DOI:
10.1039/C8RA04853C
(Paper)
RSC Adv., 2018,
8, 26399-26406
Biomethanation of blast furnace gas using anaerobic granular sludge via addition of hydrogen†
Received
6th June 2018
, Accepted 17th July 2018
First published on 24th July 2018
Abstract
The high concentrations of CO (toxic) and CO2 (greenhouse gases) in blast furnace gas (a by-product of steelworks) reflect its low calorific value. In this study, anaerobic granular sludge was used to convert carbon from blast furnace gas to methane via exogenous hydrogen addition. The inhibition of methane production by CO partial pressure (PCO) was found to start from 0.4 atm. The intermediate metabolites from CO to methane including acetate, propionate, and H2 accumulated at higher CO concentrations in the presence of 2-bromoethanesulfonic acid. After the introduction of H2 and blast furnace gas, although the hydrogen partial pressure (PH2) up to 1.54 atm resulted in the maximum CH4 yield, the whole system was not stable due to the accumulation of a large amount of volatile fatty acids. The optimum PH2 on CH4 production from the simulated blast furnace gas, 5.32 mmol g−1 VSS, was determined at 0.88 atm in this study.
Introduction
In 2014, Japan's total carbon dioxide (CO2) discharge was approximately 1.2 billion tons, accounting for 92.8% of the total greenhouse gas (GHG) emissions. The steel industry (14%) is the second largest source of CO2 emission after the power industry (39%) according to a recent survey.1 In other words, the steel industry should take an important role in reducing GHG emissions.2 Blast furnace gas (BFG) is a byproduct gas produced during the production of hot metal (liquid iron) in a blast furnace from the iron and steel industry. The composition of BFG is as follows: 20–35% CO2, 20–30% CO, 2–4% H2 and 50–60% N2.3 Although BFG can be directly used as fuel for steam boilers, dynamos, and as the supplement of traditional fossil fuels in thermal units,4 the calorific value is low due to the fact that carbon monoxide (CO) has a low energy density and CO2 is non-flammable.5 It is well known that the high affinity to metal-containing enzyme makes CO be toxic to many microorganisms.6 Therefore, the high concentration of CO in BFG makes it to be hazardous.
For the treatment of BFG, absorbents can be developed for CO2 capture from BFG to recover the carbon source for integrated steelworks.2 Besides, hydrogen can be produced from CO in BFG through water–gas shift reaction (as shown in reaction 3 below) regardless of CO2 is captured or separated.7 However, strict reaction conditions need to be controlled such as the specific catalyst development and high temperature. The mixtures of CO, CO2, and H2 can also be used as sources for biofuels production by microorganisms, like ethanol,8 volatile fatty acids9 and 2,3-butanediol.10
Compared with H2, CH4 is also a good source of clean energy, and its storage cost is only about 1/3 of H2.11 Higher quality of fuel will be obtained through biomethanation of CO2 and CO from BFG, which could be further utilized as heating fuel or power generation for steel industry. Therefore, this form of carbon recycling not only saves costs but also helps reduce GHG. In addition, there are ready-made pipe networks for CH4 transportation and distribution. As per biological methods, a moderate temperature and pressure, and a low energy consumption are their merits. Besides, the high specificity of enzymes involved may bring about higher product yields and less by-products. Biological conversion of CO2 (ref. 8 and 9) and/or CO12 to CH4 by anaerobic microorganisms have been studied and confirmed previously, such as Bugante, E. C. et al.13 used a column bioreactor to convert BFG to methane under thermophilic conditions. Two steps are generally involved in this process: the conversion from CO to CO2 and methane, and then extra H2 is added to the reactor for accomplishing the conversion of CO2 to methane. The related reaction processes for carboxydotrophic microorganisms and hydrogenotrophic methanogens are shown as follows.14
|
4CO + 2H2O → CH4 + 3CO2, ΔG0 = −53 kJ mol−1
| (1) |
|
CO + 3H2 → CH4 + H2O, ΔG0 = −151 kJ mol−1
| (2) |
|
CO + H2O → H2 + CO2, ΔG0 = −20 kJ mol−1
| (3) |
|
4CO + 2H2O → CH3COOH + 2 CO2, ΔG0 = −176 kJ mol−1
| (4) |
|
2CO + 2H2 → CH3COOH, ΔG0 = −67 kJ mol−1
| (5) |
|
CH3COOH → CH4 + CO2, ΔG0 = −31 kJ mol−1
| (6) |
|
4H2 + CO2 → CH4 + 2H2O, ΔG0 = −130.7 kJ mol−1
| (7) |
The above reactions about methane production include direct reaction (reactions (1), (2), and (7)) and indirect reaction (reactions (3)–(6)). For the indirect methane production, it mainly has two steps: precursors (H2 or acetate) formation from CO, H2 or CO2 by bacteria, and biomethanation of the precursors by methanogens. It can be seen that BFG could not be completely converted to methane without exogenous H2 addition, due to the co-existence of high concentration of CO2 in the BFG. Up to now, little information could be found on methane production from the mixture of BFG and H2 gases under mesophilic conditions. Here, exogenous H2 can be obtained from hydrogen containing industrial exhaust gases, such as coke oven gas (COG),15 the byproduct from the coke making process, contains around 54–59% of H2. Most of the COG is directly discharged into the air, resulting in seriously environmental pollutions. Therefore, the conversion of H2 from COG by microorganisms to methane would be more sustainable. For the hydrogen from electrolysis of fluoride-contaminated wastewater,16 in order to obtain only hydrogen, electrocoagulation technology can be applied to treat hydrofluoric acid wastewater by using renewable electricity without oxygen production. Exogenous H2 can also be obtained from in situ anaerobic corrosion of metallic iron,17 and the hydrogen produced by iron corrosion could serve as electron-donor for hydrogen-consuming microorganisms.
In the biological methods for treating CO or CO2, pure culture is sensitive to the changes of environment or strict sterilization conditions.14–16 However, it is well known that mixed culture presents rich functions, such as non-sterile conditions, high ability to adapt to different components of syngas,18 existence of rich variety of microorganisms and low cost than pure culture.19 These advantages make it more suitable for application in industry. Anaerobic granular sludge (AGS) with excellent settling property, capability of high biomass retention and ability to treat high-strength organic wastewater is a promising technology that has attracted more and more attention.20 It is usually used in upflow anaerobic sludge blanket (UASB), expanded granular sludge bed (EGSB) and internal circulation (IC) reactors to treat wastewaters at high organic loading rates. The structure of AGS is favorable to resist CO inhibition as the outer layer is dominated by heterogeneous population and bacteria, and the inner layer consists of large numbers of archaea like methanogens.21 AGS has been used in the mixed culture to convert CO to hydrogen22 or methane.23 However, until now, there is no documentation on the biomethanation of CO and CO2 from BFG by AGS via addition of exogenous H2 in mixed cultures at mesophilic conditions. Since the anaerobic digestion of wastewater by AGS in above-mentioned reactors involving multiple steps requires the participation of various microorganisms, it is possible for the microorganisms in the anaerobic reactor to convert CO and CO2 in the BFG to methane, while addition of exogenous hydrogen is assumed to promote the biomethanation of BFG more thoroughly.
Based on the above considerations, the present study aimed to investigate the feasibility of biomethanation of BFG by exogenous H2 addition. The potential of AGS for converting CO and CO2 to CH4 was examined. In addition, it is important to understand the possible effect of CO on the activity of the microorganisms and the CO methanation routes. Since H2 is a possible inhibitor to the anaerobic process, it is challenging to add both BFG and hydrogen in the reactors at the same time. In this study, the mechanisms for enhancing CH4 production by AGS via exogenous H2 addition were explored using batch tests.
Materials and methods
Sludge source
The sludge used in this study was obtained from a mesophilic Expanded Granular Sludge Blanket (EGSB) reactor treating brewery wastewater (Asahi, Ibaraki, Japan) and was stored at 4 °C. The major physicochemical characteristics of the AGS were as follows: total suspended solids (TSS) 11.7 (±0.5) g L−1, volatile suspended solids (VSS) 9.9 (±0.3) g L−1, and pH 6.8 (±0.1) with extracellular proteins of 128.1 (±2.9) mg g−1 VSS, extracellular polysaccharide of 9.4 (±0.3) mg g−1 VSS, and total organic carbon (TOC) of 679.9 (±0.8) mg g−1 VSS, respectively.
Experimental set-ups
Methanogenic potential of AGS
As CO and CO2 in BFG were designed to be converted to methane, batch experiment 1 was firstly conducted to investigate the methanogenic potential of the AGS sampled from the EGSB reactor. In this part, acetoclastic, carboxydotrophic and hydrogenotrophic methanogenic activities of the AGS in anaerobic cultures were tested in cylindrical pressure bottles (4.4 cm in diameter, 7 cm in height) with a volume of 110 ml. 50 ml of the basic medium24 and granular sludge (washed with phosphate buffer) were loaded into the bottles to reach a final volatile solids (VSS) concentration of 2 g L−1. The pH of the mixture was then adjusted to 7.2 with 2 M NaOH. In order to create anaerobic conditions, the bottles were flushed with pure N2 gas for 3 min after being capped and sealed with butyl rubber stoppers. A certain volume of N2 was removed from the bottle and replaced by an equivalent volume of CO (CO2, or H2) using a gas tight syringe to obtain the required partial pressure in the headspace. Different substrates were filled25 in the headspace with a volume of 60 ml or in the liquid, including R1-sodium acetate (30 mM) for acetoclastic methanogenic activity, R2-H2/CO2 (80/20, 2.5 atm) for hydrogenotrophic methanogenic activitiy, R3-CO/N2 (20/80,1 atm) for carboxydotrophic-a methanogenic activity, R4-CO/H2/N2 (20/64/16, 1 atm), or R5-CO/CO2/H2 (20/16/64, 1 atm) for carboxydotrophic-b methanogenic activity. The detailed operation conditions are shown in Table S1.† Among the bottles, R4 was designed to investigate the effect of H2 on CO fermentation under mesophilic conditions. The bottles with granular sludge and medium only (without gas substance) were used as the control (R0). These bottles were incubated in a thermostatic water bath oscillator at 37 ± 2 °C and 100 rpm. All the tests were performed in triplicate.
Effect of CO partial pressure on methane production by AGS
To investigate the effect of CO partial pressure (PCO) on methane production from CO and the possible pathway involved, the experiments were divided into two parts, with the scales and procedures being similar as the above except for that the VSS of AGS was 4 g L−1. The first part (batch experiment 2) was conducted to observe the effect of CO partial pressure (PCO) (0, 0.1, 0.2, 0.4, 0.8 atm) on methane production. In this experiment the headspaces of the bottles were purged with the mixture of CO and N2 at different ratios to obtain the required initial partial pressures of CO. The second batch experiment (batch experiment 3) was performed for 7 days to explore the CO conversion route under different PCO (0, 0.2, 0.4, 0.6, 0.8, 1 atm) with the methanogens inhibitor, 25 mM 2-bromoethanesulfonic acid (BES)26 being added. The impact of BES on the pathways of CO conversion to CH4 was summarized Fig. S1.†14
Effect of exogenous H2 partial pressure on methane production from blast furnace gas
In this experiment, the scales and procedures were similar with the batch experiment 1, which investigated the effect of exogenous H2 partial pressure on methane production from BFG by using AGS. The compositions of simulated blast furnace gas (TOMOE SHOKAI Co., LTD, Japan) consist of CO, CO2, H2, and N2 at a volume ratio of CO/CO2/H2/N2 = 22/22/4/52. Besides BFG (1 atm, PCO was 0.22 atm) in the headspace of the bottles, exogenous H2 was also added into each bottle up to a final hydrogen partial pressure (PH2) of 0.04 atm, 0.88 atm, and 1.54 atm, respectively (with a total pressure being adjusted to 2.6 atm with N2). The detailed operation conditions are shown in Table S2.† In this study, soluble total organic carbon (STOC) was measured and used in the carbon balance analysis.
Analysis and chemicals
Total suspended solids (TSS) and volatile suspended solids (VSS) were determined according to the Standard Methods.26 Two gas chromatographs (Shimadzu GC-8A, Japan) equipped with TCD were used to detect the concentrations of gaseous components. For H2, CH4, and CO2, the temperatures for the detector and injector were both 60 °C, and the column temperature was 80 °C with nitrogen being as the carrier gas. For CO analysis, the detector and injector temperatures were both 170 °C, and the column temperature was 80 °C with helium as the carrier gas. Volatile fatty acids (VFAs) concentrations were analyzed by a Shimadzu GC-14B/FID, and the column and the injector temperatures were set at 150 °C and 190 °C, respectively with nitrogen being the carrier gas. In this study, the concentrations of VFAs were expressed as the equivalent carbon values calculated from the theoretical formula of each VFA component. And the carbon content of VSS was calculated using C5H7O2N.27
Results and discussion
Methanogenesis potential of AGS under the tested conditions
During the anaerobic biomethanation process of gases with different compositions, it was observed that AGS obviously possessed hydrogenotrophic and acetoclastic methanogenic potentials (18.31 ± 1.2 mmol per g VSS per d and 6.58 ± 0.38 mmol per g VSS per d, respectively, Fig. 1), which are similar with previous researches.22,24 In order to investigate the carboxydotrophic potential of the AGS used in this study, R3 and R5 were prepared and tested. As seen, AGS also exhibited a promising carboxydotrophic potential (1.19 ± 0.03 mmol per g VSS per d and 5.56 ± 0.26 mmol per g VSS per d) even though the microorganisms from AGS might not adapt to CO as energy source in comparison to H2. For all runs, no lag phase was detected during CH4 production (Fig. S2(a)†). It was worth mentioning that during the conversion of CO/N2, CO was the only substrate, while a very small amount of H2 and VFAs (data not shown) were detected, possibly due to that they were the intermediates for CH4 production.
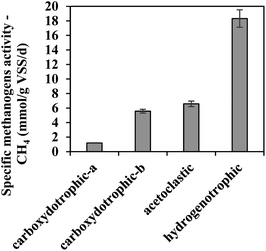 |
| Fig. 1 Anaerobic biomethanation potential of AGS used in this study at 37 °C. The substrates for carboxydotrophic-a, carboxydotrophic-b, acetoclastic, and hydrogenotrophic activities were CO/N2, CO/CO2/H2, sodium acetate, and H2/CO2, respectively. | |
The CO metabolism by microorganisms from AGS was somehow influenced by the presence of CO2 and/or H2,18,28 although it is thermodynamically feasible (reaction (2)) for methane production from CO and H2. In the process of CO fermentation under mesophilic conditions, the effect of H2 has been rarely reported.28 Compared with R3, the presence of hydrogen (R4) led to a slower trend of CO consumption which presented negatively influence (Fig. S2(b)†). Probably, CO was in part consumed during the production of the intermediates (e.g., H2 and acetate) for methanogenesis (reactions (3) and (4)). If H2 is an intermediate product, the presence of extra H2 in R4 is not conducive to the smooth progress of reaction (3). Assuming acetate is the intermediate, it may be formed from extra H2 and CO through reaction (5), and the consumption of CO in reaction (5) is slower than that of reaction (3) since 2 moles and 4 moles of CO are required to participate in the formation of 1 mole of acetate, respectively. In addition, by measuring VFAs concentrations, it accumulated at 109.5 h in R4 but not in R3 and R5 (data not shown). A similar phenomenon was noticed by Heiskanen et al. who applied Butyribacterium methylotrophicum to convert different gas substrates to biofuels (mainly acetate),29 and found that butyric acid production was increased after the supplementation of hydrogen into CO. However, the CO consumption rate in R5 was not affected by the supplementation of CO2 and H2, probably attributable to that CO2 reacted with H2 first (reaction (7)), eliminating the negative impact of CO consumption to some extent. Compared with R3, R4 seemed to have increased methane production that might be caused by the addition of hydrogen, stimulating the production of CO2 from the organics contained in the AGS,30 and then the formation of CH4. According to a previous study,28 the addition of hydrogen would not promote the direct methanogenic CO conversion in reaction (2). The microbial population presented in AGS could rapidly convert CO2 into methane within 15.5 h in R5; however, the extra CO2 production detected from 37.5 h presented a similar increasing trend as that in R3 (Fig. S2(c)†). This extra CO2 in R5 might be from CO and AGS itself. In R3, if calculated according to the reaction (1) which assumes that CO can be completely converted to CH4 and other intermediates, the produced methane (0.56 ± 0.09 mmol g−1 VSS) was lower than the theoretical value (1.34 ± 0.04 mmol g−1 VSS). This observation is attributable to that many intermediates were simultaneously generated from CO during the methanation process. At 109.5 h, the intermediates have not been completely utilized.
From the above results, it can be concluded that AGS possessed a great potential for the conversion of CO and CO2 to methane. And supplementation of H2 to CO as substrate might lead to the accumulation of VFAs.
Effect of CO partial pressure on methane production by AGS
Fig. 2 shows the relationship between PCO and methane production from CO. In general, more methane was accumulated when PCO was lower than 0.2 atm at the initial stage (within 96 h). Being similar with other reports,12,31 the methane production rate was found to be obviously inhibited when PCO was higher than 0.4 atm. When the pressure was 0.4 atm, the varying trend could be divided into two phases. During the 96 h after starting this test, the cumulative methane production from different reactors followed a descending order as reactors at 0.2 atm > 0.1 atm > 0.4 atm > 0.8 atm, illustrating that PCO ≥ 0.4 atm presented an inhibitory effect on methane production by AGS. After 96 hours' operation, due to that CO was gradually consumed by the microorganisms in the reactors, the partial CO pressure in the reactor gradually decreased and the inhibition was relieved to some extent. Therefore, the cumulative methane production under PCO of 0.4 atm exceeded those from 0.2 atm and 0.1 atm reactors. However, it was different from the flocculant digested sludge in which the methanogens were inhibited at a PCO of 0.25 atm.12 This observation reflects that the special dense structure of AGS is favorable for the resistance of CO inhibition. Along with the operation, the methane production at PCO > 0.4 atm gradually increased and even exceeded the control. This observation is possibly due to that CO was gradually consumed by the microorganisms in the reactor, and the partial CO pressure in the reactor gradually decreased and the inhibition was relieved to some extent, then CO could be used by the microorganisms and converted to methane. It has been speculated that CO in the anaerobic reactor can be first converted by bacteria into some intermediates and then produce CH4 and CO2 by methanogens.23 The CO consumptions at different partial pressures are shown in Fig. S3.†
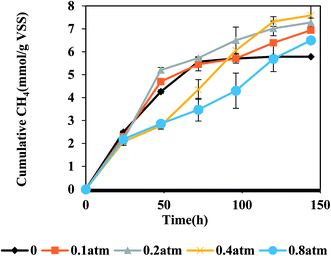 |
| Fig. 2 Effect of CO partial pressure (atm) on methane production from CO by AGS. | |
To explore the pathways from CO to methane and elucidate the effect of CO partial pressure on the intermediates produced, the gaseous components and VFAs were measured and recorded, respectively. Seen from Fig. 3(a), the concentrations of VFAs (129–247 mg C per L) on day 7 in all the bottles containing CO were obviously higher than that of the control (106 mg C per L), demonstrating an increasing trend with the increase in PCO. This observation indicates that VFAs could be produced from CO by bacteria when methanogens were inhibited by BES. The VFAs species and proportion of each individual VFA on day 7 were also examined. As seen from Fig. 3(b), no matter under which PCO condition, the dominant VFA was acetate ranging from 47% to 68%. From the control to 1 atm of CO, the second largest amount of individual VFA changed from isovaleric acid (18% in the control and 10% at 0.2 atm of PCO) to propionic acid (16% at 0.4 atm, 33% at 0.6 atm, 36% at 0.8 atm, 35% at 1 atm of PCO). This phenomenon can be explained from the following two aspects. (1) In the presence of BES, the fermentation products of organic matter contained in AGS (control) were VFAs, especially acetate and isovaleric acid in this study. (2) With the increase of substrate (CO) addition to the reactor, acetate and propionate were the main intermediates of the CO conversion, which is partially in agreement with the findings by Navarro et al.14,25 who identified an acetate-producing bacterium31 and a propionate producing bacterium32 after a long-time exposure to high CO concentrations. In addition, hydrogen was detected in the gas phase. CO has been claimed to be converted to methane via acetate (acetogenic CO-oxidizing pathway) as the precursor under mesophilic conditions, while via hydrogen (hydrogenotrophic CO-oxidizing pathway) under thermophilic conditions.26 Table 1 summarizes the related contents of acetate, propionate and H2. In this study, H2 was produced from all the PCO conditions, which might be the intermediate when PCO was higher than 0.2 atm in the headspace as a similar amount of H2 was detected in the control and the PCO at 0.2 atm reactors. It has been pointed out that the carboxydotrophic activity was insignificant when both inhibitors (BES and vancomycin) were present at the same time; still, H2 could be detected, illustrating that hydrogen-producing bacteria were not inhibited in the presence of the above two inhibitors.25 Among these three intermediates, acetate (reactions (4) and (5)) was considered as the major one due to its highest content; however, the H2/CO2 pathway (reactions (3) and (7)) may be co-existing.33
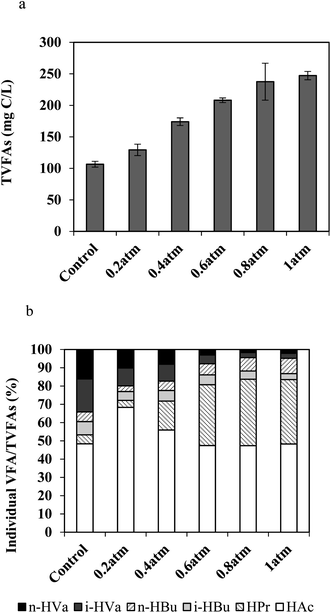 |
| Fig. 3 Effect of CO partial pressure (atm) on intermediates formation from CO in the presence of BES at 37 °C on day 7: (a) VFAs, and (b) percentage of individual VFA species to the total VFAs(TVFAs). | |
Table 1 Main products detected in the reactors under different CO partial pressures in the presence of BES
Main product |
PCO (atm) |
Control |
0.2 |
0.4 |
0.6 |
0.8 |
1 |
HAc (mmol g−1 VSS) |
1.07 ± 0.04 |
1.84 ± 0.03 |
2.07 ± 0.01 |
2.04 ± 0.06 |
2.34 ± 0.01 |
2.49 ± 0.03 |
HPr (mmol g−1 VSS) |
0.11 ± 0.02 |
0.10 ± 0.01 |
0.59 ± 0.02 |
1.44 ± 0.03 |
1.80 ± 0.02 |
1.82 ± 0.05 |
H2 (mmol g−1 VSS) |
0.02 ± 0.00 |
0.03 ± 0.01 |
0.08 ± 0.02 |
0.55 ± 0.04 |
0.75 ± 0.07 |
0.98 ± 0.03 |
The above results showed that when PCO < 0.2 atm, methane production from CO by AGS was not obviously affected. Conversely, PCO > 0.4 atm started to inhibit the activity of methanogens. The conversion of CO to methane was mainly via acetate as intermediate probably accompanied by the H2/CO2 pathway.
Effect of exogenous H2 partial pressure on methane production from blast furnace gas
High PCO presented inhibition on methane production, thus CO should be controlled at a low concentration in the anaerobic system. In this study, the CO partial pressure in BFG is about 0.22 atm, slightly higher than 0.2 atm which has been demonstrated to be a partial pressure without obviously inhibitory effect on methanogens. Due to the slight difference between 0.22 atm and 0.2 atm, the CO in BFG was not diluted in the experiments and its initial partial pressure was controlled at 0.22 atm in this test. The effect of exogenous H2 partial pressures on methane production from BFG by AGS is shown in Fig. 4(a). For the control, there was almost no lag phase which was similar with a previous report.34 Obviously, the lag period for methane production at PH2 = 0.88 atm and 1.54 atm was about 42 h, suggesting that the anaerobic microbes need some adaptation time to accommodate to the BFG and H2 environment. For BFG at PH2 of 0.04 atm, this lag period lasted 71 h, ending up with slightly more methane than the control. The methane production was 2.01 mmol g−1 VSS, 5.32 mmol g−1 VSS and 5.57 mmol g−1 VSS at PH2 of 0.04 atm, 0.88 atm and 1.54 atm, respectively. During the entire process, the highest methane production from BFG was obtained at PH2 of 1.54 atm, therefore addition of exogenous H2 favors methane generation. In addition, under the PH2 of 1.54 atm condition, only 4.7% higher methane production was obtained than that under PH2 of 0.88 atm condition, probably due to the formation of other intermediate products. The maximum H2, CO consumption and methane production rates are summarized in Table 2. The maximum CH4 production rate of 4.08 ± 0.71 mmol per g VSS per d and 4.19 ± 0.54 mmol per g VSS per d were both achieved under PH2 of 0.88 atm or 1.54 atm on day 3. The different inoculum or other conditions might result in the different time for achieving the maximum methane production rate. For example, in a continuous CO-converting reactor at 35 °C,20 the seeded anaerobic granules from a UASB plant treating fruit processing wastewater produced CH4 ranging from 0.49 ± 0.1 mmol per g VSS per d to 4.77 ± 1.21 mmol per g VSS per d during 100 days' operation, when operated at PCO in the gas feeding (CO: 30–60%) of (0.42–0.96) ± 0.6 atm and gas recirculation flow of 0–69 L h−1. As for the disaggregated sludge during 45 days' continuous CO injection to the headspace, its CH4 specific activity was 0.7 ± 1.3 mmol per g VSS per d on day 30 and 5.5 ± 1.2 mmol per g VSS per d on day 45, respectively.11 Even though the values obtained in this study are relatively lower than the above-mentioned 4.77 ± 1.21 mmol per g VSS per d and 5.5 ± 1.2 mmol per g VSS per d, it took only 3 days to reach the maximum methane production rate, far shorter than the 97 days and 45 days reported in the previous works.
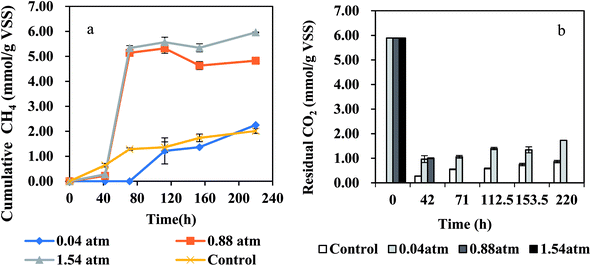 |
| Fig. 4 Effect of H2 partial pressure (atm) on BFG fermentation by AGS at 37 °C: (a) CH4, and (b) CO2. | |
Table 2 The maximum hydrogen and carbon monoxide consumptions, and methane production rates under different H2 partial pressures
PH2 |
CO consumption rate (mmol CO per g VSS per d) |
H2 consumption rate (mmol H2 per g VSS per d) |
CH4 production rate (mmol CH4 per g VSS per d) |
Control |
— |
— |
0.53 ± 0.01 |
0.04 atm |
1.67 ± 0.24 |
0.82 ± 0.05 |
0.70 ± 0.02 |
0.88 atm |
1.94 ± 0.12 |
16.34 ± 1.38 |
4.08 ± 0.71 |
1.54 atm |
1.90 ± 0.41 |
20.57 ± 0.96 |
4.19 ± 0.54 |
The H2 consumption rates under all tested groups increased with the increase in H2 partial pressure at a shaking speed of 100 rpm which may control the hydrogen gas–liquid mass transfer during the methanogenesis.35 Hydrogen gas–liquid mass transfer limitation has been commonly observed in anaerobic reactors.36 In this study hydrogen gas–liquid mass transfer was obviously the limiting factor, as the hydrogen consumption rate was proportional to initial PH2. As for the CO consumption rate, there was no significant difference between PH2 of 0.88 atm and 1.54 atm conditions, possibly due to its low concentration in BFG. In order to make the reactions (1)–(7) thermodynamically feasible, additional hydrogen which could react with CO2 is favorable for the CO consumption no matter in direct or indirect methane production from CO. CO2 in BFG was fully utilized at 71 h and 42 h when PH2 was at 0.88 atm and 1.54 atm, respectively (Fig. 4(b)). CO2 was consumed by 84% at 42 h when the initial PH2 was 0.04 atm, which later slightly increased and was not fully utilized, most probably due to the fact that CO2 was one of the products during the methanogenesis in the reactions (1), (3), (4), (6). This further suggests the necessity of exogenous hydrogen addition since it is beneficial for the consumption of CO and CO2 in BFG.
Esquivel-Elizondo et al.8 compared the effects of CO2 and H2 on CO metabolism using pure and mixed cultures (anaerobic sludge was acclimated to CO) and claimed that the main products were acetate and ethanol. In the pure cultures the additional H2 did not promote acetate production which was opposite to the phenomenon in the mixed culture. A possible explanation is that, CO dehydrogenase (CODH), the enzyme that catalyzes the reversible reduction of CO to CO2, possesses hydrogenase activity. Thus, the activity of hydrogenases in the pure culture of carboxidotrophs could be redundant. Previous studies with pure cultures also reported that H2 was not consumed along with CO.35,36 In this study, the target product was methane by using AGS (mixed culture). From the changes in VFAs species under different PH2 conditions (Fig. S4†), the co-existence of H2 may influence the balance between VFAs production and consumption to some extent. At initial PH2 of 1.54 atm, not only some increase in methane production (Fig. 4) but also VFAs accumulation were detected. This phenomenon may explain why only a slight increase (4.7%) in methane production was achieved at PH2 of 1.54 atm in comparison to PH2 of 0.88 atm condition. Another two reactions ((8) and (9)) can also be used to explain the changes in VFAs under different PH2. In order to make the reactions (8) and (9) thermodynamically feasible, low hydrogen concentration needs to be maintained during the degradation of propionate and butyrate.37
|
CH3CH2CH2COOH + 2H2O → 2CH3COOH + 2H2, ΔG0 = 45.4 kJ mol−1
| (8) |
|
CH3CH2COOH + 2H2O → CH3COOH + 3H2 + CO2, ΔG0 = 72.7 kJ mol−1
| (9) |
In this study, VFAs degradation seemed to be inhibited at PH2 up to 1.54 atm, which was mainly composed of acetic acid, butyric acid and valeric acid. Meanwhile, the remaining VFAs species and their concentrations under PH2 = 0.88 atm were almost similar with those of the control. This observation suggests that proper exogenous hydrogen can improve the biomethanation of BFG by using AGS.
Carbon balance
To monitor the anaerobic process and compare the participation of the main substances related to BFG fermentation, the carbon balance was also analyzed in this work (Fig. 5). During the biomethanation of BFG using AGS, carbon from VSS of AGS and BFG (CO and CO2) may be converted to products including methane, carbon dioxide, VFAs and other substances. As seen from Fig. 5, all CO under different tested conditions were consumed within 7 days, producing methane and VFAs as the major intermediate carbon products under higher PH2 conditions (0.88 and 1.54 am), and the percentage of methane-C was found to increase with the increase of PH2 (around 28%, 49%, and 52% at 0.04 atm, 0.88 atm, and 1.54 atm, respectively). Noticeably, CO2 was not fully utilized at PH2 of 0.04 atm, most probably due to its insufficient hydrogen. Restated, a slight increase in methane production was detected under PH2 of 1.54 atm in comparison to 0.88 atm, due to its higher accumulation of VFAs (19%) in the fermentation systems.
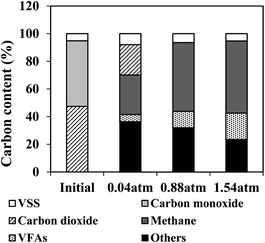 |
| Fig. 5 Carbon balance analysis for the BFG fermentation by AGS at different H2 partial pressures on day 7. | |
Conclusions
This work indicates that AGS possesses high potential for anaerobic conversion of CO from BFG to methane via VFAs (especially acetate) or H2 as intermediates under mesophilic conditions. By using the simulated BFG, the batch tests demonstrated that either CO or CO2 from BFG could be effectively converted by supplying exogenous hydrogen under an appropriate PH2 (0.88 atm in this study). Although PH2 of 1.54 atm could rapidly convert carbon source in BFG to methane, the accumulation of VFAs implies that additional design and operation should be considered for the whole BFG fermentation system.
Conflicts of interest
There are no conflicts exist.
Acknowledgements
This work was financially supported by JFE steel cooperation.
References
- GIO (The Greenhouse Gas Inventory Office of Japan), National Greenhouse Gas Inventory Report of JAPAN, 2017 Search PubMed.
- K. Goto, H. Okabe, F. A. Chowdhury, S. Shimizu, Y. Fujioka and M. Onoda, Int. J. Greenhouse Gas Control, 2011, 5, 1214–1219 CrossRef.
- B. Molitor, H. Richter, M. E. Martin, R. O. Jensen, A. Juminaga, C. Mihalcea and L. T. Angenent, Bioresour. Technol., 2016, 215, 386–396 CrossRef PubMed.
- X. Paubel, A. Cessou, D. Honore, L. Vervisch and R. Tsiava, Proc. Combust. Inst., 2007, 31, 3385–3392 CrossRef.
- P. C. Munasinghe and S. K. Khanal, Bioresour. Technol., 2010, 101, 5013–5022 CrossRef PubMed.
- E. Oelgeschläger and M. Rother, Arch. Microbiol., 2008, 190, 257–269 CrossRef PubMed.
- W. H. Chen, M. R. Lin, T. S. Leu and S. W. Du, Int. J. Hydrogen Energy, 2011, 36, 11727–11737 CrossRef.
- S. Esquivel-Elizondo, A. G. Delgado, B. E. Rittmann and R. Krajmalnik-Brown, Biotechnol. Biofuels, 2017, 10, 220 CrossRef PubMed.
- Y. Rao, J. Wan, Y. Liu, I. Angelidaki, S. Zhang, Y. Zhang and G. Luo, Water Res., 2018, 139, 372–380 CrossRef PubMed.
- M. Köpke, C. Mihalcea, F. Liew, J. H. Tizard, M. S. Ali, J. J. Conolly, B. Al-Sinawi and S. D. Simpson, Appl. Environ. Microbiol., 2011, 77, 5467–5475 CrossRef PubMed.
- M. Balat, Int. J. Hydrogen Energy, 2008, 33, 4013–4029 CrossRef.
- G. Luo, W. Wang and I. Angelidaki, Environ. Sci. Technol., 2013, 45, 2006–2012 Search PubMed.
- E. C. Bugante, Y. Shimomura, T. Tanaka, M. Taniguchi and S. Oi, J. Ferment. Bioeng., 1989, 67, 419–421 CrossRef.
- S. S. Navarro, R. Cimpoia, G. Bruant and S. R. Guiot, Front. Microbiol., 2016, 7, 1–13 Search PubMed.
- Y. Zhang, J. Liu, W. Ding and X. Lu, Fuel, 2011, 90, 324–330 CrossRef.
- S. Kim, K. Choi and J. Chung, Int. J. Hydrogen Energy, 2013, 38, 3488–3496 CrossRef.
- Y. Hu, X. Hao, D. Zhao and K. Fu, Chemosphere, 2015, 140, 34–39 CrossRef PubMed.
- C. W. Nam, K. A. Jung and J. M. Park, Bioresour. Technol., 2016, 211, 478–485 CrossRef PubMed.
- S. Esquivel-Elizondo, A. G. Delgado, B. E. Rittmann and R. Krajmalnik-Brown, Biotechnol. Biofuels, 2017, 10, 220 CrossRef PubMed.
- C. F. Shen, N. Kosaric and R. Blaszczyk, Appl. Microbiol. Biotechnol., 1993, 39, 132–137 CrossRef.
- F. A. Macleod, S. R. Guiot, J. W. Costerton and C. Hp, Appl. Environ. Microbiol., 1990, 56, 1598–1607 Search PubMed.
- Y. Liu, J. Wan, S. Han, S. Zhang and G. Luo, Bioresour. Technol., 2016, 202, 1–7 CrossRef PubMed.
- S. R. Guiot, R. Cimpoia and G. Carayon, Environ. Sci. Technol., 2011, 45, 2006–2012 CrossRef PubMed.
- Y. Zhang, Z. Zhang, K. Suzuki and T. Maekawa, Biomass Bioenergy, 2003, 25, 427–433 CrossRef.
- S. S. Navarro, R. Cimpoia, G. Bruant and S. R. Guiot, Can. J. Microbiol., 2014, 60, 407–415 CrossRef PubMed.
- J. Sipma, P. N. L. Lens, A. J. M. Stams and G. Lettinga, FEMS Microbiol. Ecol., 2003, 44, 271–277 CrossRef PubMed.
- L. Maya-Altamira, A. Baun, I. Angelidaki and J. E. Schmidt, Water Res., 2008, 42, 2195–2203 CrossRef PubMed.
- J. Sipma, R. J. W. Meulepas, S. N. Parshina, A. J. M. Stams, G. Lettinga and P. N. L. Lens, Appl. Microbiol. Biotechnol., 2004, 64, 421–428 CrossRef PubMed.
- H. Heiskanen, I. Virkajärvi and L. Viikari, Enzyme Microb. Technol., 2007, 41, 362–367 CrossRef.
- L. Appels, J. Baeyens, J. Degrève and R. Dewil, Prog. Energy Combust. Sci., 2008, 34, 755–781 CrossRef.
- M. Braun and G. Gottschalk, Zentralblatt für Bakteriologie Mikrobiologie und Hygiene: I. Abt. Originale C: Allgemeine, angewandte und ökologische Mikrobiologie, 1982, 3, 368–376 CrossRef.
- A. T. Johns, J. Gen. Microbiol., 1952, 6, 123–127 CrossRef PubMed.
- Y. Jing, S. Campanaro, P. Kougias, L. Treu, I. Angelidaki, S. Zhang and G. Luo, Water Res., 2017, 126, 19–28 CrossRef PubMed.
- R. Z. Gaur and S. Suthar, J. Cleaner Prod., 2017, 164, 557–566 CrossRef.
- G. Luo, S. Johansson, K. Boe, L. Xie, Q. Zhou and I. Angelidaki, Biotechnol. Bioeng., 2012, 109, 1088–1094 CrossRef PubMed.
- A. Pauss, G. Andre, M. Perrier and S. R. Guiot, Appl. Environ. Microbiol., 1990, 56, 1636–1644 Search PubMed.
- V. Siriwongrungson, R. J. Zeng and I. Angelidaki, Water Res., 2007, 41, 4204–4210 CrossRef PubMed.
Footnotes |
† Electronic supplementary information (ESI) available. See DOI: 10.1039/c8ra04853c |
‡ These authors contributed equally to this work. |
|
This journal is © The Royal Society of Chemistry 2018 |