig. 10, the general profile of the spectra of the spore sample was similar to that of the Cr(VI)-loaded sample, which had adsorbed 100 mg L−1 Cr(VI) solution for 3 h. The spores showed strong peaks at 3448.09, 2925.49, 1648.83., 1558.20, 1382.71, 1081.86, 1033.65 and 582.39 cm−1 in the absence of Cr(VI) (Fig. 10a). The figure shows a number of absorption peaks, indicating the complex nature of the examined spores. These peaks are characteristic for bonded hydroxyl groups, CH2 asymmetric stretch, amide I (protein C
O stretching), amide II (protein N–H bend, C–N stretch), amide III (protein C–N stretch), secondary amide (γ(N–H)+v(C–N)), pyridine(I)β(C–H) and pyridine(II)β(C–H), respectively.31–33 It was clear from the comparison of the FTIR spectra of the spores and Cr(VI)-loaded biosorbent that the bonded hydroxyl groups and amide I (protein C
O stretching) shifted visibly from 3448.09 cm−1 to 3430.74 cm−1 and from 1648.83 cm−1 to 1633.41 cm−1, respectively, indicating that these functional groups participated in Cr(VI) binding.
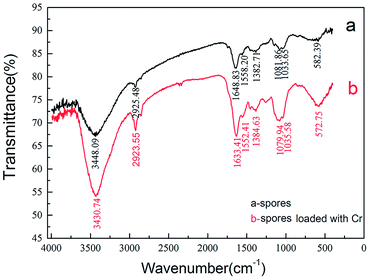 |
| Fig. 10 FTIR spectra of the A. niger spores before and after biosorption. | |
The presence of an CH2 asymmetric stretching band is mainly due to the lipids, while the existence of amide I, amide II and amide III is largely for proteins, and the pyridine bands are mainly from nucleic acids. The shifts in these functional groups during biosorption show that the proteins and nucleic acids in the biosorbent participated in Cr(VI) binding. The results from FTIR spectroscopy of the spores showed that proteins play an important role as a carrier for Cr(VI) transport and chelating substances. In addition, the appearance of pyridine bands proved that nucleic acids were also the main organics related to biosorption, which also means that the Cr(VI) biosorption was coupled with cellular metabolism and active transport.
3.10 Kinetics analysis
To gain insight into the transport mechanism, kinetics analysis was adopted. The kinetics of the Cr(VI) biosorption was assessed by applying Lagergren's first order kinetic equation and pseudo-second order kinetic equation. As can be seen in Fig. 11, the data analysis showed that the biosorption of Cr(VI) followed the pseudo-second-order kinetic model for all six initial concentrations. This indicates that the adsorption rate of the adsorption process is controlled by chemical sorption.34 The values of various kinetic parameters are given in Table 2. Changing the initial concentration of Cr(VI) affects the kinetic parameters of the adsorption process. As the initial concentration of Cr(VI) increases, the value of the equilibrium absorption capacity (q) increases as expected, but the value of the pseudo-second-order rate constant (k2) decreases. Compared with the increase in concentration due to saturation of the adsorption sites, the rate of adsorption increases at a slower rate and thus exhibits a decrease in the apparent rate constant (k2).5 Other authors have also reported consistent results regarding the kinetics of Cr(VI) adsorption on various adsorbents.35,36
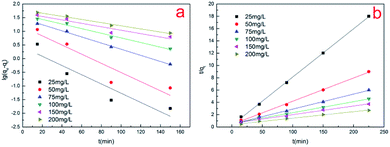 |
| Fig. 11 (a and b) Lagergren's first-order equation and the pseudo-second-order equation, respectively. | |
Table 2 Simulation of sorption kinetic equations and corresponding parameters
C0 (mg L−1) |
Lagergren's first-order |
Pseudo-second order |
k1 |
q |
R2 |
k2 |
q |
R2 |
(min−1) |
(mg g−1) |
(g mg−1 min−1) |
(mg g−1) |
25 |
0.038898 |
2.66238 |
0.80714 |
0.022265 |
12.75022 |
0.99945 |
50 |
0.038575 |
14.73941 |
0.82164 |
0.003565 |
26.44803 |
0.99779 |
75 |
0.025794 |
29.20183 |
0.9965 |
0.001309 |
40.8998 |
0.99865 |
100 |
0.01946 |
40.2337 |
0.97945 |
0.000576 |
56.14823 |
0.99385 |
150 |
0.014486 |
46.65734 |
0.88396 |
0.00033 |
71.22507 |
0.98564 |
200 |
0.013265 |
60.00674 |
0.98345 |
0.000291 |
94.87666 |
0.9902 |
3.11 Isotherm analysis
In this study, the Freundlich and Langmuir isotherms models were used to analyze the Cr(VI) sorption data (Fig. 12). The Langmuir isotherm model shows very close agreement with the experimental adsorption data at temperatures of 20 °C, 30 °C and 40 °C since the R2 values were 0.9995, 0.9880 and 0.9707, respectively. This indicates that the surface of the A. niger spores is homogenous and only one chromium molecule is accepted per active site. The adsorbed molecules are organized into a single layer, and all sites are equal in energy and there is no interaction between the adsorbed molecules.1 The adsorption parameters for the biosorption of chromium on A. niger spores at three temperatures are given in Table 3. The maximum q for Cr(VI) on the A. niger spores was increased from 48.33253 to 94.60738 mg g−1 with an increase in temperature from 20 °C to 40 °C. Other researchers have reported similar results.37,38 The increase in spore adsorption capacity with an increase in temperature may be due to the increase in the kinetic energy of adsorbent particles, resulting in increased collision frequency.39
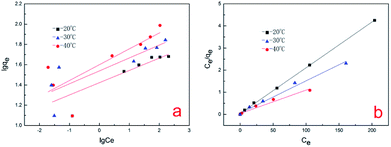 |
| Fig. 12 (a and b) Simulation of Freundlich and Langmuir isotherms, respectively. | |
Table 3 Simulation of isotherm sorption models and corresponding parameters
Temperature (K) |
Freundlich |
Langmuir |
k |
n |
R2 |
b |
q max |
R2 |
(L mg−1) |
(L mg−1) |
(mg g−1) |
293 |
26.43991 |
8.246743 |
0.6625 |
0.541623 |
48.33253 |
0.9995 |
303 |
34.58916 |
7.849294 |
0.6650 |
0.26045 |
68.58711 |
0.9880 |
313 |
39.80797 |
6.396316 |
0.5775 |
0.268888 |
94.60738 |
0.9707 |
4. Conclusion
The spores of A. niger act as an effective biosorbent to adsorb cationic heavy metals. Experiments showed that the maximum biosorption capacity of the spores is much higher than A. niger mycelium. The Cr(VI) uptake capacity increased with an increase in Cr(VI) concentration until saturation, which was found to be about 97.1 mg g−1 at pH 2.0, temperature of 40 °C and adsorbent dose of 2.0 g L−1. The biosorption process followed the pseudo-second order and Langmuir models well, indicating that the adsorption process is mainly controlled by chemical sorption. The mechanism of spores removing chromate anions from water includes adsorption of Cr(VI) onto the spores by electrostatic attraction, and then reduction of Cr(VI) to Cr(III) by complexation mechanism and redox reaction. These results were supported by EDX, FETEM, XPS and FTIR analysis. Thus, it may be concluded that A. niger spores exhibit potential for the removal of Cr(VI) in aqueous solution. However, further research is needed to establish the process with specific attention to the regeneration of the sorbent and the recovery of the adsorbed metal.
Conflicts of interest
The authors declared that they have no conflicts of interest to this work. We declare that we do not have any commercial or associative interest that represents a conflict of interest in connection with the work submitted.
Acknowledgements
This work was funded by the National Natural Science Foundation of China (No. 61605031). Science Foundation of Heilongjiang Academy of Sciences (No. ZNBZ2018GJS04). Science Foundation of Department of Science and Technology of Heilongjiang Province (No. YS17A06).
Notes and references
- Y. Khambhaty, K. Mody, S. Basha and B. Jha, Chem. Eng. J., 2009, 145, 489–495 CrossRef CAS.
- J. Barnhart, Regul. Toxicol. Pharmacol., 1997, 26, S3–S7 CrossRef CAS PubMed.
- A. Baral and R. D. Engelken, Environ. Sci. Policy, 2002, 5, 121–133 CrossRef CAS.
- S. K. Mehta and J. P. Gaur, Crit. Rev. Biotechnol., 2005, 25, 113–152 CrossRef CAS PubMed.
- N. Tewari, P. Vasudevan and B. K. Guha, Biochem. Eng. J., 2005, 23, 185–192 CrossRef CAS.
- J. Y. Wang and C. W. Cui, RSC Adv., 2017, 7, 14069–14077 RSC.
- D. Sud, G. Mahajan and M. P. Kaur, Bioresour. Technol., 2008, 99, 6017–6027 CrossRef CAS PubMed.
- R. S. Bai and T. E. Abraham, Bioresour. Technol., 2001, 79, 73–81 CrossRef.
- D. P. Mungasavalli, T. Viraraghavan and Y. C. Jin, Colloids Surf., A, 2007, 301, 214–223 CrossRef CAS.
- R. S. Bai and T. E. Abraham, J. Sci. Ind. Res., 1998, 57, 821–824 CAS.
- K. C. Sekhar, S. Subramanian, J. M. Modak and K. A. Natarajan, Int. J. Miner. Process., 1998, 53, 107–120 CrossRef CAS.
- D. Park, Y. S. Yun and J. M. Park, Process Biochem., 2005, 40, 2559–2565 CrossRef CAS.
- W. R. Bowen, R. W. Lovitt and C. J. Wright, Colloids Surf., A, 2000, 173, 205–210 CrossRef CAS.
- B. E. Priegnitz, A. Wargenau, U. Brandt, M. Rohde, S. Dietrich, A. Kwade and R. Krull, Fungal Genet. Biol., 2012, 49, 30–38 CrossRef CAS PubMed.
- A. Kapoor and T. Viraraghavan, Bioresour. Technol., 1997, 61, 221–227 CrossRef CAS.
- D. Park, Y. S. Yun, J. H. Jo and J. M. Park, Water Res., 2005, 39, 533–540 CrossRef CAS PubMed.
- R. Kumar, N. R. Bishnoi, B. K. Garima and K. Bishnoi, Chem. Eng. J., 2008, 135, 202–208 CrossRef CAS.
- Z. K. Wang, C. L. Ye, X. Y. Wang and J. Li, Appl. Surf. Sci., 2013, 287, 232–241 CrossRef CAS.
- G. Ç. Dönmez, Z. Aksu, A. Öztürk and T. Kutsal, Process Biochem., 1999, 34, 885–892 CrossRef.
- R. S. Prakasham, J. S. Merrie, R. Sheela, N. Saswathi and S. V. Ramakrishna, Environ. Pollut., 1999, 104, 421–427 CrossRef CAS.
- H. Niu and B. Volesky, Hydrometallurgy, 2003, 71, 209–215 CrossRef CAS.
- S. Tunali, I. Kiran and T. Akar, Miner. Eng., 2005, 18, 681–689 CrossRef CAS.
- Y. Sağ and Y. Aktay, Biochem. Eng. J., 2002, 12, 143–153 CrossRef.
- Y. Sağ and Y. Aktay, Process Biochem., 2000, 36, 157–173 CrossRef.
- G. Dönmez and Z. Aksu, Process Biochem., 2002, 38, 751–762 CrossRef.
- C. Chen, D. H. Wen and J. L. Wang, Bioresour. Technol., 2014, 156, 380–383 CrossRef CAS PubMed.
- D. Park, Y. S. Yun and J. M. Park, J. Colloid Interface Sci., 2008, 317, 54–61 CrossRef CAS PubMed.
- H. X. Chen, J. F. Dou and H. B. Xu, Appl. Surf. Sci., 2017, 425, 728–735 CrossRef CAS.
- D. Park, Y. S. Yun and J. M. Park, Environ. Sci. Technol., 2004, 38, 4860–4864 CrossRef CAS PubMed.
- J. L. G. Torresdey, K. J. Tiemann and V. Armendariz, J. Hazard. Mater., 2000, B80, 175–188 CrossRef.
- D. Park, Y. S. Yun, J. H. Jo and J. M. Park, Chemosphere, 2005, 60, 1356–1364 CrossRef CAS PubMed.
- J. M. Tobin, D. G. Cooper and R. J. Neufeld, Appl. Environ. Microbiol., 1984, 47, 821–824 CAS.
- T. Mathialagan and T. Viraraghavan, Bioresour. Technol., 2009, 100, 549–558 CrossRef CAS PubMed.
- Y. Ding, D. B. Jing, H. L. Gong, L. B. Zhou and X. S. Yang, Bioresour. Technol., 2012, 114, 20–25 CrossRef CAS PubMed.
- R. Elangovan, L. Philip and K. Chandraraj, J. Hazard. Mater., 2008, 152, 100–112 CrossRef CAS PubMed.
- T. Karthikeyan, S. Rajgopal and L. R. Miranda, J. Hazard. Mater., 2005, B124, 192–199 CrossRef PubMed.
- Y. Sağ and T. Kutsal, Process Biochem., 2000, 35, 801–807 CrossRef.
- V. K. Gupta, A. K. Shrivastava and N. Jain, Pergamon, 2001, vol. 17, pp. 4079–4085.
- Y. Nuhoglu and E. Oguz, Process Biochem., 2003, 38, 1627–1631 CrossRef CAS.
|
This journal is © The Royal Society of Chemistry 2018 |
Click here to see how this site uses Cookies. View our privacy policy here.