DOI:
10.1039/C8RA06217J
(Paper)
RSC Adv., 2018,
8, 33256-33268
Synthesis, surface activities, and aggregation behavior of phenyl-containing carboxybetaine surfactants†
Received
23rd July 2018
, Accepted 19th September 2018
First published on 26th September 2018
Abstract
A series of carboxybetaine surfactants, 2-((4-(alkoxy)-3,5-dimethylbenzyl)dimethyl-ammonio)acetate (CnOBCb, where n represents the hydrocarbon chain length of 12, 14, 16 and 18), were synthesized by an efficient and high-yield route for the first time. The surface activities and aggregation behavior of CnOBCb in aqueous solution were investigated by equilibrium surface tension, interfacial tension, steady-state fluorescence, dynamic light scattering (DLS), cryogenic transmission electron microscopy (cryo-TEM) and negative-staining transmission electron microscopy (TEM) measurements. In comparison with conventional N-alkylbetaine surfactants (CnCb), the CnOBCb species, with a phenyl group introduced in the hydrophobic tail, exhibited excellent surface activities, including lower critical micelle concentration (cmc), lower surface tension and stronger adsorption tendency at an air/water interface. CnOBCb also displayed high efficiency in reducing the toluene/water interfacial tension, with C12OBCb achieving an ultralow interfacial tension (10−3 mN m−1) at concentrations from 0.2 to 1 mmol dm−3. The fluorescence intensity ratio and the scattering intensity in DLS measurements changed remarkably at concentrations around the cmc. Furthermore, the CnOBCb species spontaneously formed vesicles above the cmc in aqueous solution, and the size of the aggregates increased with increasing surfactant concentrations. Flooding experiments showed that CnOBCb could effectively improve oil recovery by 7.85–10.55%.
1. Introduction
Zwitterionic surfactants incorporating both positively and negatively charged hydrophilic headgroups in the same molecule are attracting increasing attention.1,2 As an important type of zwitterionic surfactant, betaines have a hydrophilic headgroup comprised of a quaternary ammonium group and an anionic group such as carboxylate, sulfonate, sulfate, or phosphate.3 Betaine-type surfactants possess distinctive physicochemical properties such as high foam stability, skin and eye compatibility, high efficiency in lowering surface tension, and low toxicity,4 leading to a wide range of applications in enhanced oil recovery,5–7 detergents,8,9 cosmetics2,10 and textile production.11
As the geometry of betaine-type surfactants has a significant effect on their physicochemical properties, considerable efforts have been devoted to their structural optimization. Most reported modifications have been focused on varying the hydrophobic tail,12–15 hydrophilic headgroup,16–18 and spacer group.19 Meanwhile, the introduction of a phenyl group into a hydrocarbon chain significantly influences physicochemical properties such as the critical micelle concentration (cmc), surface tension (γ), standard free energy of micellization (ΔG0mic), and aggregation structures.20–26 The location of the phenyl group can also affect the size and structure of the micellar aggregates.27 Thus, incorporating a phenyl group into betaines may results in phenyl-containing betaine-type surfactants with unique properties. For example, Hu et al.28 studied the adsorption behavior of two pairs of novel betaines and found that the benzyl-substituted alkyl betaines exhibited lower cmc values and enhanced efficiency in lowering the surface tension at air/water interface than the corresponding linear surfactants. Further investigation of the interfacial properties of the same set of surfactants via molecular dynamics simulations confirmed that benzene ring incorporation could change and fix the order of the alkyl chain to some extent.29 However, there are few studies on phenyl-containing betaine-type surfactants.28–31 The syntheses of phenyl-containing betaine-type surfactants reported in literature are time consuming and inefficient. Thus, the development of an efficient method for synthesizing this type surfactant is still desirable. Further, a systematic study of the effects of the phenyl group on the surface activities and aggregation behavior of these surfactants would help provide a full understand of betaine-type surfactants and experimental guidelines for their application.
In this work, we report a novel series of phenyl-containing carboxybetaine surfactants, 2-((4-(alkyoxy)-3,5-dimethylbenzyl)dimethylammonio)acetate (CnOBCb, where n represents hydrocarbon chain length of 12, 14, 16 and 18), synthesized in high yield using a three-step reaction. The surface activities and aggregation behavior of these surfactants in aqueous solution were systematically investigated by equilibrium surface tension, interfacial tension, steady-state fluorescence, dynamic light scattering, cryogenic transmission electron microscopy (cryo-TEM) and negative-staining transmission electron microscopy (TEM) measurements. In addition, the effect of phenyl group incorporation, hydrocarbon chain length, and the surfactant concentration on the adsorption and aggregation behavior was clarified by comparison with N-alkylbetaine surfactants (CnCb).
2. Experimental methods
2.1. Materials
n-Dodecyl bromide (98%), n-tetradecyl bromide (98%), n-hexadecyl bromide (97%), n-octadecyl bromide (97%), 2,6-dimethylphenol (99%) were purchased from Aladdin Co. (China) and used without further purification. N,N-Dimethylglycine (98%) was supplied by Alfa Aesar (USA). Sodium hydroxide (99%), paraformaldehyde (99%), tetrabutylammonium bromide (TBAB) (99%), calcein (99%), sodium bicarbonate (99%) and zinc chloride (98%) were obtained from Tianjin Huchen chemical plant (China). Lauryl betaine (C12Cb) (90%) was supplied by BEIJING OUHE TECHNOLOGY CO., LED. Crude oil was obtained from Xinjiang Oilfield (China). The viscosity of oil was 15.8 mPa s at 40 °C, and its density was 0.86 g cm−3. All organic solvents were purchased from Beijing Chemical Co. (China) and were of analytical grade. Deionized water was used in all experiments.
2.2. Synthesis
All the surfactants were synthesized according to Scheme 1. 1H and 13C NMR spectra were recorded at 25 °C on a 500 MHz Bruker III AVANCE spectrometer. Chemical shifts were reported in ppm relative to TMS (for 1H, δ 0.00), CD3OD (for 1H, δ 3.31; for 13C, δ 48.80), and CDCl3 (for 1H, δ 7.26; for 13C, δ 77.00). High-resolution mass spectra analysis was performed on a Bruker APEX_ULTRA_ 9.4 FT-ICR-MS spectrometer. 1H and 13C NMR spectra were provided in the ESI.†
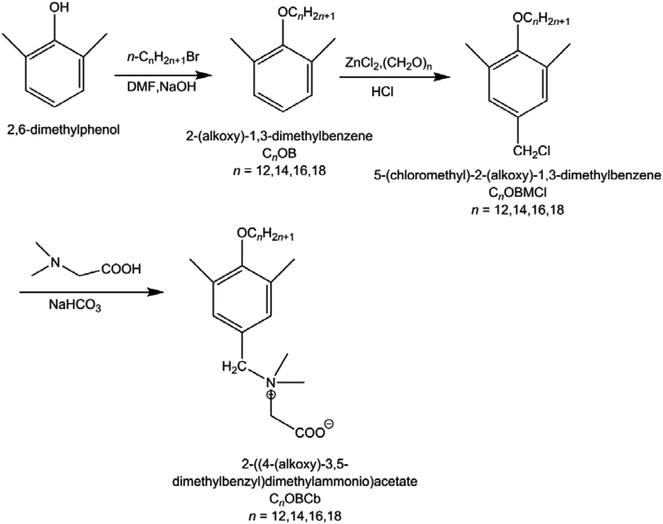 |
| Scheme 1 Synthesis route of CnOBCb (n = 12, 14, 16, 18). | |
2.2.1. Synthesis of 2-(alkoxy)-1,3-dimethylbenzene (CnOB). Sodium hydroxide (0.1 mol, 4 g) was slowly added to a solution of 2,6-dimethylphenol (0.1 mol, 12.2 g) in ethanol (20 mL), and the mixture was stirred at 25 °C for 2 h. After heating to 60 °C, n-dodecyl, n-tetradecyl, n-hexadecyl, or n-octadecyl bromide (0.1 mol, 25.4–34.3 g) in N,N-dimethylformamide (30 mL) was added dropwise to the residue, and the resulting mixture was stirred for 6 h. Then the hot mixture was cooled to room temperature and filtered to remove the insoluble inorganic salt. The filtrate was washed with deionized water and dried over anhydrous sodium sulfate. After filtration, the solution was concentrated in vacuo and CnOB was obtained by reduced pressure distillation (188–226 °C, −0.1 MPa) as a colorless liquid.
2.2.1.1. 2-(Dodecyloxy)-1,3-dimethylbenzene (C12OB). Colorless liquid, yield (76%), 1H NMR (500 MHz, CDCl3) δ 0.87–0.91 (t, 3H, Asd CH3), 1.28–1.38 (m, 16H, –O–CH2–CH2–CH2–(CH2)8–CH3), 1.46–1.54 (m, 2H, –O–CH2–CH2–CH2–(CH2)8–CH3), 1.77–1.84 (m, 2H, –O–CH2–CH2–CH2–(CH2)8–CH3), 2.28 (s, 6H, Ar–(CH3)2), 3.74–3.77 (t, 2H, –O–CH2–CH2–CH2–(CH2)8–CH3), 6.89–6.92 (t, 1H, para-ArH), 6.99–7.01 (d, 2H, meta-ArH). 13C NMR (125 MHz, CDCl3) δ 14.1, 16.2, 22.7, 26.1, 29.3, 29.5, 29.6, 29.6, 29.6, 30.4, 31.9, 72.2, 122.5, 128.6, 130.8, 156.0. 156.2. HRMS (ESI+) m/z: calcd for C20H35O+ (M + H), 291.2684; found, 291.2682.
2.2.1.2. 2-(Tetradecyloxy)-1,3-dimethylbenzene (C14OB). Colorless liquid, yield (79%), 1H NMR (500 MHz, CDCl3) δ 0.88–0.91 (t, 3H, –CH3), 1.28–1.37 (m, 20H, –O–CH2–CH2–CH2 –(CH2)10–CH3), 1.47–1.53 (m, 2H, –O–CH2–CH2–CH2–(CH2)10–CH3), 1.78–1.84 (m, 2H, –O–CH2–CH2–CH2–(CH2)10–CH3), 2.28 (s, 6H, Ar–(CH3)2), 3.75–3.77 (t, 2H, –O–CH2–CH2–CH2–(CH2)10–CH3), 6.89–6.92 (t, 1H, para-ArH), 7.00–7.01 (d, 2H, meta-ArH). 13C NMR (125 MHz, CDCl3) δ 14.1, 16.2, 22.7, 26.1, 29.3, 29.5, 29.6, 29.6, 29.6, 29.7, 30.4, 31.9, 72.2, 123.5, 128.7, 130.9, 156.0. 156.2. HRMS (ESI+) m/z: calcd for C22H39O+ (M + H), 319.2295; found, 319.2296.
2.2.1.3. 2-(Hexadecyloxy)-1,3-dimethylbenzene (C16OB). White solid, yield (74%), 1H NMR (500 MHz, CDCl3) δ 0.88–0.91 (t, 3H, –CH3), 1.28–1.38 (m, 24H, –O–CH2–CH2–CH2–(CH2)12–CH3), 1.48–1.54 (m, 2H, –O–CH2–CH2–CH2–(CH2)12–CH3), 1.78–1.84 (m, 2H, –O–CH2–CH2–CH2–(CH2)12–CH3), 2.29 (s, 6H, Ar–(CH3)2), 3.75–3.78 (t, 2H, –O–CH2–CH2–CH2–(CH2)10–CH3), 6.90–6.93 (t, 1H, para-ArH), 7.00–7.02 (d, 2H, meta-ArH). 13C NMR (125 MHz, CDCl3) δ 14.1, 16.2, 22.7, 26.1, 29.3, 29.5, 29.6, 29.6, 29.6, 29.7, 30.4, 31.9, 72.2, 123.5, 128.7, 130.9, 156.0. 156.2. HRMS (ESI+) m/z: calcd for C24H43O+ (M + H), 347.3308; found, 347.3310.
2.2.1.4. 2-(Octadecyloxy)-1,3-dimethylbenzene (C18OB). White solid, yield (73%), 1H NMR (500 MHz, CDCl3) δ 0.87–0.90 (t, 3H, –CH3), 1.27–1.38 (m, 28H, –O–CH2–CH2–CH2–(CH2)14–CH3), 1.47–1.53 (m, 2H, –O–CH2–CH2 –CH2–(CH2)14–CH3), 1.78–1.83 (m, 2H, –O–CH2–CH2–CH2–(CH2)14–CH3), 2.28 (s, 6H, Ar–(CH3)2), 3.74–3.77 (t, 2H, –O–CH2–CH2–CH2–(CH2)10–CH3), 6.89–6.92 (t, 1H, para-ArH), 7.00–7.01 (d, 2H, meta-ArH). 13C NMR (125 MHz, CDCl3) δ 14.1, 16.2, 22.6, 26.1, 29.3, 29.5, 29.6, 29.6, 29.6, 29.7, 30.4, 31.9, 72.2, 123.5, 128.7, 130.9, 156.0. 156.2. HRMS (ESI+) m/z: calcd for C26H47O+ (M + H), 375.3620; found, 375.3621.
2.2.2. Synthesis of 5-(chloromethy)-2-(alkoxy)-1,3-dimethylbenzene (CnOBMCl). Acetic acid (20 mL) and TBAB (0.005 mol, 1.6 g) were added to a stirred solution of CnOB (0.1 mol, 29.1–37.3 g) dissolved in approximately 30 mL of n-hexane. Then, dry hydrogen chloride gas was continuously bubbled through the solution at room temperature. After increasing the temperature to 70 °C, zinc chloride (0.1 mol, 13.6 g) and paraformaldehyde (0.1 mol, 3 g) were slowly added, and the resulting mixture was refluxed for 8 h. The upper organic phase was separated, washed with deionized water, and then dried over anhydrous sodium sulfate. After filtration, the filtrate was concentrated under reduced pressure and CnOBMCl was purified by flash column chromatography using petroleum ether and ethyl acetate = 20
:
1 as the eluent.
2.2.2.1. 5-(Chloromethy)-2-(dodecyloxy)-1,3-dimethylbenzene (C12OBMCl). White solid, yield (82%), 1H NMR (500 MHz, CDCl3) δ 0.87–0.90 (t, 3H, –CH3), 1.27–1.36 (m, 16H, –O–CH2–CH2–CH2–(CH2)8–CH3), 1.46–1.52 (m, 2H, –O–CH2–CH2–CH2– (CH2)8–CH3), 1.76–1.82 (m, 2H, –O–CH2–CH2–CH2–(CH2)8–CH3), 2.27 (s, 6H, Ar–(CH3)2), 3.73–3.75 (t, 2H, –O–CH2–CH2–CH2–(CH2)8–CH3), 4.50 (s, 2H, para-Ar–CH2Cl), 7.03 (s, 2H, meta-ArH). 13C NMR (125 MHz, CDCl3) δ 14.1, 16.2, 22.6, 26.1, 29.5, 29.6, 29.6, 29.6, 30.3, 31.9, 46.3, 72.3, 129.1, 131.4, 132.4, 156.2. HRMS (ESI+) m/z: calcd for C21H35O+ (M − Cl), 303.2682; found, 303.2685.
2.2.2.2. 5-(Chloromethy)-2-(tetradecyloxy)-1,3-dimethylbenzene (C14OBMCl). White solid, yield (77%), 1H NMR (500 MHz, CDCl3) δ 0.87–0.90 (t, 3H, –CH3), 1.27–1.36 (m, 16H, –O–CH2–CH2–CH2–(CH2)10–CH3), 1.46–1.52 (m, 2H, –O–CH2–CH2–CH2–(CH2)10–CH3), 1.76–1.82 (m, 2H, –O–CH2–CH2–CH2–(CH2)10–CH3), 2.27 (s, 6H, Ar–(CH3)2), 3.73–3.75 (t, 2H, –O–CH2–CH2–CH2–(CH2)10–CH3), 4.50 (s, 2H, para-Ar–CH2Cl), 7.03 (s, 2H, meta-ArH). 13C NMR (125 MHz, CDCl3) δ 14.1, 16.2, 22.6, 26.1, 29.3, 29.5, 29.6, 29.6, 29.6, 29.6, 30.3, 31.9, 46.3, 72.3, 129.1, 131.3, 132.4, 156.2. HRMS (ESI+) m/z: calcd for C23H39O+ (M − Cl), 331.2995; found, 331.2997.
2.2.2.3. 5-(Chloromethy)-2-(hexadecyloxy)-1,3-dimethylbenzene (C16OBMCl). White solid, yield (81%), 1H NMR (500 MHz, CDCl3) δ 0.87–0.90 (t, 3H, –CH3), 1.27–1.36 (m, 16H, –O–CH2–CH2–CH2–(CH2)12–CH3), 1.46–1.52 (m, 2H, –O–CH2–CH2–CH2–(CH2)12–CH3), 1.76–1.82 (m, 2H, –O–CH2–CH2–CH2–(CH2)12–CH3), 2.27 (s, 6H, Ar–(CH3)2), 3.73–3.75 (t, 2H, –O–CH2–CH2–CH2–(CH2)12–CH3), 4.50 (s, 2H, para-Ar–CH2Cl), 7.03 (s, 2H, meta-ArH). 13C NMR (125 MHz, CDCl3) δ 14.1, 16.2, 22.6, 26.1, 29.3, 29.5, 29.6, 29.6, 29.6, 29.6, 29.6, 30.3, 31.9, 46.3, 72.3, 129.1, 131.4, 132.4, 156.2. HRMS (ESI+) m/z: calcd for C25H43O+ (M − Cl), 359.3308; found, 359.3308.
2.2.2.4. 5-(Chloromethy)-2-(octadecyloxy)-1,3-dimethylbenzene (C18OBMCl). White solid, yield (75%), 1H NMR (500 MHz, CDCl3) δ 0.87–0.90 (t, 3H, –CH3), 1.27–1.36 (m, 16H, –O–CH2–CH2–CH2–(CH2)14–CH3), 1.46–1.52 (m, 2H, –O–CH2–CH2–CH2– (CH2)14–CH3), 1.76–1.82 (m, 2H, –O–CH2–CH2–CH2–(CH2)14–CH3), 2.27 (s, 6H, Ar–(CH3)2), 3.73–3.75 (t, 2H, –O–CH2–CH2–CH2–(CH2)14–CH3), 4.50 (s, 2H, para-Ar–CH2Cl), 7.03 (s, 2H, meta-ArH). 13C NMR (125 MHz, CDCl3) δ 14.1, 16.2, 22.6, 26.1, 29.3, 29.5, 29.5, 29.6, 29.6, 29.6, 29.6, 30.3, 31.9, 46.2, 72.3, 129.1, 131.4, 132.4, 156.2. HRMS (ESI+) m/z: calcd for C27H47O+ (M − Cl), 387.3621; found, 387.3620.
2.2.3. Synthesis of CnOBCb. Sodium bicarbonate (0.02 mol, 1.68 g) was slowly added to a solution of N,N-dimethylglycine (0.02 mol, 2.06 g) in 40 mL of ethanol at 80 °C and stirred for approximately 1 h. CnOBMCl (0.02 mol, 6.76–8.44 g) dissolved in 15 mL of n-hexane was added dropwise into this solution, and the mixture was refluxed for 6 h. After all solvent was evaporated, the resulting waxy crude betaine surfactant was extracted with 20 mL of ethanol. The extract was evaporated to dryness and repeatedly recrystallized from a mixture of methanol and acetone. The product was then dried at 60 °C under vacuum.
2.2.3.1. 2-((4-(Dodecyloxy)-3,5-dimethylbenzyl)dimethylammonio)acetate (C12OBCb). White solid, yield (85%), 1H NMR (500 MHz, CDCl3) δ 0.89–0.91 (t, 3H, –CH3), 1.30–1.40 (m, 16H, –O–CH2–CH2–CH2–(CH2)8–CH3), 1.51–1.57 (m, 2H, –O–CH2–CH2–CH2–(CH2)8–CH3), 1.78–1.84 (m, 2H, –O–CH2–CH2–CH2–(CH2)8–CH3), 2.30 (s, 6H, Ar–CH3), 3.19 (s, 6H, –CH2–N–(CH3)2–CH2–COO−), 3.64 (s, 3H, –CH2–N–(CH3)2–CH2–COO−), 3.79–3.82 (t, 2H, –O–CH2–CH2–CH2–(CH2)8–CH3), 4.67 (s, 2H, Ar–CH2–N+(CH3)2–CH2–), 7.19 (s, 2H, meta-ArH). 13C NMR (125 MHz, CDCl3) δ 14.2, 16.2, 22.6, 27.0, 30.2, 30.4, 30.5, 30.5, 30.5, 31.2, 32.8, 51.0, 63.7, 67.3, 73.2, 124.2, 133.0, 134.3, 159.1, 168.8. HRMS (ESI+) m/z: calcd for C25H43NO3+ (M + H), 406.3315; found, 406.3309.
2.2.3.2. 2-((4-(Tetradecyloxy)-3,5-dimethylbenzyl)dimethylammonio)acetate (C14OB Cb). White solid, yield (91%), 1H NMR (500 MHz, CDCl3) δ 0.88–0.91 (t, 3H, –CH3), 1.30–1.40 (m, 20H, –O–CH2–CH2–CH2–(CH2)10–CH3), 1.51–1.57 (m, 2H, –O–CH2–CH2–CH2–(CH2)10–CH3), 1.78–1.84 (m, 2H, –O–CH2–CH2–CH2–(CH2)10–CH3), 2.30 (s, 6H, Ar–CH3), 3.19 (s, 6H, –CH2–N–(CH3)2–CH2–COO−), 3.64 (s, 3H, –CH2–N–(CH3)2–CH2–COO−), 3.79–3.81 (t, 2H, –O–CH2–CH2–CH2–(CH2)10–CH3), 4.67 (s, 2H, Ar–CH2–N+ (CH3)2–CH2–), 7.19 (s, 2H, meta-ArH). 13C NMR (125 MHz, CDCl3) δ 14.2, 16.2, 23.5, 27.0, 30.2, 30.4, 30.5, 30.5, 30.5, 30.6, 31.2, 32.8, 51.0, 63.7, 67.3, 73.2, 124.2, 133.0, 134.3, 159.1, 168.8. HRMS (ESI+) m/z: calcd for C27H47NO3+ (M + H), 434.3628; found, 434.3630.
2.2.3.3. 2-((4-(Hexadecyloxy)-3,5-dimethylbenzyl)dimethylammonio)acetate (C16OB Cb). White solid, yield (88%), 1H NMR (500 MHz, CDCl3) δ 0.88–0.91 (t, 3H, –CH3), 1.29–1.41 (m, 24H, –O–CH2–CH2–CH2–(CH2)12–CH3), 1.51–1.57 (m, 2H, –O–CH2–CH2–CH2–(CH2)12–CH3), 1.78–1.84 (m, 2H, –O–CH2–CH2–CH2–(CH2)12–CH3), 2.29 (s, 6H, Ar–CH3), 3.18 (s, 6H, –CH2–N–(CH3)2–CH2–COO−), 3.64 (s, 3H, –CH2–N–(CH3)2–CH2–COO−), 3.79–3.81 (t, 2H, –O–CH2–CH2–CH2–(CH2)12–CH3), 4.67 (s, 2H, Ar–CH2–N+ (CH3)2–CH2–), 7.18 (s, 2H, meta-ArH). 13C NMR (125 MHz, CDCl3) δ 14.2, 16.2, 23.5, 27.0, 30.2, 30.4, 30.5, 30.5, 30.5, 30.6, 31.2, 32.8, 51.0, 63.7, 67.3, 73.2, 124.2, 133.0, 134.3, 159.1, 168.8. HRMS (ESI+) m/z: calcd for C29H51NO3+ (M + H), 462.3941; found, 462.3942.
2.2.3.4. 2-((4-(Octadecyloxy)-3,5-dimethylbenzyl)dimethylammonio)acetate (C18OB Cb). White solid, yield (83%), 1H NMR (500 MHz, CDCl3) δ 0.88–0.91 (t, 3H, –CH3), 1.29–1.40 (m, 28H, –O–CH2–CH2–CH2–(CH2)14–CH3), 1.51–1.57 (m, 2H, –O–CH2–CH2–CH2–(CH2)14–CH3), 1.78–1.84 (m, 2H, –O–CH2–CH2–CH2–(CH2)14–CH3), 2.30 (s, 6H, Ar–CH3), 3.18 (s, 6H, –CH2–N–(CH3)2–CH2–COO−), 3.64 (s, 3H, –CH2–N–(CH3)2–CH2–COO−), 3.79–3.81 (t, 2H, –O–CH2–CH2–CH2–(CH2)14–CH3), 4.67 (s, 2H, Ar–CH2–N+ (CH3)2–CH2–), 7.18 (s, 2H, meta-ArH). 13C NMR (125 MHz, CDCl3) δ 14.2, 16.3, 23.5, 27.0, 30.3, 30.4, 30.5, 30.5, 30.5, 30.6, 31.2, 32.8, 51.0, 63.7, 67.3, 73.2, 124.2, 133.0, 134.3, 159.1, 168.7. HRMS (ESI+) m/z: calcd for C31H55NO3+ (M + H), 490.4254; found, 490.4258.
2.3. Measurements
2.3.1. Thermal stability measurements. The thermostability of CnOBCb (n = 12, 14, 16, or 18) was investigated using a HTG–3 thermogravimetric analyzer (Beijing Henven Scientific Instrument Factory, China). The sample was heated from 10 to 600 °C with a heating rate of 10 °C min−1 in a nitrogen atmosphere at a flow rate of 50 mL min−1.
2.3.2. Surface tension measurements. The surface tensions of aqueous surfactant solutions were measured with a BZY–2 automatic tensiometer by using the Wilhelmy plate technique at 25.0 ± 0.1 °C. To obtain equilibrium surface tension, sets of measurements were taken until the change in surface tension was less than 0.01 mN m−1 every 3 min. The cmc and surface tension at the cmc (γcmc) were determined from the break point of the surface tension and the logarithm of the concentration curve (γ − log
C), respectively. The adsorption amount of surfactant Γ was calculated according to the Gibbs adsorption isotherm equation:32 |
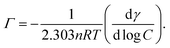 | (1) |
where γ is the surface tension in mN m−1, Γ is the adsorbed amount in mol m−2, R is the gas constant (8.314 J mol−1 K−1), T is the absolute temperature in K, C is the surfactant concentration, and (dγ/d
log
C) is the slope below the cmc in the surface tension plot. The value of n, which dependents theoretically on the surfactant type and structure, is taken as 1 for a zwitterionic surfactant in aqueous solution.33 The occupied area per surfactant molecule at the cmc, Acmc (nm2), is obtained from the saturation adsorption (Γcmc) using the following equation: |
 | (2) |
where N is Avogadro's number (6.022 × 1023 mol−1) and, Γcmc is the surface excess concentration at the cmc.
2.3.3. Interfacial tension measurements. The interfacial tension between toluene (or n-heptane) and aqueous surfactant solution was measured using a TX–500C spinning drop interfacial tension meter (Shanghai Zhongchen, China) at 25.0 ± 0.1 °C. The rotation speed was set at 12 rps in all measurements. Interfacial tension values were recorded until equilibrium was reached.
2.3.4. Steady-state fluorescence measurements. The fluorescence measurements were performed using a RF5301 fluorescence spectrophotometer (Shimadzu Corporation, Japan). The spectra were recorded between 350 and 500 nm with an excitation wavelength of 335 nm, and the excitation and emission slit widths were fixed at 3.0 nm. The concentration of pyrene in each solution was 1 × 10−6 mol dm−3. The fluorescence intensity ratio of the first to the third vibronic peaks, I1/I3, were used to estimate the micropolarity in its solubilization site.34
2.3.5. Dynamic light scattering (DLS). DLS measurements were performed with a Nano ZS Zetasizer (Malvern Instruments Laboratory, UK) equipped with a Helium–Neon laser source operating at λo = 632.8 nm. The scattering intensity was measured at an angle of 173° to the incident beam. The autocorrelation function was analyzed using the CONTIN method. The apparent hydrodynamic diameter (Dh) was obtained according to the Stokes–Einstein equation,25,27 Dh = kT/(3πηD), where D is the diffusion coefficient, k is the Boltzmann constant, T is the absolute temperature, and η is the viscosity of the solution. All sample solutions were filtered through a 0.45 μm membrane filter and measured three times at 25 °C.
2.3.6. Transmission electron microscopy (TEM). TEM measurements were performed on a JEM–2100 transmission electron microscope (JEOL Ltd., Japan) at an accelerating voltage of 200 kV. The TEM samples were prepared using the negative-staining method with phosphotungstic acid aqueous solution (2%). A droplet of the sample solution was spread on a 300-mesh copper grid coated with a Formvar film, and excess sample solution was wicked away with a piece of filter paper. When the grid was partially dried, a drop of staining solution was added onto the grid over 10 min. After drying, the morphologies of the samples were examined by TEM.
2.3.7. Core flooding experiment. Surfactant flooding experiments were performed in the artificial sandstone core at the oil reservoir temperature of 40 °C. To begin with, the porosity and permeability of the sandstone core were determined using an Automated Permeameter–Porosimeter (Coretest systems, Inc., U.S.A), and the basic parameters of sandstone core were given in Table 3. The core plug was loaded into the core holder and vacuumed for 2 h. After the core was saturated with formation brine (the composition of brine was listed in Table S2†), the crude oil was injected into core plug until the water cut reached less than 1%. After that, the core was aged in the core holder for 24 h and water flooding was conducted at a flow rate of 0.4 mL min−1 until the water cut reached 98%. Finally, 0.3 PV surfactant slug was injected into the core after water flooding and then subsequent water flooding was conducted until water cut reached 98%. The produced fluid was collected using a measuring cylinder to record the oil and water production.
3. Results and discussion
3.1. Thermogravimetric analysis (TGA)
The thermostability of CnOBCb (n = 12, 14, 16, or 18) was determined through TGA to evaluate the temperature range in which these surfactants can be applied. The TGA curves of CnOBCb are shown in Fig. 1. The results show that the weight of CnOBCb remains essentially unchanged below 200 °C. As the temperature increases, the compounds gradually decompose and then totally break down at approximately 500 °C. These findings indicate that CnOBCb (n = 12, 14, 16, or 18) exhibit excellent thermostability and can be utilized at temperatures up to 200 °C. Additionally, changing the hydrocarbon chain length did not significantly affect the onset temperature of thermal decomposition. It is also worth mentioning that the thermal decomposition temperatures of CnOBCb are higher than that of N-dodecyl-N,N-dimethyl-2-ammonio-1-ethanecarbonate (C12Cb, C12H25N+(CH3)2CH2COO−; 160 °C).35 Thus, C12OBCb shows better thermal stability than the corresponding linear betaine-type surfactants and the introduction of a phenyl group in the surfactant molecule can significantly increase the thermal stability of betaine-type surfactants.
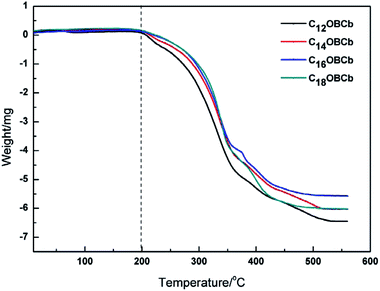 |
| Fig. 1 TGA curves of CnOBCb. | |
3.2. Equilibrium surface tension
The surface tension is plotted as a function of concentration of CnOBCb (n = 12, 14, 16, or 18) in Fig. 2. The surface tension of CnOBCb initially decreases as the surfactant concentration increases, and then reaches an equilibrium value. The break points of the curves were taken as cmc values. The cmc, γcmc, Γcmc, and Acmc values of CnOBCb are listed in Table 1, along with the data for CnCb (CnH2n+1N+(CH3)2–CH2COO−) for comparison.36 The variation of the cmc with the hydrocarbon chain length for homologous straight-chain ionic surfactants can be described by the empirical Klevens equation37 as follows: |
log cmc = A − Bn
| (3) |
where n is the number of carbon atoms in the hydrocarbon chain. The A value is a constant for a particular ionic head at a given temperature, whereas the B value is close to 0.3 for anionic and cationic surfactants and 0.5 for zwitterionic and nonionic surfactants. The relationships between the cmc and the hydrocarbon chain length of CnOBCb and CnCb are plotted in Fig. 3. For the CnCb series, the logarithm of the cmc decreases linearly as the hydrocarbon chain length increases. However, for CnOBCb, a similar trend is observed for hydrocarbon chain lengths of 12–16 and a deviation from linearity occurs when the hydrocarbon chain length reaches 18. This phenomenon has also been observed in some gemini zwitterionic surfactants.38,39 A reasonable explanation, as reported by Mukerjee,40 is that the cmc only changes slightly as the hydrocarbon chain length increases up to 18 because the long hydrocarbon chains coil in aqueous solution. The B value of CnOBCb with hydrocarbon chain lengths from 12 to 16 is determined as 0.47, which is close to those for zwitterionic betaine surfactants such as sulfobetaine-type surfactants (CnSb; B = 0.48)41 and CnCb (B = 0.49).36 It is also noteworthy that the cmc values of CnOBCb (n = 12, 14, or 16) are approximately one order of magnitude lower than those of CnCb with the same hydrocarbon chain lengths. Thus, the phenyl-containing CnOBCb surfactants have a better micellization ability at relatively low concentrations. This behavior is attributed to an enhancement of hydrophobic interactions caused by the introduction of a phenyl group near the headgroup.25,42
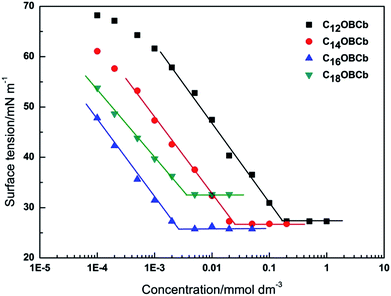 |
| Fig. 2 Variation of the surface tension with the surfactant concentration for CnOBCb at 25 °C. | |
Table 1 Surface activity parameters of carboxyl betaine surfactants CnOBCb
Surfactant |
cmc (mmol dm−3) |
γcmc (mN m−1) |
106 Γcmc (mol m−2) |
Acmc (nm2) |
C12OBCb |
0.1881 |
27.3 |
2.63 |
0.63 |
C14OBCb |
0.0229 |
26.7 |
2.66 |
0.62 |
C16OBCb |
0.0023 |
25.6 |
2.76 |
0.60 |
C18OBCb |
0.0035 |
32.6 |
2.33 |
0.71 |
C12H25N+(CH3)2CH2COO− |
1.8 |
36.5 |
3.57 |
0.47 |
C14H29N+(CH3)2CH2COO− |
0.18 |
37.5 |
3.55 |
0.47 |
C16H33N+(CH3)2CH2COO− |
0.02 |
39.7 |
4.13 |
0.40 |
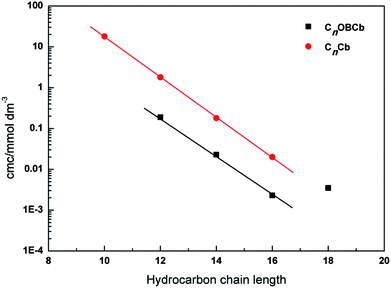 |
| Fig. 3 Relationship between the cmc and hydrocarbon chain length for CnOBCb and N-alkylbetaine surfactants CnCb. | |
For n = 12, 14, and 16, the γcmc values of CnOBCb are much smaller than those of CnCb (36.5–39.7 mN m−1 at 23 °C), indicating that CnOBCb has a greater efficiency in lowering the surface tension of water than CnCb. Meanwhile, the γcmc values of CnOBCb decrease on increasing the hydrocarbon chain length from 12 to 16. However, for n = 18, the γcmc value is 32.63 mN m−1, which is slightly larger than those of other homogeneous surfactants. This aberrant behavior has also been observed for ultra-long unsaturated amidobetaine surfactants,43 cationic gemini surfactants,39,44 and zwitterionic heterogemini surfactants.22 This phenomenon can be attributed to the fact that a long hydrophobic chain (n = 18) increase the steric hindrance between surfactant molecules, which makes surfactant molecules prone to forming premicellar aggregates in aqueous solution rather than adsorbing at the air/water interface.43
3.3. Interfacial tension measurements
The interfacial tension as a function of CnOBCb concentration in the range of 0.05–1 mmol dm−3 against toluene and n-heptane are illustrated in Fig. 4(a) and (b), respectively. The CnOBCb surfactants exhibit similar trends against both toluene and n-heptane, that is, the interfacial tensions decrease almost linearly with increasing surfactant concentrations in the investigated range. For the toluene/water interface, C12OBCb exhibits the lowest interfacial tension among the CnOBCb species, reaching an ultralow value of 10−3 mN m−1 in the concentration range of 0.2–1 mmol dm−3, which indicates excellent interfacial activity. For the n-heptane/water interface, C16OBCb exhibits the lowest interfacial tension, reaching a value of 0.24 mN m−1 at 1 mmol dm−3 (Fig. 4(b)). For both the toluene/water and n-heptane/water interfaces, C18OBCb shows the lowest capability for reducing the interfacial tension. This behavior is probably due to the longer hydrophobic chains in C18OBCb being prone to curl, leading to a loose arrangement of molecules at the interface. Thus, the concentration of effective surfactant molecules decreases in comparison with those of other homogenous CnOBCb species. Interestingly, it is noted that the interfacial tension values in toluene/water for CnOBCb (n = 12, 14, 16, or 18) are lower than those in n-heptane/water at the same concentrations. It can be deduced that the CnOBCb surfactants exhibit much higher efficiency in reducing interfacial tension against arenes than n-alkanes with the same number of carbon atoms in the molecular structure.
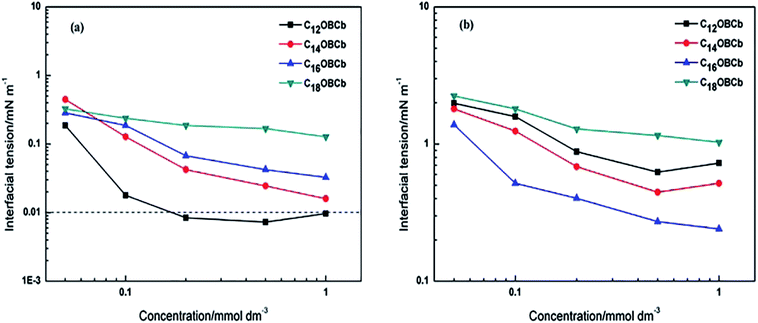 |
| Fig. 4 Variation of the interfacial tension with CnOBCb concentration against toluene (a) and n-heptane (b) at 25 °C. | |
3.4. Adsorption properties
The Acmc, which is closely correlated with the Γcmc, can provide information about the orientation and packing degree of surfactant molecules at the air/water interface. The Acmc values of CnOBCb, listed in Table 1, range from 0.60 to 0.71 nm2, which are slightly larger than those of CnCb.36 This difference may be caused by the bulky phenyl group within the hydrophobic tail. Meanwhile, the Acmc values decrease marginally on increasing the hydrocarbon chain length from 12 to 16, and then increase abruptly for n = 18. The initial decrease of Acmc reflects that tight packing of CnOBCb molecules at the air/water interface owing to the enhancement of hydrophobic interactions between the hydrocarbon chains. It seems that the presence of a rigid phenyl group in the hydrophobic tail favors an extended orientation of the hydrophobic chain, resulting in close packing of the surfactant molecules at the air/water interface.25 Further, π–π interactions between adjacent phenyl groups may drive more compact packing attract CnOBCb molecules to in the surface monolayer.24 Notably, the Acmc value of C18OBCb is slightly larger than those of other homogeneous surfactants, which indicates that the hydrophobic tails are not vertical to the surface, likely resulting from coiling of the longer hydrophobic chains.45,46
The efficiency and effectiveness of surfactant adsorption at the air/water interface can be characterized by the logarithm of the surfactant concentration C20 at which the surface tension of water is reduced by 20 mN m−1 (pC20) and by the cmc/C20 ratio,47 respectively. The pC20 and cmc/C20 ratio values of CnOBCb are shown in Table 2 together with the data of CnCb for comparison. The pC20 values of CnOBCb increase as the hydrocarbon chain length increases from 12 to 16 and are larger than those of CnCb with the same hydrocarbon chain lengths (pC20 = 3.91–5.54 (ref. 48)), suggesting that the CnOBCb surfactants have a greater tendency to adsorb at air/water interface. However, for C18OBCb, the pC20 value becomes smaller, possibly because of premicellar aggregation in solution. Further, the variation of the cmc/C20 ratio values of CnOBCb with the hydrocarbon chain length follows the same trend as the pC20 values, and the cmc/C20 ratio values of CnOBCb are approximately four times larger than those of CnCb (cmc/C20 = 6.5–7.5 (ref. 48)). This finding suggests that it is more beneficial for CnOBCb molecules to absorb at the air/water interface than to form micelles in the surfactant solution.
Table 2 Parameters on micellization and adsorption of carboxyl betaine surfactants CnOBCb
Surfactant |
pC20 |
cmc/C20 |
ΔG0mic (kJ mol−1) |
ΔG0ads (kJ mol−1) |
C12OBCb |
5.13 |
25.4 |
−31.20 |
−46.82 |
C14OBCb |
6.06 |
26.3 |
−36.42 |
−52.03 |
C16OBCb |
7.07 |
27.1 |
−42.12 |
−57.60 |
C18OBCb |
6.63 |
15.1 |
−41.08 |
−56.40 |
C12H25N+(CH3)2CH2COO− |
3.91 |
6.5 |
— |
— |
C14H29N+(CH3)2CH2COO− |
4.62 |
7.5 |
— |
— |
C16H33N+(CH3)2CH2COO− |
5.54 |
6.9 |
— |
— |
According to the single-stage equilibrium model, the standard free energy of micellization (ΔG0mic) and adsorption (ΔG0ads) for zwitterionic surfactants in aqueous solution can be calculated using the following equations:49,50
|
ΔG0mic = RT ln Xcmc
| (4) |
|
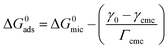 | (5) |
where
R is the gas constant (8.314 J mol
−1 K
−1),
T is the absolute temperature,
Xcmc is the cmc as a molar fraction (
Xcmc = cmc/55.4),
γ0 and
γcmc are the surface tensions of water and surfactant solution at the cmc, respectively. Because the micellization of C
nOBCb is a spontaneous process, the calculated Δ
G0mic values should be negative. The Δ
G0mic and Δ
G0ads values of C
nOBCb (
n = 12, 14, 16, or 18) are tabulated in
Table 2. Based on these results, the Δ
G0ads value of C
nOBCb is more negative than its Δ
G0mic value, signifying that the adsorption process is more favorable than micellization. This finding is also supported by the
pC20 and cmc/
C20 ratio values of C
nOBCb being larger than those of C
nCb. In addition, the negative values of Δ
G0mic and Δ
G0ads become larger on increasing the hydrocarbon chain length from 12 to 16, but are slightly smaller when the hydrocarbon chain length reaches 18. This phenomenon suggests that a driving force for micellization or adsorption is derived from the hydrophobic effect among hydrocarbon chains
51 and the deviation may be due to the stereo inhibition of the longer hydrocarbon chain.
36
3.5. Micropolarity and micellization
The micropolarity and micellization of CnOBCb (n = 12, 14, 16, or 18) were investigated using pyrene fluorescence and DLS measurements. In general, the scattering intensity obtained from DLS measurements increases as increasing the size and number of micelles increase. In fluorescence measurement, the I1/I3 ratio decreases from approximately 1.8 to 1.2 when micelles are formed, owing to the transfer of pyrene molecules from the water environment into the interior hydrophobic region of micelles.15 The variations of I1/I3 ratio (the fluorescence spectra of CnOBCb are shown in the Fig. S13–S16†) and the scatting intensity as a function of CnOBCb (n = 12, 14, 16, or 18) concentration are shown in Fig. 5. The I1/I3 ratio starts to decrease and the scattering intensity increases at a concentration close to the corresponding cmc. These results indicate that aggregates are formed at these concentrations in aqueous solution. Additionally, the I1/I3 ratios of CnOBCb with n = 12, 14, 16 and 18 gradually decrease with increasing surfactant concentration. This trend is similar to the fluorescence results for heterogemini surfactants reported by Yoshimura et al.36 who suggested that this phenomenon is probably due to a broad micelle size distribution. It is also worth noting that the minimum values of the I1/I3 ratio at high concentrations above the cmc are similar for the four CnOBCb surfactants, suggesting that the hydrophobic length of the surfactant molecules has little effect on the I1/I3 ratio values. This result is also in good agreement with the behavior of conventional surfactants.52,53
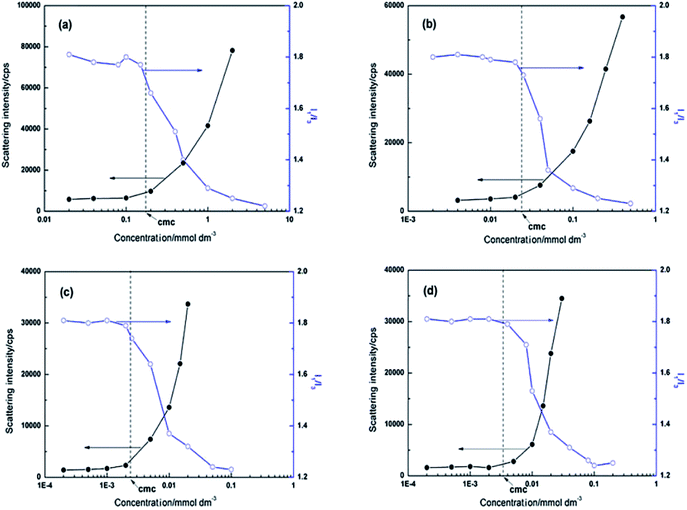 |
| Fig. 5 Variation of the pyrene fluorescence intensity ratio I1/I3 and scattering intensity by DLS with the surfactant concentration for CnOBCb: n = (a) 12, (b) 14, (c) 16, (d) 18. | |
3.6. Size and morphology of aggregates
To investigate the effect of the CnOBCb concentration on the size and morphology of aggregates in aqueous solution, DLS and TEM measurements were performed. The apparent hydrodynamic diameters (Dh) for CnOBCb (n = 12, 14, 16, or 18) were determined by DLS over a wide concentration range from 5 × cmc to 20 × cmc. As shown in Fig. 6, CnOBCb show a bimodal distribution containing two peaks with a wide range of Dh values from 10 to 200 nm. As the light scattering intensity is proportional to the sixth power of the diameter of the particles, the major populations of aggregates are relatively small ones in the surfactant solution. The Dh values of aggregates of CnOBCb (n = 12, 14, 16, or 18) slightly increase with increasing surfactant concentrations, which implies that the small aggregates existing at low surfactant concentrations can gradually transform into large aggregates as the surfactant concentration increases. This trend is similar to the results obtained for sulfatebetaine zwitterionic gemini surfactants.54
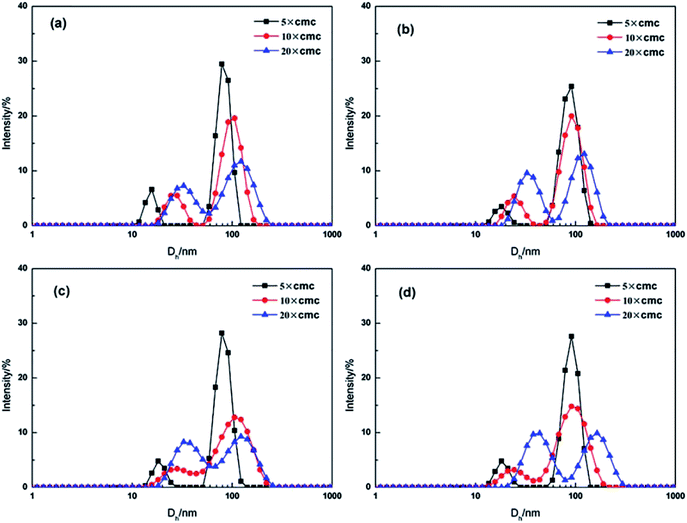 |
| Fig. 6 Variation of size distribution with the surfactant concentration by DLS for CnOBCb: n = (a) 12, (b) 14, (c) 16, (d) 18. | |
To directly visualize of the morphologies of the CnOBCb aggregates, TEM measurements were conducted at the concentrations used for DLS measurements. TEM images of CnOBCb at different concentrations (Fig. 7) clearly exhibit the presence of closed vesicles in aqueous solution. The vesicles size determined from TEM measurements are consistent with the DLS results. For C12OBCb and C14OBCb, predominant small spherical micelles and several large vesicles with average diameter about 15 and 80 nm, respectively, were observed at low concentrations (5 × cmc) (Fig. 7(a) and (d)). On further increasing the surfactant concentration to 10 × cmc, the small micelles grow into partially elongated vesicles and larger spherical vesicles (Fig. 7(b) and (e)), consistent with the higher polydisperse index (PDI) value obtained by DLS. The elongated vesicles are 50–80 nm long with diameters of 5 nm. The growth of spherical micelles into partially elongated micelles has been reported for ionic liquids containing an imidazolium ring,54 this may be caused by the stronger hydrophobicity imparted by the presence of a phenyl group within the long hydrocarbon chain. When the concentration increases to 20 × cmc, the sizes of the small and large vesicles are centered at 35 and 110 nm, respectively. Surprisingly, the formation of elongated vesicles was not observed in for C16OBCb and C18OBCb (Fig. 7(h) and (k)), and only spherical micelles or elliptical vesicles were formed within the investigated concentration ranges. The Cryo-TEM experiments for CnOBCb (n = 12, 14, 16, 18) at the concentrations of 20 × cmc were carried out and the Cryo-TEM images were shown in Fig. 8. The formation of spherical particles was also observed for all of these four surfactants from cryo-TEM images whose dimension matches closely with DLS and TEM data.
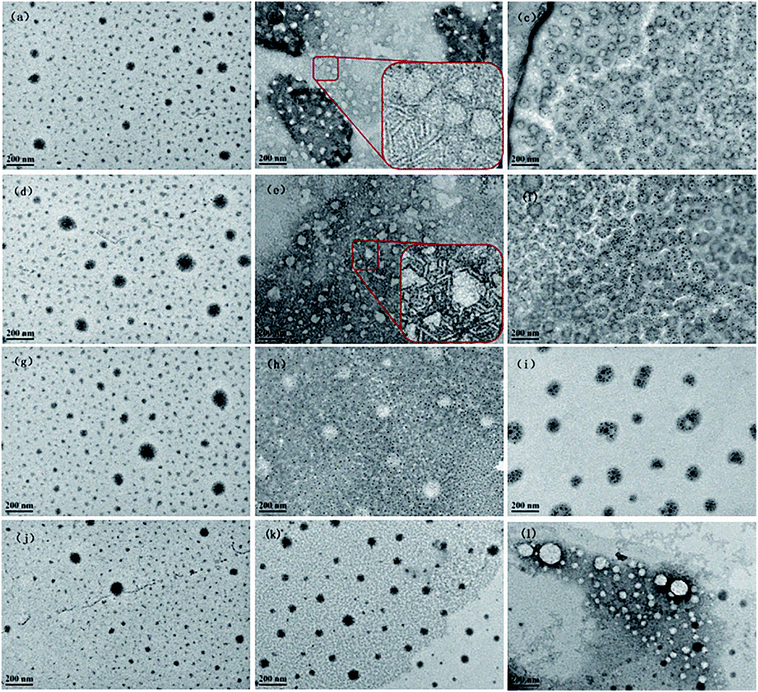 |
| Fig. 7 TEM images of CnOBCb (n = 12, 14, 16 and 18) aggregates: C12OBCb at (a) 0.94 mmol dm−3 (5 × cmc), (b) 1.88 mmol dm−3 (10 × cmc), (c) 3.76 mmol dm−3 (20 × cmc); C14OBCb at (d) 0.114 mmol dm−3 (5 × cmc), (e) 0.229 mmol dm−3 (10 × cmc), (f) 0.458 mmol dm−3 (20 × cmc); C16OBCb at (g) 0.0115 mmol dm−3 (5 × cmc), (h) 0.023 mmol dm−3 (10 × cmc), (i) 0.046 mmol dm−3 (20 × cmc); C18OBCb at (j) 0.0175 mmol dm−3 (5 × cmc), (k) 0.035 mmol dm−3 (10 × cmc), (l) 0.07 mmol dm−3 (20 × cmc). | |
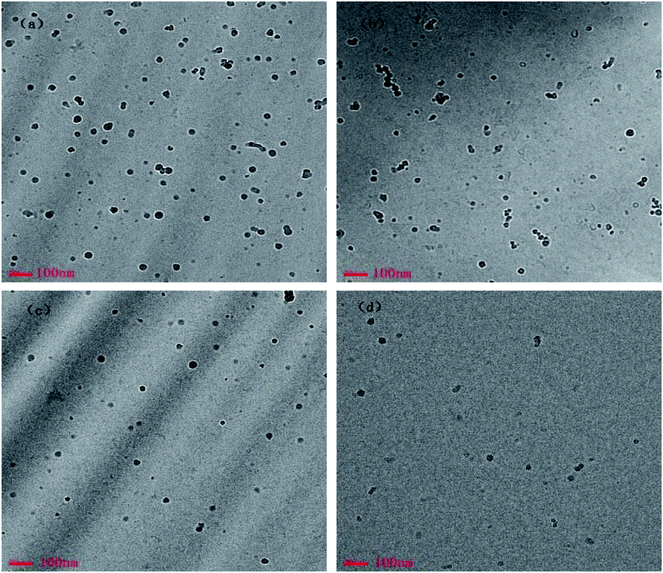 |
| Fig. 8 Cryo-TEM images of vesicles formed at 20 × cmc for (a) C12OBCb (3.76 mmol dm−3), (b) C14OBCb (0.458 mmol dm−3), (c) C16OBCb (0.046 mmol dm−3), and (d) C18OBCb (0.07 mmol dm−3). | |
Moreover, optical microscope by OLYMPUS BX51 Fluorescence Microscopy was performed to investigate encapsulation ability of this new class of surfactants. The fluorescent image for C12OBCb solution (3.76 mmol dm−3) dyed with calcein is shown in Fig. S17.† It can be seen that calcein was well encapsulated into the vesicles. The diameter of small vesicles is a few tens of nanometers, which is also consistent with the DLS and TEM results.
3.6. Core flooding experiment
The capability of enhancing oil recovery for the C12Cb and CnOBCb (n = 12, 14, 16, 18) solution was evaluated in sandstone core, and the results of the surfactant flood ing were summarized in Table 3. The displacement efficiency of CnOBCb (n = 12, 14, 16, 18) is slightly higher than that of C12Cb (6.73%) at the same surfactant concentration (Fig. 9). C12OBCb enhanced an additional oil recovery of 10.55%, which was the highest among the CnOBCb species. The relatively higher oil recovery for CnOBCb surfactants could be attributed to the excellent interfacial tension properties.
Table 3 Core parameters and EOR of C12Cb and CnOBCb (n = 12, 14, 16, 18)
Core no. |
Length (cm) |
Diameter (cm) |
Porosity (%) |
Permeability (mD) |
Sample (0.5 mmol dm−3) |
E1 (%) |
E2 (%) |
EOR (%) |
1# |
7.162 |
2.520 |
26.45 |
132.65 |
C12Cb |
48.54 |
55.27 |
6.73 |
2# |
7.228 |
2.524 |
25.82 |
126.46 |
C12OBCb |
48.89 |
59.44 |
10.55 |
3# |
7.218 |
2.518 |
26.15 |
134.60 |
C14OBCb |
47.03 |
56.11 |
9.08 |
4# |
7.224 |
2.516 |
25.87 |
137.22 |
C16OBCb |
48.49 |
57.73 |
9.24 |
5# |
7.216 |
2.520 |
25.80 |
134.81 |
C18OBCb |
48.41 |
56.26 |
7.85 |
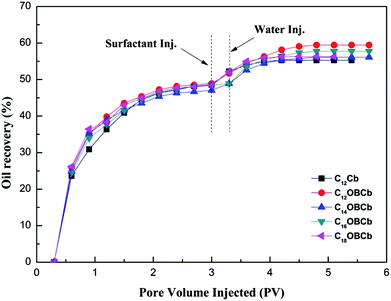 |
| Fig. 9 Oil recovery as a function of pore volume injected (PV) for C12Cb and CnOBCb (n = 12, 14, 16, 18). | |
4. Conclusions
A novel series of phenyl-containing carboxybetaine surfactants were successfully prepared by an efficient and high-yield synthesis route and characterized by 1H NMR, 13C NMR, and ESI-HRMS. The TGA results showed that CnOBCb was stable and difficult to decompose below 200 °C. CnOBCb exhibited superior surface activities, including lower cmc (0.1881–0.0023 mmol dm−3), higher efficiency in lowering the surface tension of water (25.6–32.6 mN m−1) and stronger adsorption tendency at the air/water interface, in comparison with CnCb with the same hydrocarbon chain length. CnOBCb also showed excellent interfacial tension activity against toluene, with C12OBCb reaching ultralow interfacial tension (10−3 mN m−1) in the range of 0.2–1 mmol dm−3. The DLS and TEM results showed that the CnOBCb could spontaneous aggregate into vesicles, and the sizes of the aggregates increase with increasing surfactant concentrations. In particular, CnOBCb with n = 12 and 14 aggregated to form elongated micelles at 10 × cmc. From these results, it could be concluded that the presence of a phenyl group in the hydrophobic chain has a dramatic effect on the surface–active properties and aggregation behavior of betaine-type surfactants. Moreover, core flooding experiments directly demonstrate that CnOBCb could remarkably enhance oil recovery by 7.85–10.55%.
Conflicts of interest
There are no conflicts to declare.
Acknowledgements
We are grateful for the financial supports from the National Natural Science Foundation of China (Grants No. 51474223).
References
- V. Seredyuk, E. Alami, M. Nydén and K. Holmberg, Langmuir, 2001, 17, 5160–5165 CrossRef CAS.
- K. Singh and D. G. Marangoni, J. Colloid Interface Sci., 2007, 315, 620–626 CrossRef CAS PubMed.
- D. Wieczorek, A. Dobrowolski, K. Staszak, D. Kwaśniewska and P. Dubyk, J. Surfactants Deterg., 2017, 20, 151–158 CrossRef CAS PubMed.
- S. S. Hu, L. Zhang, Z. C. Xu, Q. T. Gong, Z. Q. Jin, L. Luo, L. Zhang and S. Zhao, Appl. Surf. Sci., 2015, 355, 868–877 CrossRef CAS.
- B. L. Song, X. Hu, X. Q. Shui, Z. G. Cui and Z. J. Wang, Colloids Surf., A, 2016, 489, 433–440 CrossRef CAS.
- Z. G. Cui, D. Qi, B. l. Song, X. M. Pei and X. Hu, Energy Fuels, 2016, 30, 2043–2051 CrossRef CAS.
- S. Alzobaidi, C. Da, V. Tran, M. Prodanović and K. P. Johnston, J. Colloid Interface Sci., 2017, 488, 79–91 CrossRef CAS PubMed.
- T. Tamuraa, T. Iiharab, S. Nishidab and S. Ohtab, J. Surfactants Deterg., 1997, 2, 207–211 CrossRef.
- I. Bozetine, T. A. Zaïd, C. E. Chitour and J. P. Canselier, J. Surfactants Deterg., 2008, 11, 299–305 CrossRef CAS.
- R. Mostafalul, A. Banaei and F. Ghorbani, J. Surfactants Deterg., 2015, 15, 919–922 CrossRef.
- N. C. Christov, N. D. Denkov, P. A. Kralchevsky, K. P. Ananthapadmanabhan and A. Lips, Langmuir, 2004, 20, 565–571 CrossRef CAS PubMed.
- B. L. Song, X. Hu, X. Q. Shui, Z. G. Cuia and Z. J. Wang, Colloids Surf., A, 2016, 489, 433–440 CrossRef CAS.
- V. T. Kelleppan, J. E. Moore, T. M. McCoy, A. V. Sokolova, L. de Campo, B. L. Wilkinson and R. F. Tabor, Langmuir, 2018, 34, 970–977 CrossRef CAS PubMed.
- K. Nyuta, T. Yoshimura and K. Esumi, J. Colloid Interface Sci., 2006, 301, 267–273 CrossRef CAS PubMed.
- T. Yoshimura, T. Ichinokawa, M. Kaji and K. Esumi, Colloids Surf., A, 2006, 273, 208–212 CrossRef CAS.
- J. H. Zhao, C. L. Dai, Q. F. Ding, M. Y. Du, H. S. Feng, Z. Y. Wei, A. Chen and M. W. Zhao, RSC Adv., 2015, 5, 13993–14001 RSC.
- L. Y. Hou, H. N. Zhang, H. Chen, Q. B. Xia, D. Y. Huang, L. Meng and X. F. Liu, J. Surfactants Deterg., 2013, 17, 403–408 CrossRef.
- M. Zhou, G. Luo, Z. Zhang, S. S. Li and C. W. Wang, J. Mol. Struct., 2017, 1144, 199–205 CrossRef CAS.
- D. Wieczorek, A. Dobrowolski, K. Staszak, D. Kwasniewska and P. Dubyk, J. Surfactants Deterg., 2017, 10, 151–158 CrossRef PubMed.
- R. A. Khalil and F. A. Saadoon, J. Saudi Chem. Soc., 2015, 19, 423–428 CrossRef.
- V. K. Sharma, S. Mitra, M. Johnson and R. Mukhopadhyay, J. Phys. Chem. B, 2013, 117, 6250–6255 CrossRef CAS PubMed.
- V. I. Martín, A. Rodríguez, M. M. Graciani, I. Robina, A. Carmona and M. L. Moyá, J. Colloid Interface Sci., 2011, 363, 284–294 CrossRef PubMed.
- Y. J. Li, J. Reeve, Y. L. Wang, R. K. Thomas, J. B. Wang and H. K. Yan, J. Phys. Chem. B, 2005, 109, 16070–16074 CrossRef CAS PubMed.
- K. Taleb, M. Mohamed-Benkada, N. Benhamed, S. Saidi-Besbes, Y. Grohens and A. Derdour, J. Mol. Liq., 2017, 241, 81–90 CrossRef CAS.
- M. A. Hegazy, M. Abdallah and H. Ahmed, Corros. Sci., 2010, 52, 2897–2904 CrossRef CAS.
- Q. Zhang, M. Z. Tian, Y. C. Han, C. X. Wu, Z. B. Li and Y. L. Wang, J. Colloid Interface Sci., 2011, 362, 406–414 CrossRef CAS PubMed.
- S. De, V. K. Aswal and S. Ramakrishnan, Langmuir, 2010, 26, 17882–17889 CrossRef CAS PubMed.
- S. S. Hu, Z. H. Zhou, L. Zhang, Z. C. Xu, Q. T. Gong, Z. Q. Jin, L. Zhang and S. Zhao, Soft Matter, 2015, 11, 7960–7968 RSC.
- Z. Y. Liu, Z. C. Xu, H. Zhou, Y. L. Wang, Q. Liao, L. Zhang and S. Zhao, J. Mol. Liq., 2017, 240, 412–419 CrossRef CAS.
- Q. Q. Zhang, B. X. Cai, H. Z. Gang, S. Z. Yang and B. Z. Mu, RSC Adv., 2014, 4, 38393–38396 RSC.
- L. F. Dong, X. L. Cao, Z. Q. Li, L. Zhang, Z. C. Xu, L. Zhang and S. Zhao, Colloids Surf., A, 2014, 444, 257–268 CrossRef CAS.
- M. J. Rosen, A. W. Cohen, M. Dahanayake and X. Y. Hua, J. Phys. Chem., 1982, 86, 541–545 CrossRef CAS.
- V. Seredyuk, E. Alami, M. Nydén and K. Holmberg, Langmuir, 2001, 17, 5160–5165 CrossRef CAS.
- K. Kalyanasundaram and J. K. Thomas, J. Am. Chem. Soc., 1977, 99, 2039–2044 CrossRef CAS.
- J. X. Shen, Y. Y Bai, Q. W. Yin, W. X. Wang, X. Y Ma and G. Y Wang, J. Ind. Eng. Chem., 2017, 56, 82–89 CrossRef CAS.
- T. Yoshimura, K. Nyuta and K. Esumi, Langmuir, 2005, 21, 2682–2688 CrossRef CAS PubMed.
- H. B. Klevens, J. Phys. Colloid Chem., 1948, 52, 130–148 CrossRef CAS PubMed.
- F. M. Menger, J. S. Keiper and V. Azov, Langmuir, 2000, 16, 2062–2067 CrossRef CAS.
- M. J. Rosen, J. H. Mathias and L. Davenport, Langmuir, 1999, 15, 7340–7346 CrossRef CAS.
- P. Mukerjee, Adv. Colloid Interface Sci., 1967, 1, 242–275 CrossRef.
- J. G. Weers, J. F. Rathman, F. U. Axe, C. A. Crichlow, L. D. Foland, D. R. Scheuing, R. J. Wiersema and A. G. Zielske, Langmuir, 1991, 7, 854–867 CrossRef CAS.
- J. Hoque, P. Akkapeddi, V. Yarlagadda, D. S. Uppu, P. Kumar and J. Haldar, Langmuir, 2012, 28, 12225–12234 CrossRef CAS PubMed.
- D. Feng, Y. M. Zhang, Q. S. Chen, J. Y. Wang, B. Li and Y. J. Feng, J. Surfactants Deterg., 2012, 15, 657–661 CrossRef CAS.
- F. M. Menger and C. A. Littau, J. Am. Chem. Soc., 1993, 115, 10083–10090 CrossRef CAS.
- B. Li, Q. Zhang, Y. Xia and Z. N. Gao, Colloids Surf., A, 2015, 470, 211–217 CrossRef CAS.
- L. M. Zhou, X. H. Jiang, Y. T. Li, Z. Chen and X. Q. Hu, Langmuir, 2007, 23, 11404–11408 CrossRef CAS PubMed.
- M. Q. Ao, G. Y. Xu, Y. Y. Zhu and Y. Bai, J. Colloid Interface Sci., 2008, 326, 490–495 CrossRef CAS PubMed.
- M. J. Rosen and J. T. Kunjappu, Surfactants and interfacial phenomena, Surfactants and interfacial phenomena, 4th edn, 2012. pp. 93–94 Search PubMed.
- X. Q. Wang, J. Liu, L. Yu, J. J. Jiao, R. Wang and L. M. Sun, J. Colloid Interface Sci., 2013, 391, 103–110 CrossRef CAS PubMed.
- Y. Chevalier, Y. Storet, S. Pourchet and P. L. Perchec, Langmuir, 1991, 7, 848–853 CrossRef CAS.
- T. Yoshimura and K. Esumi, J. Colloid Interface Sci., 2004, 276, 231–238 CrossRef CAS PubMed.
- K. Sakai, S. Umezawa, M. Tamura, Y. Takamatsu, K. Tsuchiya, K. Torigoe, T. Ohkubo, T. Yoshimura, K. Esumi, H. Sakai and M. Abe, J. Colloid Interface Sci., 2008, 318, 440–448 CrossRef CAS PubMed.
- B. Cai, X. F. Li, Y. Yang and J. F. Dong, J. Colloid Interface Sci., 2012, 370, 111–116 CrossRef CAS PubMed.
- G. Singh, G. Singh and T. S. Kang, J. Phys. Chem. B, 2016, 120, 1092–1105 CrossRef CAS PubMed.
Footnote |
† Electronic supplementary information (ESI) available. See DOI: 10.1039/c8ra06217j |
|
This journal is © The Royal Society of Chemistry 2018 |