DOI:
10.1039/C8RA06110F
(Paper)
RSC Adv., 2018,
8, 36858-36868
Enhanced adsorption behaviors of Co2+ on robust chitosan hydrogel microspheres derived from an alkali solution system: kinetics and isotherm analysis†
Received
18th July 2018
, Accepted 20th October 2018
First published on 31st October 2018
Abstract
Chitosan hydrogel microspheres derived from the LiOH/KOH/urea aqueous system demonstrate great characteristics of high mechanical strength, relative chemical inertness, renewability and 3-D fibrous network, making them promising functional supports. This work aims to investigate the tunable Co2+ adsorption behaviors on these robust chitosan microspheres in detail, providing the theoretical basis for optimizing the preparation procedure of chitosan microspheres supported Co3O4 catalysts in the future. The experimental results revealed that the fabricated original chitosan microspheres with more extended chain conformation could display enhanced adsorption capacity for Co2+ at determined concentration both in water and alcohol solutions, which is about 2–7 times higher than that of the conventional chitosan hydrogel microspheres prepared from the acetic acid solution. The kinetic experiments indicated that the adsorption process in water solution agreed with the pseudo-second-order kinetic equation mostly, while the chemical and physical adsorptions commonly contribute to the higher Co2+ adsorption on chitosan microspheres in alcohol solution. Moreover, in both cases, the film diffusion or chemical reaction is the rate limiting process in the initial adsorption stage, and the adsorption of Co2+ on chitosan microspheres can well fit to the Langmuir isotherm. Thermodynamic analysis demonstrated that such adsorption behaviors were dominated by an endothermic (ΔH° > 0) and spontaneous (ΔG° < 0) process.
1. Introduction
Cobalt-based materials have shown great applications in a wide range of fields, such as electrochromic devices, ceramic pigments, catalysts, solid-state sensors, magnetism, solar energy absorbers, energy storage, etc.1–4 As one of the important species, cobalt oxide is of special interest due to its excellent catalytic performance in various reactions.5–9 For example, Schüth et al. have demonstrated that immobilization of Co3O4 nanoparticles onto the mesoporous carbon could exhibit efficient catalytic action for transfer hydrogenation of α, β-unsaturated aldehydes with a selectivity higher than 95% at full conversion.10 Tüysüz's group revealed that Co3O4 clusters loaded in monodisperse mesoporous silica spheres showed a clear advantage over non-supported Co3O4 nanoparticles toward the photochemical water oxidation.11 Similar behavior was also reported, where mesoporous Co3O4 nanoparticles supported on mesoporous silica SBA-15 displayed enhanced efficiency in N2O decomposition relative to bare Co3O4.12 These findings clearly indicate that the catalysis is closely related to the carriers. The support matrixes with small pore size and high surface area not only facilitate the Co3O4 nanoparticles with smaller size, but also could prevent the catalyst from aggregation, ensuring the accessibility of the substrate to the catalytic active sites. Meanwhile, the carriers would enable an easy recovery and recycling of catalysts from the reaction mixture. Since the Co3O4 nanoparticles are usually prepared by introducing Co2+ as the precursor into the support matrix, the investigation of the adsorption kinetics and isotherm behaviors of Co2+ on support matrixes will have great significance on the development of catalyst supports.
As a popular natural polysaccharide, chitosan has been proved to be an eco-friendly transition metal ions support, owing to the characteristics of relative chemical inertness, strong affinity and sustainability. Benefitting from its abundant hydroxyl and amino groups, several transition metal ions could be anchored onto the chitosan matrix.13,14 Nevertheless, the fabricated single-component chitosan materials obtained from the traditional acid solution could display poor mechanical property. Thus, most of the investigation mainly focused the adsorption behaviors on the chitosan hybrid nanocomposites.15,16 In addition, native chitosan matrix does not exhibit high adsorption efficiency for the target metal ions. In order to improve the uptake efficiency for target adsorbates, various functional groups or cross-linkers (e.g. EDTA, EGTA, DTPA) have to be employed to the bio-derived chitosan adsorbents.17–20 All these occurrences lead to the limitation for chitosan as a stable heterogeneous catalysts support.21 The invention of a “green” solvent system consisting of alkali/urea has opened up an avenue to fabricate the supertough chitosan hydrogel materials.22 The obtained chitosan hydrogel with a nanofibrous structure could display higher mechanical strength than that prepared from traditional acidic solvent (about 100 times). As shown in Fig. S1,† the chitosan hydrogel beads fabricated from the alkaline system could sustain their original shape, while the hydrogel beads from the acetic acid solution could be easily broken into pieces under intense agitation. Hence, it is expected that this pure chitosan hydrogel-based material derived from alkaline solvent system would be an ideal and stable candidate for Co2+ support. In this work, robust chitosan microspheres were prepared from the LiOH/KOH/urea aqueous system by using the W/O emulsion method, with the simultaneous chemical crosslink to improve stability. Taking advantage of the 3-D fibrous network structures in microspheres and high surface area, Co2+ could be easily absorbed onto the chitosan nanofibers in matrix with enhanced adsorption capacity. Thus, the impacts of the solution pH, initial Co2+ concentration and incubation temperature on Co2+ adsorption onto chitosan hydrogel spheres in water and alcohol solution were investigated in detail, respectively. The related adsorption kinetics and isotherm behaviors were also explored. This work will provide the theoretical basis and guidance for the further preparation of chitosan microspheres supported Co3O4 catalyst.
2. Experimental
2.1 Materials
Chitosan powder with a degree of deacetylation about 89% was purchased from Ruji Biotechnology Co., Ltd. (Shanghai, China). All of the chemical reagents including KOH, LiOH·H2O, urea, NaOH, HCl, CoCl2, epichlorohydrin, chloroform, methylbenzene acetone anhydrous DMSO, alcohol and NH3·H2O were analytical grade from commercial sources in China. Tween-80, Span-80, glutaraldehyde, toluene and isooctane were supplied by Sigma-Aldrich Company. NaCl, EDTA·2Na and acetic acid were obtained from VWR International.
2.2 Fabrication of the robust chitosan microspheres
Robust chitosan microspheres derived from the alkali solvent system were prepared by utilizing a conventional emulsion method.23 Specifically, 4 g chitosan powder were dissolved in 100 g aqueous solution consisting of LiOH·H2O/KOH/urea/H2O with the weight ratio of 7.9
:
7:8
:
77.1 via a freezing-thawing method. The freezing-thawing process was allowed to repeat twice with the purpose of complete dissolution. Then, 2 mL epichlorohydrin as the chemical crosslinking reagent was added dropwise into the viscous chitosan solution and stirred at −20 °C for 2 h. After centrifugation at 4300 rpm for 5 min at 4 °C to remove the air bubbles, the homogeneous transparent chitosan pre-gel solution (120 mL) was swiftly added into the pre-mixed organic solvents containing 500 mL of isooctane and 5 mL Span-80 in a flask. Under an intense agitation at 1300 rpm for 2 h at room temperature, the gelated chitosan micro-droplets formed. Subsequently, the emulsion was transferred into 2 L alcohol/water solution (v/v = 7
:
3), the emulsion was broken and the chitosan microspheres were acquired. Finally, the microspheres were successively washed with ultrapure water and alcohol for three times to remove the residual chemicals. For the preparation of chitosan beads, the obtained pre-gel chitosan solution was allowed to drip into hot water by an injector to get the raw beads. After thorough washing with ultrapure water, the chitosan hydrogel beads were collected.
2.3 Fabrication of the conventional chitosan microspheres
The conventional chitosan microspheres from the acetic acid were fabricated via a W/O emulsification cross-linking reaction method.24 Briefly, certain amount of chitosan powder was firstly dissolved in 2% acetic acid to give a 3% chitosan solution. After centrifugation at 4300 rpm for 15 min at 4 °C to remove the undissolved precipitation, the obtained homogeneous transparent chitosan acid solution (100 mL), Tween-80 (2 mL), Span-80 (2 mL) and toluene (600 mL) were simultaneously added into a flask, allowing to stir mechanically for 1 h to form an emulsion. Then, 20% glutaraldehyde stock solution (1 mL) as the crosslinking agent was added into the emulsion system for incubating another 2 h at room temperature. With the following incubation in 0.1 mol L−1 NaOH aqueous solution for 1 h, the chitosan microspheres were acquired. Finally, the chitosan microspheres were successively washed with 70% alcohol solution and ultrapure water for three times to remove the residual chemicals. For the preparation of chitosan beads from the acid solution, the obtained pre-gel chitosan acid solution was allowed to drip into 0.1 mol L−1 hot NaOH aqueous solution by an injector to get the raw beads. After thorough washing with ultrapure water, the chitosan hydrogel beads were collected.
2.4 Adsorption behaviors studies
The adsorption behaviors of Co2+ on chitosan microspheres including the effects of pH and incubation temperature, adsorption isotherms, kinetic mechanisms and thermodynamic parameters, were investigated intensively. All the experiments were performed in 100 mL plastic tubes containing 50 mL of Co2+ solution by utilizing the batch equilibrium method. Meanwhile, all the tubes were sealed with the caps to avoid the adverse evaporation during the incubation process. In the adsorption isotherm, concentrations of Co2+ in each solution ranged from 0.5 to 10.0 mmol L−1 and the incubation temperature was set to be 293 K. To each container, 5 g chitosan microspheres were added. After incubation for 24 h, the final concentration of Co2+ in each beaker was determined with ICP-OES spectrometer. Thereby, the adsorption capacity, qe (mmol g−1), of Co2+ on chitosan microspheres could be calculated following the below equation:25 |
 | (1) |
where c0 and ce are the initial and final concentrations of Co2+ (mmol L−1), respectively, V is the total volume of Co2+ aqueous solution (L) in each tube and W is the mass of chitosan microspheres (g).
Kinetic behaviors for Co2+ adsorption on chitosan microspheres in water and alcohol solutions were investigated, respectively. For the kinetic studies in water solution, 5 g original microspheres were added to 50 mL Co2+ aqueous solution. For the investigation of kinetic behavior in alcohol solution, solvent exchange in the matrix was firstly performed by placing the chitosan microspheres in alcohol for 5 days. The alcohol was changed with the fresh one every 24 h. Then, 1 g samples were introduced to 50 mL Co2+ alcohol solution. Both the concentrations of the solutions were kept at 1.0 mmol L−1 with a fixed pH value of 7.0. At different time intervals, the final concentration of Co2+ in each container was analyzed by ICP-OES. To investigate the impact of pH on the Co2+ adsorption capacity, the pH values of Co2+ aqueous solution varied from 1.0 to 10.0 by adding NaOH or HCl diluted aqueous solutions. In order to explore the dependence of adsorption efficiency on the incubation temperature, the experimental tests were processed at the temperatures of 277, 293 and 310 K.
2.5 Desorption and regeneration studies
In order to evaluate the desorption and regeneration abilities, 5.0 g of chitosan microspheres were firstly placed in 50 mL of 10.0 mmol L−1 Co2+ aqueous solution. After incubation for 24 h at 293 K, the adsorbent and aqueous solution were collected, respectively, for the further experiments. The aqueous solution was used to determine the adsorption capacity and desorption efficiency. The chitosan microspheres were regenerated by using 10 mL of 0.1 mol L−1 EDTA as the static eluting solution for 3 times. Each static elution time was set to be 8 h. Then, the adsorbent was fully washed by 1.0 mol L−1 NaCl solution and deionized water for the succeeding adsorption performance. This adsorption–desorption cycle was allowed to repeat three times under the same condition.
2.6 Characterization
The surface morphologies of chitosan hydrogel microspheres before and after adsorption of Co2+ were characterized by using a field emission scanning electron microscope (SEM, SIRION TMP, FEI) at an accelerating voltage of 5 kV. The samples were prepared by immersing them in liquid nitrogen with subsequent freeze drying treatment. The existence and electronic states of cobalt element on chitosan microspheres were evaluated on an X-ray photoelectron spectroscopy (XSAM800, Kratos, UK) using monochromated Mg Kα (1253.6 eV) source at 16 mA × 12 kV. The exact concentration of Co2+ in solution was determined by the ICP-OES instrument with an RF generator power 1150 W (Thermo, USA). The auxiliary, carrier and plasma gas (Ar) flow rates were set to be 0.5, 0.5 and 14.0 L min−1, respectively. The wavelength of emission line for cobalt is 228.616 nm. For the measurement of Co2+ in alcohol solution, to avoid the influence of alcohol, the solvent of collected samples was replaced into the equivalent amount of water in advance. EDS was displayed on a JEM 2100F STEM/EDS and the X-ray energy resolution is 132 eV.
3. Results and discussion
3.1 Stability of Co2+ on chitosan hydrogel matrix
The success of in situ synthesis of Co3O4 nanoparticles on chitosan hydrogel microsphere and thereby catalytic performance require that chitosan chains should display great coordination ability with Co2+. Hence, the adsorption ability and stability of Co2+ on chitosan hydrogel matrix were firstly explored. For the sake of easy observation, the chitosan beads were employed. As shown in Fig. 1a–c, the original chitosan beads derived from alkali solvent system were white translucent. After incubating the chitosan beads in CoCl2 water or alcohol solution for 24 h, the beads showed light pink and blue colors, respectively. These results demonstrated that Co2+ could be adsorbed on chitosan hydrogel matrix in both solutions. The pink color indicates the formation of cobalt hydrates in water system. It is believed that the chemical groups on chitosan chains, such as –OH, –NH2 and –NHCOCH3 moieties commonly contributed to the strong binding interaction with Co2+. As illustrated in Fig. 1d, besides the chelating ability of –NH2,26,27 Co2+ could be immobilized to polymer chains probably via electrostatic (i.e., ion-dipole) interaction. It is expected that the reciprocal interactions between the electron-rich oxygen atoms of polar hydroxyl or ether groups of chitosan and the transition metal cations occurred.28 Meanwhile, these strong interactions could favor to stabilize the further synthesized catalytic nanoparticles by bonding with their surface metal atoms.28 Moreover, due to the strong affinity, the anchored Co2+ could not be released from the chitosan matrix even in many organic solvents upon 48 h incubation. As indicated in Fig. 1e, no obvious color changes was observed for Co2+ supported chitosan beads in the selected solvents, revealing the potential application of chitosan microspheres as the effective catalyst support. It should be noted that the NH3·H2O component is not allowed in solvent system, since it shows higher coordination ability with Co2+, and could give rise to the detachment of Co-based materials from the matrix.
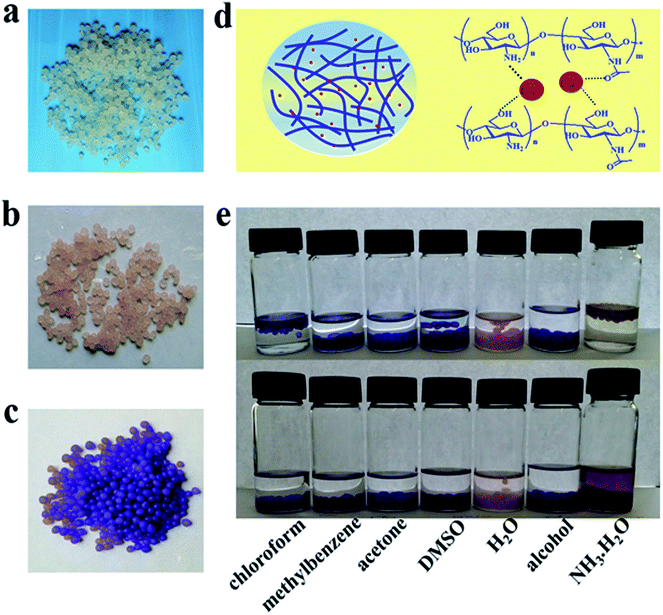 |
| Fig. 1 Photographs of the chitosan hydrogel spheres, (a) before adsorption of Co2+, after adsorption of Co2+ (b) in water and (c) in alcohol solution. (d) Schematic of the possible coordination effects for Co2+ in chitosan hydrogel matrix. (e) The stabilities of Co2+ anchored in hydrogel sphere matrix in different solvents with the incubation time for 1 h (top) and 48 h (bottom). | |
3.2 Adsorption of Co2+ on chitosan microspheres
In order to facilitate the Co2+ adsorption on support, chitosan microspheres with large specific surface area were prepared by using the traditional emulsion method. As shown in Fig. 2a and b, the obtained microspheres were spherical shape with a mean diameter of 50 μm. The SEM image of microsphere surface (Fig. 2c) displayed a homogeneous network architecture woven by nanofibers. These nanofibers not only endow the chitosan hydrogel with much higher mechanical strength,22 but also chemically crosslinked with each other to develop many nanopores with the size of 50–400 nm. These nanopores are essential for the introduction of metal ions, which further would allow the Co-based catalytic nanoparticles growing in the limited pore size. After adsorption, however, the pore size of chitosan microspheres was becoming smaller (Fig. 2d), indicating that Co2+ was filled in the pore via strong interactions. EDS and XPS analysis were also employed to verify the existence of Co2+ in chitosan microspheres. As shown in Fig. 2e and f, certain amount of Co element was detected in the samples. Meanwhile, the appearance of peaks for Co 2p3/2 at 779.6 eV and 784.35 eV and peaks for Co 2p1/2 at 795.4 eV and 800.75 eV can be observed.29 These findings clearly demonstrated that Co2+ was fully anchored on chitosan microspheres.
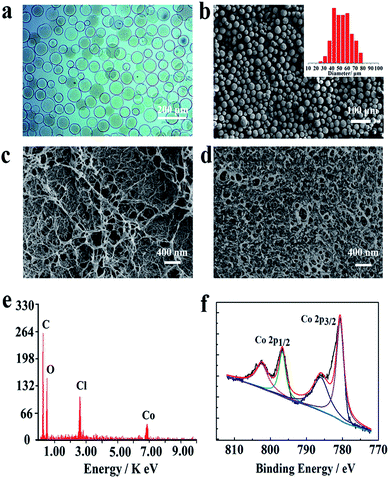 |
| Fig. 2 (a) Optical microscope and (b) SEM images of the prepared chitosan microspheres. The variation of SEM images for the chitosan microsphere surface (c) before and (d) after adsorption of Co2+. (e) Elemental analysis and (f) XPS spectrum of Co 2p for the chitosan microspheres after adsorption of Co2+. | |
3.3 Adsorption kinetics of Co2+ on chitosan microspheres
Fig. 3 presents the dependence of the qe value for Co2+ adsorption on chitosan microspheres versus the incubation time in water and alcohol solutions, respectively. Similar adsorption behaviors were observed in both solutions. The qe increased almost linearly in the initial period, and then gradually reached saturation after 24 h incubation. The difference is that chitosan microspheres display a higher qe value (about 4 times) in alcohol than in water. This is most likely because that Co2+ could exist in water in terms of various hydrated cobalt(II) ions, such as [Co(H2O)4]2+, [Co(H2O)6]2+ and [CoCl(H2O)5]+.30,31 These hydrate ions with great molecular size could eventually reduce the adsorption of Co2+ on the chitosan chains to a certain degree. In addition, all the time profiles of the Co2+ uptake in each solution was smooth and continuous until the equilibrium, implying that the monolayer adsorption possibly occurred on the surface of the chitosan microspheres.25
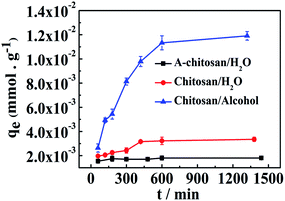 |
| Fig. 3 The effect of contact time on the Co2+ adsorption onto chitosan microspheres in water and alcohol solutions at 20 °C. A-chitosan indicated the chitosan microspheres derived from the acetic acid solutions. | |
More interestingly, the chitosan microspheres fabricated from the alkaline solvent system could display enhanced adsorption efficiency for Co2+ at determined concentration both in the water and alcohol solutions, by comparing to the conventional chitosan hydrogel microspheres from the acetic acid solution (A-chitosan). As indicated in figure, the loading capacity on chitosan microsphere is about 2–7 times as higher as that of the A-chitosan matrix, demonstrating the advantages in adsorption performance. The more extended polymer chain conformation in chitosan 3-D fibrous hydrogel network will supply more binding sites for metal ions, and be favorable to the binding activity. In order to investigate the adsorption kinetic mechanisms for cobalt ions on the chemically cross-linked chitosan microspheres, several basic models as described below were introduced. These models could be readily used to evaluate the ions or dye molecules uptake rate, which determines the residence time of adsorbent uptake at the solid–solution interface.32 The related parameters obtained from these equations will supply useful information for designing and modeling the adsorption process.
3.3.1. The pseudo-first-order and pseudo-second-order equations. The adsorption mechanism of cobalt ions in solid phase adsorption is well explained with respect to the correlation of the kinetic data. The pseudo-first-order kinetic equation can be depicted as follows:33 |
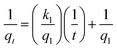 | (2) |
The pseudo-second-order kinetic model can be referred to:34
|
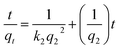 | (3) |
where
qt is the uptake amount of Co
2+ (mmol g
−1) on chitosan microspheres at different incubation time,
q1 and
q2 are the maximum adsorption capacity (mmol g
−1) for the pseudo-first-order and pseudo-second-order adsorption, respectively.
k1 is the pseudo-first-order rate constant for the adsorption process (h
−1),
k2 is the rate constant of pseudo-second-order (g mmol
−1 h
−1). The validity of these models can be interpreted by the linear plots of ln(
qe −
qt)
versus t (
Fig. 4a) and
t/
qt versus t (
Fig. 4b), respectively. Thereby, the values of
k1,
k2,
q1,
q2 and correlation coefficients
R12 and
R22 for Co
2+ adsorption in different solutions can be calculated from these fitting plots, as listed in
Table 1.
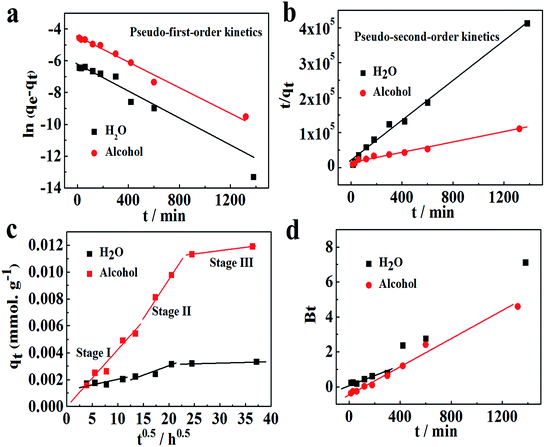 |
| Fig. 4 (a) Pseudo-first-order model and (b) pseudo-second-order model for Co2+ adsorption onto chitosan microspheres in H2O and alcohol solutions at 20 °C. (c) Intra-particle diffusion plots and (d) Boyd plots for Co2+ adsorption on chitosan microspheres in different solutions at 20 °C. | |
Table 1 Kinetic parameters for Co2+ adsorption onto chitosan microspheres in different solutions
|
Pseudo-first-order kinetics |
Pseudo-second-order kinetics |
k1 (h−1) |
qe (mmol g−1) |
R12 |
k2 (g mmol−1 h−1) |
qe (mmol g−1) |
R22 |
H2O |
0.005 |
0.0023 |
0.9796 |
4.526 |
0.0035 |
0.9922 |
Alcohol |
0.0039 |
0.0112 |
0.9834 |
0.3850 |
0.0137 |
0.9795 |
According to the calculated results from the pseudo-second-order kinetic equation, the maximum adsorption capacity for Co2+ in water aqueous solution, q2, is almost same to the observed experimental data. Meanwhile, the correlation coefficients (R22) for pseudo-second-order kinetic model is 0.9922, which is much closer to 1.0. Hence, the adsorption in water solution follows the pseudo-second-order kinetic model, implying that Co2+ adsorption on chitosan microspheres is controlled by the inner surface adsorption. In other words, Co2+ adsorption behavior on chitosan microspheres is dominated by chemical adsorption.35 However, compared to the adsorption in water, the correlation coefficients (R12 and R22) of the pseudo-first-order and pseudo-second-order models for the Co2+ adsorption in alcohol solution, are closer to each other (0.9834 and 0.9795). Conclusively, the liquid film diffusion and the inner surface adsorption are the rate-limiting steps. This also suggests that the chemical and physical adsorptions commonly contribute to the Co2+ adsorption on chitosan microspheres in alcohol solution.29,36
3.3.2. Intra-particle diffusion model. Adsorption process usually involves multiple steps,37 including (1) the migration of ions or dye molecules from bulk liquid medium to the surface of the adsorbent; (2) the subsequent diffusion of the adsorbed molecules to the exterior of the matrix through boundary layer; (3) immobilization of solute molecules at an active site on the surface of the adsorbent and (4) intra-particle diffusion into the interior of the adsorbent through the pores. Usually, the intra-particle diffusion process is considered to be the rate-controlling step in many adsorption process, where the uptake amount of solutes increases almost linearly with t0.5 rather than with the contact time t.38 Therefore, the adsorption behavior can be depicted by the intra-particle diffusion model:where kpi is the intra-particle diffusion rate constant of stage i (mmol g−1 h−0.5) and Ci, the corresponding intercept at stage i, represents the thickness of boundary layer. The larger intercept demonstrates the greater boundary layer effect.39 According to the theory, qt versus t0.5 should be linear when the intra-particle diffusion occurred in the adsorption process. Otherwise, additional mechanisms combined with the intra-particle diffusion are involved. In some cases, the plot of qt against t0.5 may display a multi-linear profile in the adsorption process. The first sharp region would be the instantaneous adsorption or external surface adsorption. The second region is a slow-adsorption stage, which is deemed to be the rate-limiting step over the whole adsorption process. In the third region, as the final equilibrium stage, the intra-particle diffusion begins to slow down, since the solute concentration in solution decreased and the body of the adsorbent saturated with the solutes.38,40Fig. 4c shows the intra-particle diffusion plot of Co2+ uptake on chitosan microspheres in water and alcohol solutions, respectively. In both cases, the linear lines of all the three stages did not pass through the origin, revealing that the overall rate of mass transfer at the initial stage of adsorption process did not solely depend on the intra-particle diffusion. It is concluded that both the film diffusion (or chemical reaction) at the first linear portion and the following pore diffusion at the second linear segments dominate the rate of whole adsorption process. Further evidence could be collected from the data fitting based on the Boyd's model,41,42 which is given as:
|
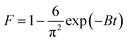 | (5) |
where
F represents the fractional attainment of equilibrium at different contact times
t, and
Bt is a function of
F |
 | (6) |
where
qt and
qe are the amount of Co
2+ adsorbed (mmol g
−1) on chitosan microspheres at time
t and at equilibrium state, respectively.
Then eqn (5) could be rewritten as:
|
Bt = −0.4977 − ln(1 − F)
| (7) |
Fig. 4d shows the Boyd plots for the Co2+ adsorption on chitosan microspheres in each solution system. The rate-controlling step in the adsorption process will be easily distinguished from the linearity of the plots. If the values of Bt change linearly against the incubation time t and pass through the origin, the rate of mass transfer is controlled by the pore diffusion. If the plot profile is nonlinear or linear without passing through the origin, the film diffusion or chemical reaction will mainly contribute to the adsorption rate. These results clearly indicated that similar mechanisms occurred to the Co2+ adsorption on chitosan microspheres in water and alcohol solutions, where the film diffusion or chemical reaction is the rate-limiting step in the initial period of adsorption process and then follows the intra-particle diffusion.
3.4 Effects of pH on Co2+ adsorption on chitosan microspheres
It has been proved that chemically crosslinked chitosan hydrogels derived from the alkaline solvent system exhibited significant pH sensitive behaviors.16 As illustrated in Fig. S2,† chitosan hydrogel showed the higher swelling ratios along with the decrease in pH value, but without any obvious degradation or loss in weight. The protonation of amine groups below pH 6.5 plays an important role in the whole swelling process.43 Thus, the adsorption behaviors for Co2+ on chitosan microspheres, particularly for the adsorption capacity, is also believed to be strongly dependent on the pH values of solution. As shown in Fig. 5, it presents different behaviors for Co2+ adsorption on chitosan microspheres during the pH range of 1.0 to 10.0. The adsorption capacity increased sharply as the pH value increased from 3.0 to 7.0. When the pH of the solution is at a low value, the amino groups of chitosan are protonated and H+ could compete with cobalt ions for the adsorption sites of –NH2, resulting in a lower number of binding sites for cobalt ions. Meanwhile, the protonation of –NH2 would result in the electrostatic repulsive force with Co2+ and prevent the metal complex formation. However, with an increase in pH value, the protonated amino groups are deprotonated gradually, allowing for more and stronger chelating interactions between cobalt ions and chitosan chains. Similar mechanism was also involved in the polyethylenimine-cross-linked cellulose nanocrystals as an efficient adsorbents for rare earth elements.44 The protonation of amine groups (NH3+ and –NH2+–) would result in a strong electrostatic repulsion of metal ions at low pH value, whereas the lone pair of electrons could exist in the neutral nitrogen atoms of –NH2 and –NH– groups under high pH condition, eventually leading to the formation of coordination bond between N atoms of the amino groups and the rare earth element atoms.
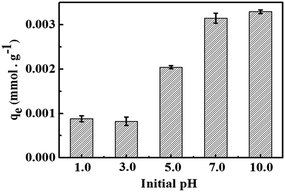 |
| Fig. 5 Effects of pH on the uptake capacity of Co2+ on chitosan microspheres in water solution at 20 °C. | |
Neither the low nor much higher pH values are beneficial to the Co2+ adsorption on chitosan matrix. When the pH values is higher than 7.0, Co2+ and the excessive OH− ligands could form the stable hydroxo-cobalt(II) complexes.31 The loading ability might be suppressed in a certain degree. Therefore, the premium pH environment for Co2+ adsorption performance should be limited to the neutral solution.
3.5 Adsorption isotherms of Co2+ on chitosan microspheres
The equilibrium adsorption isotherm is fundamental in describing the relationship between the uptake amount of metal ions at the determined temperature per unit mass of the adsorbent and the liquid phase ions concentration at equilibrium. Generally, the adsorption capacity of metal ions in aqueous solution increases as their concentration increases.25 As presented in Fig. 6a, the amount of Co2+ adsorbed onto the chitosan microspheres in water solution, qe, increased from 9.74 × 10−4 to 1.36 × 10−2 mmol g−1, when the initial concentration of Co2+ increased from 0.5 to 10.0 mmol L−1 at 293 K. Same tendency was observed for the Co2+ adsorption in alcohol solution (Fig. 6d), where the loading capacity onto the chitosan microspheres increased from 5.82 × 10−3 to 5.43 × 10−2 mmol g−1. To further distinguish the differences of adsorption in each solution and quantify the adsorption capacity of Co2+ on chitosan microspheres, adsorption isotherms including the Langmuir and Freundlich equations were employed in this work. It is of great importance to learn more about the mutual interactions between each other and optimize the use of adsorbents. The Langmuir isotherm model is established on the basis of the following assumptions: monolayer adsorption occurs between the solute molecules and the adsorbent; the number of active sites on the adsorbent surface is limited and the adsorption of molecules on the surface of the adsorbent is uniform. It is represented in the following form:25 |
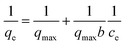 | (8) |
where qmax is the maximum adsorption capacity regarding to the monolayer coverage on the surface (mmol g−1) and b is the Langmuir constant (L mmol−1), reflecting the uptake efficiency. Unlike Langmuir model, the Freundlich isotherm is an empirical equation, which is mainly used to study the multilayer adsorption process on heterogeneous surface. It can be expressed as follows:45 |
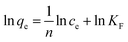 | (9) |
where KF is the Freundlich isotherm constant, and the exponent 1/n is the heterogeneity factor, demonstrating the adsorption capacity and adsorption intensity, respectively.
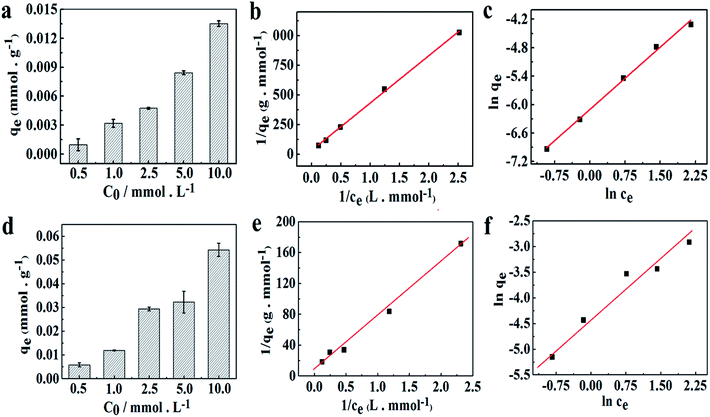 |
| Fig. 6 Adsorption isotherms for Co2+ adsorption on chitosan microspheres in (a) water and (d) alcohol solutions at 20 °C. Langmuir isotherms for Co2+ adsorption on chitosan microspheres in (b) water and (e) alcohol solutions at 20 °C. Freundlich isotherms for Co2+ adsorption on chitosan microspheres in (c) water and (f) alcohol solutions at 20 °C. | |
Fig. 6b exhibits the Langmuir isotherm for Co2+ adsorption on chitosan microspheres in water solution at 293 K, and the corresponding parameters are summarized in Table 2. The value of qmax was approximately evaluated to be 0.032 mmol g−1, suggesting that the chitosan microspheres could display high adsorption capacity for Co2+ in water solution. Fig. 6c shows the Freundlich profile for Co2+ adsorption in the same process. In this empirical equation, the Freundlich constant (1/n) is closely related to the adsorption intensity of the adsorbent. When 0.1 < 1/n ≤ 0.5, the adsorbent could perform excellent uptake behavior; 0.5 < 1/n ≤ 1, adsorbates are easy to be anchored on the matrix; 1/n > 1, adsorption behavior hardly occurs.46 As indicated in Table 2, the value of 1/n was determined to be 0.876 at 293 K, revealing that Co2+ could be easily anchored on the chitosan microspheres. In comparison, the adsorption behavior of Co2+ on chitosan microspheres could be better described by the Langmuir equation, since the regression coefficient of R2 obtained from the Langmuir model (R2 = 0.9985) is higher than that obtained from the Freundlich model (R2 = 0.9751). Namely, the adsorption process in water solution is primarily dominated by monolayer adsorption.
Table 2 Langmuir and Freundlich parameters for Co2+ adsorption onto chitosan microspheres in water and alcohol solutions
|
Langmuir parameters |
Freundlich parameters |
qmax (mmol g−1) |
b (L mmol−1) |
R2 |
1/n |
KF (mmol g−1) |
R2 |
H2O |
0.032 |
0.079 |
0.9985 |
0.876 |
0.0022 |
0.9751 |
Alcohol |
0.128 |
0.112 |
0.9891 |
0.735 |
0.0126 |
0.9446 |
As for the adsorption activity in alcohol system, the maximum loading efficiency for Co2+ on chitosan microspheres was greatly enhanced by 3 times (shown in Fig. 6e and Table 2). Meanwhile, the Freundlich constant was estimated to be 0.735, which is smaller than that collected from the water solution (Fig. 6f and Table 2). These results fully demonstrated the occurrence of higher loading efficiency for Co2+ on chitosan microspheres and stronger affinity of Co2+ to chitosan chains in alcohol solution. Moreover, it was found that the adsorption of Co2+ preferably followed the Langmuir adsorption model with a correlation coefficient R2 value of 0.9891, which is significantly better than the Freundlich model (R2 = 0.9446). Hence, it can be concluded that the chemical adsorption was readily involved in the process of Co2+ adsorption in alcohol solution.
3.6 Thermodynamic parameters of Co2+Adsorption
Fig. 7a and c depict the effects of incubation temperature on the uptake capacity of Co2+ on chitosan microspheres in each solution. The equilibrium adsorption capacity in water and alcohol solutions increased from 2.41 × 10−3 to 3.57 × 10−3 mmol g−1 and from 1.10 × 10−2 to 1.38 × 10−2 mmol g−1, respectively, as the temperature was elevated from 4 to 37 °C, confirming the endothermic nature of the on-going process in both cases. Hence, it is essential to clarify the feasibility and endothermic nature of the adsorption process in terms of the changes in thermodynamic parameters, such as standard free energy (ΔG°), enthalpy change (ΔH°), and entropy change (ΔS°). Gibbs energy change, ΔG°are determined based on the following equations:47 |
ΔG° = −RT ln Kd
| (10) |
|
 | (11) |
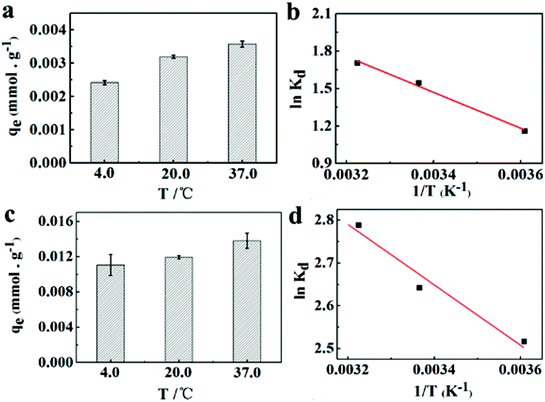 |
| Fig. 7 Effects of temperature on uptake capacity of Co2+ on chitosan microspheres in (a) water and (c) alcohol solution. (b and d) The corresponding Van't Hoff plots for Co2+ adsorption on chitosan microspheres in each solution. | |
The values of ΔH°and ΔS° can be calculated by van't Hoff equation:
|
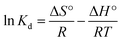 | (12) |
where
Kd is indicative of the standard thermodynamic equilibrium constant at the determined temperature,
R is the gas constant (8.314 J mol
−1 K
−1),
T is the absolute temperature (K).
The Gibbs free energy can predict whether an adsorption behavior will undergo a favorable and spontaneous process. As shown in Table 3, the values of ΔG°for Co2+ adsorption on chitosan microspheres in all cases were negative, demonstrating that the adsorption of Co2+ on chitosan microspheres could occur spontaneously. The decrease in the negative value of ΔG° with an increase in temperature indicates that the higher temperature is beneficial to the Co2+ adsorption in both solutions. Furthermore, combined the eqn (11) with eqn (12), the linear plotting of ln
Kd versus 1/T could give more information (Fig. 7b and d). According to the fitting profiles, the values of ΔH° and ΔS° in water solution were determined to be 11.94 kJ mol−1 and 52.81 J mol−1 K−1, and the values in alcohol solution were estimated to be 5.71 kJ mol−1 and 41.43 J mol−1 K−1, respectively. The positive values of ΔH°suggest the endothermic nature of the adsorption process and the existence of an energy barrier. The positive values of ΔS°imply the good binding ability of Co2+ to the chitosan microspheres and the increased randomness at the solid–solution interface.48 All the thermodynamic parameters confirmed that chitosan microspheres derived from alkaline solution could display a high-efficiency adsorption to Co2+ in aqueous solutions.
Table 3 Thermodynamic parameters for Co2+ adsorption onto chitosan microspheres in water and alcohol solutions
Temperature (K) |
ΔHo (kJ mol−1) |
ΔSo (J mol−1 K−1) |
ΔGo (kJ mol−1) |
H2O |
Alcohol |
H2O |
Alcohol |
H2O |
Alcohol |
277 |
11.94 |
5.71 |
52.81 |
41.43 |
−2.67 |
−5.80 |
293 |
|
|
|
|
−3.78 |
−6.44 |
310 |
|
|
|
|
−4.39 |
−7.19 |
3.7 Regeneration
As an advanced adsorbent for feasible and practical application, how to recover and maintain the original adsorption capacity is of great significance. In this study, Co2+-loaded chitosan microspheres were regenerated by using 0.1 mol L−1 EDTA aqueous solution,49 and the adsorption–desorption cycle was repeated three times. As shown in Table 4 and Fig. S3,† the desorption ratios of Co2+ from the matrix were evaluated to be 81.51, 86.84, and 83.49% in each cycle. These results reveal that most of the Co2+ adsorbed on chitosan microspheres could be removed in the process of static elution incubation, testifying the potential re-usability of the adsorbent. When the regenerated chitosan microspheres were deposited in Co2+ water solution upon the same incubation condition, they showed a slight decrease tendency in the loading efficiency after each cycle. The existence of the small and reasonable decrease in adsorption capacity in subsequent cycles may be due to the static elution method, resulting in the incomplete desorption of ions from the matrix. In spite of this, chitosan microspheres derived from the alkaline solution still could be good candidates for future application.
Table 4 Repeated adsorption capacity and desorption efficiency of Co2+ on chitosan microspheres in the desorption–regeneration cycles
Cycle number |
Adsorption capacity (mmol g−1) |
Desorption efficiency (%) |
1 |
0.0135 |
81.51 |
2 |
0.0106 |
86.84 |
3 |
0.0087 |
83.49 |
4. Conclusion
Chemically crosslinked robust chitosan microspheres were successfully prepared from the LiOH/KOH/urea aqueous system via the W/O emulsion method. The obtained microspheres with 3-D fibrous network structures could display greatly enhanced adsorption capacity to Co2+ in both water and alcohol solutions, by comparing to the conventional chitosan hydrogel microspheres from the acetic acid solution. Meanwhile, the Co2+ ions could be highly stabilized on the chitosan matrix in many organic solvents. The good correlation coefficient (0.9922) suggests that the adsorption in water solution obeys the pseudo-second-order kinetic model, implying that Co2+ adsorption on chitosan microspheres is mainly controlled by the inner surface adsorption. While in the alcohol solution, both the chemical and physical adsorptions commonly take the responsibility for the higher Co2+ adsorption capacity on chitosan microspheres. In addition, the adsorption behaviors strongly depend on the initial concentration of the Co2+, pH value and the incubation temperature. The adsorption process of Co2+ on chitosan microspheres in both cases can be suitably described by the Langmuir isotherm, and the thermodynamic analysis indicates that the adsorption behavior was spontaneous and endothermic. This work provides the full theoretical basis for loading Co2+, and reveals that chitosan microspheres would be readily taken as a candidate support for cobalt-based catalysts.
Conflicts of interest
There are no conflicts to declare.
Acknowledgements
We gratefully acknowledge the financial supports from Natural Science Foundation of China (No. 51603195 and 21504025), Natural Science Foundation (No. 110246) and New Faculty Startup Funds from North University of China. We also greatly appreciate Prof. Wei Li's assistance in the grammar and spelling throughout the manuscript and Dr Melanie Barnes's help in ICP testing.
References
- D. Wang, Q. Wang and T. Wang, Inorg. Chem., 2011, 50, 6482–6492 CrossRef CAS PubMed.
- X. Liu, Q. Long, C. Jiang, B. Zhan, C. Li, S. Liu, Q. Zhao, W. Huang and X. Dong, Nanoscale, 2013, 5, 6525–6529 RSC.
- S. H. Kazemi, B. Karimi, S. A. Aghdam, H. Behzadnia and M. A. Kiani, RSC Adv., 2015, 5, 69032–69041 RSC.
- H. B. Wu, H. Pang and X. W. D. Lou, Energy Environ. Sci., 2013, 6, 3619–3626 RSC.
- F. Wyrwalski, J.-M. Giraudon and J.-F. Lamonier, Catal. Lett., 2010, 137, 141–149 CrossRef CAS.
- Z.-S. Wu, W. Ren, L. Wen, L. Gao, J. Zhao, Z. Chen, G. Zhou, F. Li and H.-M. Cheng, ACS Nano, 2010, 4, 3187–3194 CrossRef CAS PubMed.
- X. Xie and W. Shen, Nanoscale, 2009, 1, 50–60 RSC.
- T. E. Davies, T. García, B. Solsona and S. H. Taylor, Chem. Commun., 2006, 3417–3419 RSC.
- X. Deng and H. Tüysüz, ACS Catal., 2014, 4, 3701–3714 CrossRef CAS.
- G. H. Wang, X. Deng, D. Gu, K. Chen, H. Tüysüz, B. Spliethoff, H. J. Bongard, C. Weidenthaler, W. Schmidt and F. Schüth, Angew. Chem., 2016, 128, 11267–11271 CrossRef.
- X. Deng, R. Rin, J. C. Tseng, C. Weidenthaler, U. P. Apfel and H. Tüysüz, ChemCatChem, 2017, 9, 4238–4243 CrossRef CAS.
- H. M. Choi, S.-J. Lee, S.-H. Moon, T. N. Phan, S. G. Jeon and C. H. Ko, Catal. Commun., 2016, 82, 50–54 CrossRef CAS.
- W. W. Ngah, L. Teong and M. Hanafiah, Carbohydr. Polym., 2011, 83, 1446–1456 CrossRef.
- C. Gerente, V. Lee, P. L. Cloirec and G. McKay, Crit. Rev. Environ. Sci. Technol., 2007, 37, 41–127 CrossRef CAS.
- J. Roosen, J. Spooren and K. Binnemans, J. Mater. Chem. A, 2014, 2, 19415–19426 RSC.
- L. Zeng, Y. Chen, Q. Zhang, X. Guo, Y. Peng, H. Xiao, X. Chen and J. Luo, Carbohydr. Polym., 2015, 130, 333–343 CrossRef CAS PubMed.
- F. Zhao, E. Repo, M. Sillanpää, Y. Meng, D. Yin and W. Z. Tang, Ind. Eng. Chem. Res., 2015, 54, 1271–1281 CrossRef CAS.
- F. Zhao, E. Repo, D. Yin and M. E. Sillanpää, J. Colloid Interface Sci., 2013, 409, 174–182 CrossRef CAS PubMed.
- F. Zhao, E. Repo, D. Yin, L. Chen, S. Kalliola, J. Tang, E. Iakovleva, K. C. Tam and M. Sillanpää, Sci. Rep., 2017, 7, 15811 CrossRef PubMed.
- F. Zhao, E. Repo, D. Yin, Y. Meng, S. Jafari and M. Sillanpää, Environ. Sci. Technol., 2015, 49, 10570–10580 CrossRef CAS PubMed.
- E. Guibal, Prog. Polym. Sci., 2005, 30, 71–109 CrossRef CAS.
- J. Duan, X. Liang, Y. Cao, S. Wang and L. Zhang, Macromolecules, 2015, 48, 2706–2714 CrossRef CAS.
- J. Duan, X. Liang, J. Guo, K. Zhu and L. Zhang, Adv. Mater., 2016, 28, 8037–8044 CrossRef CAS PubMed.
- M. Kong, X. G. Chen, C. S. Liu, C. G. Liu, X. H. Meng and L. J. Yu, Colloids Surf., B, 2008, 65, 197–202 CrossRef CAS PubMed.
- H. Tang, W. Zhou and L. Zhang, J. Hazard. Mater., 2012, 209, 218–225 CrossRef PubMed.
- Z. Sun, F. Lv, L. Cao, L. Liu, Y. Zhang and Z. Lu, Angew. Chem., Int. Ed., 2015, 54, 7944–7948 CrossRef CAS PubMed.
- A. Varma, S. Deshpande and J. Kennedy, Carbohydr. Polym., 2004, 55, 77–93 CrossRef CAS.
- J. He, T. Kunitake and A. Nakao, Chem. Mater., 2003, 15, 4401–4406 CrossRef CAS.
- W. Luo, Z. Bai and Y. Zhu, New J. Chem., 2017, 41, 3487–3497 RSC.
- T. Swift, Inorg. Chem., 1964, 3, 526–529 CrossRef CAS.
- K. Mech, P. Żabiński and R. Kowalik, J. Electrochem. Soc., 2013, 160, D246–D250 CrossRef CAS.
- M. Sulak, E. Demirbas and M. Kobya, Bioresour. Technol., 2007, 98, 2590–2598 CrossRef CAS PubMed.
- H. Tu, M. Huang, Y. Yi, Z. Li, Y. Zhan, J. Chen, Y. Wu, X. Shi, H. Deng and Y. Du, Appl. Surf. Sci., 2017, 426, 545–553 CrossRef CAS.
- Y.-S. Ho and G. McKay, Process Biochem., 1999, 34, 451–465 CrossRef CAS.
- A. S. Özcan and A. Özcan, J. Colloid Interface Sci., 2004, 276, 39–46 CrossRef PubMed.
- S. Azizian and H. Bashiri, Langmuir, 2008, 24, 11669–11676 CrossRef CAS PubMed.
- M. Doğan, M. Alkan, A. Türkyilmaz and Y. Özdemir, J. Hazard. Mater., 2004, 109, 141–148 CrossRef PubMed.
- B. Hameed, I. Tan and A. Ahmad, Chem. Eng. J., 2008, 144, 235–244 CrossRef CAS.
- D. Kavitha and C. Namasivayam, Bioresour. Technol., 2007, 98, 14–21 CrossRef CAS PubMed.
- Q. Sun and L. Yang, Water Res., 2003, 37, 1535–1544 CrossRef CAS PubMed.
- A. Özcan, E. M. Öncü and A. S. Özcan, J. Hazard. Mater., 2006, 129, 244–252 CrossRef PubMed.
- G. Boyd, A. Adamson and L. Myers Jr, J. Am. Chem. Soc., 1947, 69, 2836–2848 CrossRef CAS PubMed.
- G. Crini and P.-M. Badot, Prog. Polym. Sci., 2008, 33, 399–447 CrossRef CAS.
- F. Zhao, E. Repo, Y. Song, D. Yin, S. B. Hammouda, L. Chen, S. Kalliola, J. Tang, K. C. Tam and M. Sillanpää, Green Chem., 2017, 19, 4816–4828 RSC.
- G. Bayramoglu, A. Denizli, S. Bektas and M. Y. Arica, Microchem. J., 2002, 72, 63–76 CrossRef CAS.
- B. Samiey and M. Dargahi, Open Chem., 2010, 8, 906–912 CAS.
- D. Ding, Y. Zhao, S. Yang, W. Shi, Z. Zhang, Z. Lei and Y. Yang, Water Res., 2013, 47, 2563–2571 CrossRef CAS PubMed.
- X.-F. Sun, S.-G. Wang, X.-W. Liu, W.-X. Gong, N. Bao, B.-Y. Gao and H.-Y. Zhang, Bioresour. Technol., 2008, 99, 3475–3483 CrossRef CAS PubMed.
- L. Zhou, Y. Wang, Z. Liu and Q. Huang, J. Hazard. Mater., 2009, 161, 995–1002 CrossRef CAS PubMed.
Footnote |
† Electronic supplementary information (ESI) available. See DOI: 10.1039/c8ra06110f |
|
This journal is © The Royal Society of Chemistry 2018 |
Click here to see how this site uses Cookies. View our privacy policy here.