DOI:
10.1039/C8RA03923B
(Paper)
RSC Adv., 2018,
8, 38483-38498
Toxicity of fungicides to Pisum sativum: a study of oxidative damage, growth suppression, cellular death and morpho-anatomical changes
Received
8th May 2018
, Accepted 1st November 2018
First published on 15th November 2018
Abstract
Considering the fungicidal threat to the sustainable agro-environment, the toxicological impacts of three fungicides, namely kitazin, hexaconazole and carbendazim, on the biological, chemical and morpho-anatomical changes of peas were assessed. Fungicide applications in general caused a slow but gradual reduction in growth, symbiosis and yields of peas, which, however, varied appreciably among species and concentrations of the three fungicides. Of the three fungicides, carbendazim had the most lethal effect, in which it delayed seed germination and also diminished the overall pea growth. Carbendazim at 3000 μg kg−1 maximally reduced the germination, SVI, size of roots and shoots and total dry matter accumulation in roots, shoots and whole plants distinctly by 40%, 84%, 72%, 73%, 68%, 75% and 73% (p ≤ 0.05), respectively. Hexaconazole at 120 μg kg−1 significantly (p ≤ 0.05) declined total chlorophyll, carotenoids, grain yields, grain protein, root P and shoot N by 19%, 28%, 46%, 69%, 48% and 51%, respectively, over the control. The synthesis of stress biomarkers and oxidative stress were increased with increasing dosage rates of fungicides. Proline content in roots, shoots, leaves and grains, MDA, electrolyte leakage and H2O2 of plants grown in soil treated with 288 μg kg−1 kitazin were increased significantly (p ≤ 0.05) by 73%, 52%, 41%, 24%, 59%, 40% and 27%, respectively, relative to the control. Antioxidant defence enzymes were greater in pea foliage. The SEM and CLSM images revealed an obvious alteration in root tips, enhanced cellular damage and cell death when plants were raised under fungicide stress. Also, morpho-anatomical variations in fungicide-treated foliage were visible in the SEM images. Overall, the present study suggests that a careful and secure strategy should be adopted before fungicides are chosen for enhancing pulse production in different agro-climatic regions.
1. Introduction
Pea (Pisum sativum L.) is one of the most widely cultivated crops and is grown both as a vegetable and as a pulse crop. Globally, it is grown in an area of 1.1 million ha with a total production of 9.2 million tonnes with a productivity of 8.35 tonnes per ha.1 Nutritionally, it contains high concentrations of protein, carbohydrates, vitamins and minerals (Fe, Ca, K and P), which make peas a valuable human dietary component.2 Also, due to low fats, sodium and cholesterols, it is used to prevent cardiovascular diseases.3 When grown in different parts of the world, this crop faces many challenges from weeds and insects,4 phytopathogens5 and environmental variables.6 Chief among these are the diseases caused by various phytopathogens that can lead to severe losses in crop yields.5 So, to protect peas from fungal damage and consequently to maintain their nutritional composition, various synthetic fungicides,7 for example, carbendazim, mancozeb, kitazin, hexaconazole, thiram, are applied indiscriminately in farming practices.8 The majority of these fungicides when applied, however, persist in soil and become non-biodegradable.9 Such fungicides in turn destruct the soil fertility,10 leading eventually to losses in the growth, symbiotic attributes and yields of legumes.11 Some fungicides even abolish nodulation and the N2-fixation processes of several grains and forage legumes. For instance, hexaconazole, a broad-spectrum systemic fungicide, is used to manage phytopathogenic fungi12 on the one hand, while it negatively affects the BNF, ureide levels, N transformation and overall performance of leguminous plants on the other hand.13,14 Also, it decreases chlorophyll and carotenoid pigment formation by legumes, for example, Phaseolus vulgaris.15 In yet another study, the fungicide pyrimorph has been found to strongly inhibit the electron-transport reactions of chloroplasts and thus damages the physiological machinery of whole plants.16 These fungicides act as multiple site inhibitors and have various types of toxic action on cells, for example chelation and the formation of mixed disulfide bonds transport across the membranes. Dialkyldithiocarbamates inhibit a widespread fungal enzyme, but the scheme of pyruvic dehydrogenase is predominantly delicate to such type of fungicides.17 Also, these anti-fungal compounds are reported to increase the ratios of NAD and NADP, thus interfering with the electron-transport system (ETS) and increasing the ATP levels by inducing change in enzymes system, which results in the conservation of leaf and grain protein and chlorophyll in plants.
Additionally, the application of systemic fungicides, for example, benzimidazoles, triazole and dithiocarbamate have been reported to affect the effective quantum yield of PSII (ΦPSII) and the maximal quantum efficiency of PSII (Fv/Fm) of plants. Since the growth and overall performance of plant vigour depends on photosynthesis to assimilate C, the impairment of photosynthesis will have destructive impacts on biomass production and thus concurrently on the yields of plants. Fungicides-induced toxicity to plants also generates oxidative stress and leads to the accumulation of reactive oxygen species (ROS). The ROS so deposited inside plant tissues in turn disrupt the membrane integrity, leading eventually to the leakage of electrolytes outside the cell.18 Such a continued leakage of essential ions from inside to the exterior environment ultimately results in the death of plants. Taking into consideration the critical risks associated with the application of fungicides in legume production, the current study employed peas as a test crop with an aim to: (i) evaluate the biotoxic impact of kitazin, hexaconazole and carbendazim on the chemical and biological properties, and grain features, (ii) estimate the influence of fungicides on symbiotic characteristics, photosynthetic pigments and nutrient uptake, (iii) assess the responses of fungicides on stress biomarkers, oxidative stress and antioxidant defence enzymes and (iv) estimate the deleterious impact of fungicides on leaf anatomy, structural distortion, cytotoxicity and cellular damage.
2. Materials and methods
2.1. Fungicide application and growth conditions
Vigorous and undeviating sized seeds of P. sativum (var. Arkil) obtained from local seed markets were surface sterilized with 70% ethanol for 3 min, followed by 4% sodium hypochlorite (3 min), and then washed repetitively six times with six changes of sterile water and finally dried at room temperature. Properly sterilized seeds (eight seeds per pot) were sown in earthen pots (22 cm high and 25 cm internal diameter) containing 4 kg unsterilized alluvial sandy clay loam soils (sand 667 g kg−1, silt 190 g kg−1, clay 143 g kg−1, organic matter 6.2 g kg−1 0.4%, Kjeldahl N 0.75 g kg−1, Olsen P 16 mg kg−1, pH 7.2 and WHC 0.44 mL g−1, CEC 11.7 and 5.1 cmol kg−1 AEC). A total of 10 treatments with one untreated control and three concentrations (normal, double and three times more) of kitazin, hexaconazole and carbendazim were added pre-sowing to each individual pot. The normal dosage rates (1×) of fungicides were (μg kg−1): 96 (kitazin: a.i. 48% EC, CAS No. 26087-47-8; M.W: 260.288 g mol−1, P.I. Industries Ltd., Rajasthan, India), 40 (hexaconazole: a.i 5% SC, CAS No. 79983-71-4; M.W: 314.21 g mol−1, Parijat Agrochemicals, New Delhi, India) and 1000 (carbendazim: a.i 50% w/w, CAS No. 10605-21-7; M.W: 191.19 g mol−1, Parijat Agrochemicals, New Delhi, India). The fungicides for application were prepared in double-distilled water (DDW). Each individual experiment was performed in triplicate (n = 3) and all the treated and untreated pots were set up in a completely randomized block design (CRD). Pots were carefully thinned 15 days after emergence and three plants were maintained in each individual pot. The experimental pots were irrigated regularly with tap water and were kept in an open field condition (day/night: 25/15 °C, 55–60% relative humidity, photoperiod of 9 h light/14 h dark, and light irradiance of 150 mol m2 s−1). The whole study was performed for two successive years and each individual experiment with identical/similar treatment was repeated for two consecutive years to validate the reproducibility and accuracy of the data.
2.2. Germination efficiency and seedling vigour index
Healthy seeds of P. sativum were surface sterilized using 3% (w/v) sodium hypochlorite (NaOCl) for 3 min, followed by three successive washings with DW. Five days after sowing (DAS), the radicle emergence of one millimetre was considered as a germinated seed and the germination percentage was calculated as:
The percentage of germination was used further to calculate the seedling vigour index:19
seedling vigor index (SVI) = [root length + shoot length] × % seed germination |
2.3. Toxic impacts of fungicides on the biological parameters of P. sativum
2.3.1. Dry matter accumulation and estimation of the photosynthetic pigments. All plants (three plants) in one pot for each treatment were uprooted at 90 DAS, while six plants in the two remaining pots were uprooted at 130 DAS. The uprooted plants were carefully washed and oven-dried, and the dry matter was subsequently determined. Chlorophyll content was measured at 90 DAS according to the method of Arnon.20 The pigments were extracted from fresh leaves by macerating in 80% acetone. Absorption of the chlorophyll and carotenoids present in the extract was determined using a UV visible spectrophotometer (UV-2450, Shimadzu, Tokyo, Japan). The total photosynthetic pigments (chl a, b and total chlorophyll) were calculated as:
mg chl a per g tissue = 12.7 (A663) − 2.69 (A645) × V/1000 × W |
mg chl b per g tissue = 22.9 (A645) − 4.68 (A663) ×V/1000 × W |
mg total chl per g tissue = 20.2 (A645) + 8.02 (A663) × V/1000 × W |
The carotenoid content was determined by the formula as suggested by Krik and Allen21:
carotenoids (mg g−1 tissue) = (A480) + 0.114 (A663) − 0.638 (A645) |
where
Aλ = absorbance at specific wavelength
λ (nm);
V = final volume of chlorophyll extraction in 80% acetone and
W = fresh weight of tissue extract.
2.3.2. Symbiotic attributes, nutrient uptake and grain features. Nodules detached at 90 DAS and 130 DAS from the root systems of untreated (control) and fungicides-treated P. sativum plants were counted, oven-dried at 80 °C and the nodule dry biomass was recorded. The leghaemoglobin (LHb) content in fresh nodules recovered from the root system of P. sativum at 90 DAS and at harvest was assayed by the method of Sadasivam and Manickam.22 Briefly, fresh nodules were crushed with the help of a mortar and pestle in 5 mL sodium phosphate buffer (pH 7.4) and filtered through two layers of cheese cloth. The nodule debris was discarded. The turbid reddish-brown filtrate was clarified by centrifugation at 10
000g for 30 min. The supernatant was diluted to 10 mL with sodium phosphate buffer (pH 7.4). The extract was divided equally into two glass tubes (5 mL per tube) and an equal amount of alkaline pyridine reagent was added to each tube. The haemachrome formed was read at 556 and 539 λ after adding a few crystals of potassium hexacyanoferrate (K3FeCN6) and sodium dithionite (Na2S2O4), respectively. The leghaemoglobin content was calculated using the formula:
LHb content (mM) = [λ556 − λ539] × 2D/23.4, where D = initial dilution |
The total P and N content in the roots and shoots of P. sativum removed at harvest (130 DAS) were measured by the method of Jackson23 and Lindner,24 respectively. Grain features, such as the formation of seeds (seed yield) and seed protein,25 were estimated at harvest.
2.4. Oxidative stress and antioxidant enzymes
2.4.1. Measurements of proline accumulation. The proline content in roots, shoots, leaves (at 90 DAS) and seeds (at harvest) of P. sativum grown both in fungicides-enriched and conventional soil (untreated) was determined by the method of Bates et al.26 Briefly, 1 g fresh plant organs were homogenized with 5 mL of 3% (w/v) aqueous sulfosalicylic acid (C7H6O6S). The resulting homogenate was filtered through Whatman No. 2 filter paper. The resulting cell extract was then centrifuged at 8000 rpm for 20 min to remove cell debris. The cell filtrate (2 mL) with free proline was treated with acid ninhydrin (2 mL) and glacial acetic acid (2 mL) at 80 °C for 1 h. The reaction was terminated in an ice bath. Coloured complex was extracted in 4 mL toluene and absorbance was recorded at 520 nm. A proline standard was prepared by dissolving proline in 3% (w/v) sulfosalicylic acid.
2.4.2. Measurement of leaf electrolyte leakage, H2O2 accumulation and MDA extraction. The total inorganic ions leaked out of the leaves were measured at 90 DAS by the method as described by Ismail and Hall.27 First, 0.5 g of leaves were taken in a boiling test tube containing 10 mL of deionized water and the electrical conductivity (EC) was measured (ECa). The contents were heated at 45 °C and 55 °C for 30 min each in a water bath and the EC was measured (ECb). Later, the controls were boiled at 100 °C for 10 min and the EC was again recorded (ECc). The electrolyte leakage was calculated using the formula:
electrolyte leakage (%) = [(ECb − ECa)] × 100/(ECc) |
Hydrogen peroxide (H2O2) accumulated within foliage tissues was determined at 90 DAS by the method of Patterson et al.28 For this, 500 mg plant sample was homogenized in 3 mL of 50 mM phosphate buffer (pH 6.8). The homogenate was centrifuged at 6000 × g for 25 min. A total of 3 mL extract was mixed with 0.1% titanium chloride prepared in 20% (v/v) H2SO4 and the mixture was again centrifuged at 6000 × g for 15 min. The absorbance of the colour was read at 410 nm. The H2O2 content was computed on a fresh mass basis using a standard curve of known H2O2 concentration.
Lipid peroxidation levels (MDA content) were assessed using malondialdehyde (MDA) according to the method described by Ahmed et al.29 Fresh leaves (500 mg) were homogenized using a prechilled mortar and pestle with 10 mL 5% (w/v) trichloroacetic acid (TCA), and centrifuged at 12
000 × g for 20 min at 4 °C. The supernatant (2 mL) was added to a tube containing 2 mL 0.67% (w/v) TBA. This tube was heated in a water bath at 100 °C for 30 min, rapidly cooled to 4 °C in an ice bath and then centrifuged at 10
000 × g for 10 min at 4 °C. The absorbance of the supernatant was recorded at 532 nm and 600 nm. The MDA content was calculated using the extinction coefficient of 155 mM−1 cm−1:
MDA level (μmol L−1) = 6.45 × (λ532 − λ600) − 0.56 × λ450 |
2.4.3. Extraction and determination of activities of antioxidant enzyme. Antioxidant enzymes, such as GPX, POD and APX, in the foliage tissues of P. sativum were determined at 90 DAS. For guaiacol peroxidase (GPX, E.C. 1.11.1.7), foliage tissues (100 mg) were homogenized in tris buffer and the homogenate was centrifuged at 12
000 rpm for 20 min at 4 °C. GPX activity was determined in the enzyme extract according to the method of Zhang et al.30 The increase in absorbance at 470 nm due to the formation of tetra guaiacol (ε = 26.6 mM−1 cm−1) was expressed as μmol mg protein−1 min−1. POD and APX activities were determined by the methods of Leonard et al.31 and Hammerschmidt et al.,32 respectively.
2.5. Root tip distortion, intracellular root damage and cell viability
The toxic and damaging impact of fungicides on the root morphology of P. sativum plants grown on soft agar plates for 7 days in the presence of 1000 μg mL−1 each of kitazin, hexaconazole and carbendazim was monitored under SEM (JSM 6510 LV, JEOL, Japan). Briefly, root samples were washed three times with PBS, fixed for 12 h in 2% (v/v) glutaraldehyde prepared in 0.1 M phosphate buffer (pH 7.0), washed three times with the same buffer and dehydrated in a graded series of ethanol (30%, 50%, 70%, 90% and 100%) at 4 °C. Samples were dried in a critical point dryer (CPD), fixed in gold stubs and finally viewed under SEM.33
To evaluate the damage caused by fungicides, the roots of P. sativum plants grown for 7 days on soft agar plates amended with 0 (untreated control), 1×, 2× and 3× concentrations each of kitazin, hexaconazole and carbendazim were used. After successive washing with PBS, the root samples were stained with a mixture of acridine orange (AO) and propidium iodide (PI) and mounted on a glass slide. The stained roots were observed for increasing dead tissues with progressively increasing fungicide concentrations under an LSM-780 Confocal Microscope (Zeiss, Germany). The loss of cell viability was determined using the Evans blue staining method as previously described by Shahid and Khan.34 Briefly, fungicide-treated roots were stained with Evans blue (0.25% w/v) dye for 15 min and successively washed with distilled water for 30 min. Samples were then viewed under CLSM to assess cell death. The fluorescence intensity of the root tip, meristematic zone and elongation zone were quantified using the Leica application suite (LASAF lite 2.6.0) software. Five roots per treatment (n = 5) were taken for analysis and the intensity counts, averaged and presented as the mean ± S.D. as a function of fungicide concentration. Three channels for a single region of interest (ROI) were measured using an inbuilt histogram tool of LASAF lite and the mean intensity curves were plotted for three distinctive zones of roots.
2.6. Statistical analysis
All experiments were performed in a randomized design with three replicates (n = 3) of each individual treatment for two consecutive years. The two-year data obtained for P. sativum plants were pooled and the mean separation within columns was analyzed. All data represented are the mean ± standard deviation (SD) of three replicates (n = 3) of each treatment. Different letters show a significant difference between treatments at p ≤ 0.05. Also, the least significant difference (LSD) among the treatment means was calculated by two-way ANOVA (analysis of variance) at p ≤ 0.05 using the Minitab 17 statistical program.
3. Results and discussion
3.1. Seed germination and vigour index
Germination is indeed a vital phenomenon during the life cycle of any plant. Hence, seed germination and the seedling vigour index are considered the most significant physiognomies of the seeds to be used for cultivation. Seeds that germinate rapidly and vigorously in favourable conditions under controlled environments are likely to produce vigorous seedlings in fields also. In this study, the germination efficiency of P. sativum seeds sown in soils treated differently with varying doses of fungicides differed appreciably (Fig. 1A). Seeds were sown in controlled conditions (untreated) germinated to the maximum level of 95%. Among the normal, double- and three-times concentrations of fungicides, the three times more doses each of kitazin, hexaconazole and carbendazim in general caused a maximum decrease in seed germination and SVI. For example, kitazin (288 μg kg−1), hexaconazole (120 μg kg−1) and carbendazim (3000 μg kg−1) significantly (p ≤ 0.05) reduced the seed germination efficiency by 15%, 20% and 40%, whereas the SVI was reduced significantly (p ≤ 0.05) by 54%, 62% and 84%, respectively, in comparison to the untreated control (Fig. 1B). The performance of some pesticides particularly systemic fungicides in a soil system shows that higher concentrations of fungicides require more time to degrade and hence are reported to show that the higher (3×) dose of fungicides have harmful effects on seed germination, SVI and other plant parameters. Similarly, the toxic and depressive effect of glyphosate on the germination efficiency of Dimorphandra wilsonii, a member of the Fabaceae family, has recently been reported.35
 |
| Fig. 1 Toxic impact of fungicides (μg kg−1): kitazin at 96 (K1); 192 (K2) and 288 (K3); hexaconazole at 40 (H1) 80 (H2) and 120 (H3) and carbendazim at 1000 (C1), 2000 (C2) and 3000 (C3) on various biological characteristics of P. sativum; (A) indicates the per cent seed germination, (B) represents the seedling vigour index, (C) depicts the length of plant organs (root and shoot) at different stages, (D) indicates the fresh weight of plant organs and (E) shows the dry matter accumulation within the plants removed at 90 and 130 DAS, and (F) indicates the total dry matter accumulation. Each value is a mean of three replicates, where each replicate constituted three plants per pot. Mean values followed by different letters are significantly different at p ≤ 0.05. according to DMRT test. Vertical bars represent the mean ± SD (n = 3). ANOVA significant at p ≤ 0.05. Error bars represent S.D. While, df represents the degree of freedom. | |
3.2. Biological characteristics of P. sativum under the influence of fungicides
3.2.1. Length, dry biomass and photosynthetic pigments. The impact of different concentrations of kitazin, hexaconazole and carbendazim on the bio-features of P. sativum was variable (Fig. 1). The harshness of fungicides was moderately lower at 1× and 2× concentrations of each fungicide. However, at the highest concentrations (3×), even though the lethality was more pronounced, the toxicity to the measured parameters decreased with the advancing age of plants. For instance, the root length was decreased significantly (p ≤ 0.05) by 46%, 49% and 69% when pea plants were grown with 288 μg kg−1 kitazin, 120 μg kg−1 hexaconazole and 3000 μg kg−1 carbendazim, respectively, over the untreated control at 90 DAS (Fig. 1C). Whereas, at 130 DAS, the root length declined by 27%, 12% and 56% over the control at the same rate of kitazin, hexaconazole and carbendazim, respectively. Among all fungicides, the three times greater concentration of carbendazim had the most lethal impact on the measured parameters compared to hexaconazole (at 120 μg kg−1) and kitazin (at 288 μg kg−1). Similarly, dry matter production declined progressively with consistently increasing concentrations of fungicides. For example, a maximum reduction of 73% (at 90 DAS) and 70% (130 DAS) in the dry biomass of P. sativum was observed at a three times greater concentration of carbendazim relative to the untreated control. A trend similar to carbendazim was observed for hexaconazole and kitazin. While comparing the dry matter accumulation in shoot tissues, the maximum (69%) and minimum (13%) decrease in shoot dry biomass was observed at 3000 μg kg−1 of carbendazim and 40 μg kg−1 of hexaconazole, respectively, over the untreated control (Fig. 1E and F). The drop in dry biomass of pea crops upon the application of fungicide may probably be due to the modification/inhibition of several enzymes involved in the growth, development, physiology and metabolic activities of plants. In a similar study, the phytotoxic impact of higher doses of pesticide on the biological parameters of Lupinus was reported.36 The uptake of fungicides by plants occurs primarily through the root systems from where they are translocated to aerial organs, such as leaves and fruits. Once taken up by roots, these fungicides are translocated to the shoots either through xylem or phloem, or in roots via the phloem alone. Following the pesticide uptake, there could be an undeniable reduction in the growth of various plant organs. Another reason for the reduction in the growth of plants may probably be the unavailability of humic acids, fulvic acids, organic and inorganic matter present in the soil system due to the interruption of fungicides.Photoreceptor pigments (chlorophylls and carotenoids) play an important role in the formation of organic molecules, such as carbohydrates and proteins.37 Under abiotic stress conditions, the chlorophyll content and other photosynthetic pigments of plants, however, generally decreases.38 In our study, the photosynthetic pigments in the fresh foliage of P. sativum declined constantly with an independent increase in fungicide concentration (Fig. 2). A steady decline in the photosynthetic pigments was recorded as the concentration of each pesticide increased. Among fungicides, the three times greater rate of carbendazim decreased the chl a, b, total chl and carotenoid contents maximally by 35%, 42%, 32% and 38%, respectively. Similarly, other fungicides had a destructive impact on the measured photosynthetic pigments of pea plants. Likewise, the carotenoid contents in the fresh foliage of P. sativum plants grown with 288, 120 and 3000 μg kg−1 kitazin, hexaconazole and carbendazim were diminished by 16%, 28% and 38%, respectively, with respect to the control. In an identical experiment, Mourad et al.15 reported that the application of systemic fungicide hexaconazole decreased the chlorophyll and carotenoid pigments of Phaseolus vulgaris. Pesticides, including fungicides, adversely affect the metabolic enzymes involved in chlorophyll/carotenoid synthesis. Therefore, it seems probable that the fungicides used in this study might have inhibited the functioning of the enzymes of the photosynthetic carbon reduction (PCSR) cycle, such as Rubisco, 3PGA kinase, NADP, NAD-glyceraldehyde-3-P dehydrogenase and aldolase. Additionally, in a finding, Parween et al.39 described that Vigna radiata L. under the influence of pesticide displayed a decline in the growth attributes with increasing concentration, showing a reduction in the number and area of the leaf, which might be due to hinderance in the translocation of the photosynthetic rate and the reduction in chlorophyll content. The investigation of numerous photosynthetic pigments and fluorescence parameters of plants raised in fungicides-stressed environments40 validated that the light reactions of photosynthesis are also very sensitive to the exposure of these chemicals.
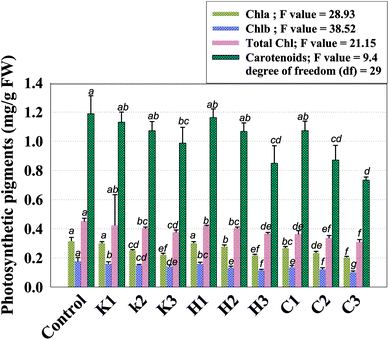 |
| Fig. 2 Toxic influence of fungicides (μg kg−1): kitazin at 96 (K1); 192 (K2) and 288 (K3); hexaconazole at 40 (H1) 80 (H2) and 120 (H3) and carbendazim at 1000 (C1), 2000 (C2) and 3000 (C3) on photosynthetic pigments (chl a, chl b, total chl and carotenoid content) of P. sativum plants. Each value is a mean of three replicates, where each replicate constituted three plants per pot. Mean values (±) followed by different letters are significantly different at p ≤ 0.05 according to DMRT test. Vertical bars represent the mean ± SD (n = 3). ANOVA significant at p ≤ 0.05. Error bars represent S.D. While, df represents the degree of freedom. | |
3.2.2. Symbiotic attributes, grain features and nutritional content. The symbiotic attributes, such as nodule number, nodule dry biomass and leghaemoglobin (LHb) content, in fresh nodules, recovered from P. sativum plants uprooted at 90 and 130 DAS, declined continually with an independent increase in fungicide concentrations (Fig. 3E and F). For instance, compared to the untreated control, the nodule number, nodule biomass and LHb contents measured at 90 DAS decreased by 51%, 50% and 38%, respectively, at 120 μg kg−1 concentration of hexaconazole, respectively (Fig. 3F); whereas, significant (p ≤ 0.05) declines of 77%, 65% and 69%, were observed in the nodule number, nodule biomass and LHb content, respectively, in carbendazim (3000 μg kg−1)-treated plants over the control. Similarly, a reduction in the symbiotic attributes of herbicides-treated greengram plants has recently been reported.41 The deleterious impact of fungicides on the symbiotic parameters might be due to the deterioration of growth regulatory enzymes involved in the progression and improvements of legumes or due to the distraction of signalling between phyto-chemicals, for instance, luteolin, apigenin and Nod D receptors, which are the principal components for the initiation of nodule formation and nitrogen fixation.42
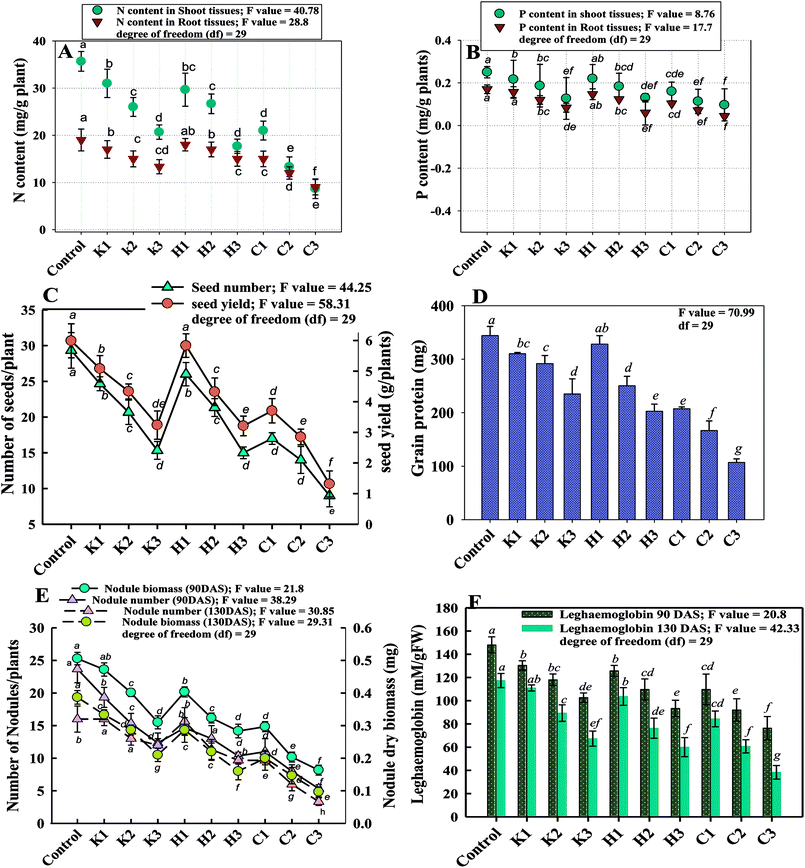 |
| Fig. 3 Inhibitory effect of fungicides (μg kg−1): kitazin at 96 (K1); 192 (K2) and 288 (K3); hexaconazole at 40 (H1) 80 (H2) and 120 (H3) and carbendazim at 1000 (C1), 2000 (C2) and 3000 (C3) on nitrogen contents in root shoot tissues (A), phosphorous contents in root and shoot tissues (B), seed attributes, number of seeds and grain yield (C), grain protein (D) and symbiotic attribute; number of nodules and dry biomass of nodules and (E), leghaemoglobin content (F) in P. sativum plants at different growth stages. Vertical bars and scatter lines represent the mean ± SD (n = 3). ANOVA significant at p ≤ 0.05. Error bars represent S.D. While, df represents the degree of freedom. | |
The impact of varying concentrations of kitazin, hexaconazole and carbendazim on the quantity and quality of grains of P. sativum plants was, however, inconsistent. The seed yield (SY) and grain protein (GP) of P. sativum estimated at harvest decreased gradually with the dose-dependent increase in fungicides (Fig. 3C and D). As an example, 192, 80 and 2000 μg kg−1 kitazin, hexaconazole and carbendazim significantly (p ≤ 0.05) declined the SY of P. sativum by 26%, 27% and 52%, respectively, over the untreated control. Like SY, a maximum reduction in GP was also observed at the highest test concentration of each fungicide. As an example, a maximum decline of 39% in GP was recorded when P. sativum was raised in the presence of 1000 μg kg−1 of carbendazim over the untreated control.
Two-way ANOVA significantly (p ≤ 0.05) showed the statistical effect of the untreated control and fungicides (untreated control × fungicides) on the measured parameters. The deleterious impact of the fungicides on the seed features could be attributed to the uptake and translocation of fungicides even within the edible parts of the plants. The accumulation of fungicides within grains of legumes could thus be a major human health concern. Hence, it requires attention to find ways as to how the toxicity of fungicides and their consequential deposition within grains can be avoided. Furthermore, the total P and N content inside the plant organs (roots and shoots) also declined following the fungicide application. A dependable decrease in P and N content within the shoots and roots was recorded (Fig. 3A and B). The root P, shoot P, root N and shoot N decreased by 29%, 28%, 21% and 26%, respectively, when P. sativum plants were grown at 196 μg kg−1 kitazin over the untreated control. Also, hexaconazole and carbendazim when applied at the three times dose rate reduced the root P, shoot P, root N and shoot N by 64%, 48%, 31% and 50%, respectively, over the control (Fig. 3A and B). The results obtained here indicated that each concentration of fungicides enhanced the P and N content in the shoots compared to those recorded for roots, which could possibly be due to rapid translocation of nutrients from the roots to shoots during the vegetative growth of P. sativum. Various mechanisms have been proposed to clarify the mechanism leading to the biochemical changes brought about by fungicides, which result in the change of nutritive composition. For example, the growth of plants is affected by an osmotic shock effect of systemic fungicides, which causes a release of structural protein and loss of transportability in the cells. Also, the possibility of the inhibition of protein synthesis and a decrease in carbohydrate content cannot be ruled out. Earlier studies suggested that toxicants produced by pesticides, including fungicides application, retarded the protein and carbohydrate synthesis by inducing an alteration in cytochrome oxidase activity, blocking alternative respiratory pathways and an accumulation of succinate.
3.2.3. Proline and malondialdehyde (MDA) content. Proline (water-soluble amino acid) produced by plants under harsh environments is considered an important biomolecule that protects membranes from the destructive effects of stressors by acting as a scavenger. Additionally, it also acts as a compatible osmolyte and hence can help to store C and N. Besides these, proline can be a ROS scavenger, function as molecular chaperone stabilizing structure of proteins and help to maintain cytosolic pH and to balance the redox status of cells. Hence, the increase in free cellular proteins secreted under variable environmental stresses has been reported to play multifarious defensive roles in the majority of plant species.43 Considering the importance of proline in plants' defence under stressed environments and the lack of information available on proline extracted from this plant, the proline secreting ability of pea grown under fungicide stress was tested. Here, we observed a substantial accumulation of proline in different organs of P. sativum plants grown under different fungicide regimes. The results of this study represent a direct and positive relationship between the rates of fungicides added to soil and the proline level synthesized by different organs of pea. Expectedly, the concentration of proline in roots, shoots and leaves measured at 90 DAS and those in grains detected at 130 DAS increased progressively with enhancing the concentrations of each fungicide (Fig. 4). A maximum of 44.8 (62% increase), 61.4 (67% increase), 49.7 (65% increase) and 27 mg g−1 fresh weight (53% increase) of proline was found in roots, shoots, leaves and grains, respectively, of plants grown at 3000 μg kg−1 of carbendazim. Similarly, hexaconazole at 120 μg kg−1 and 296 μg kg−1 kitazin increased the proline content in shoots maximally by 56% and 52%, while it was 76 and 73%, in roots, respectively, over the untreated control (Fig. 4D and E). Organ wise, the proline concentration was recorded more in shoots than in the other organs of pea plants. The increase in the level of proline is interesting because this is likely to protect the pea plants from the harmful effects of fungicides and oxidative stress and its increased level suggests that stressor molecules, for example fungicides here, probably have an inducible effect on proline synthesis.
 |
| Fig. 4 Fungicides (μg kg−1): kitazin at 96 (K1); 192 (K2) and 288 (K3); hexaconazole at 40 (H1) 80 (H2) and 120 (H3) and carbendazim at 1000 (C1), 2000 (C2) and 3000 (C3) affects the antioxidant enzymes and proline content in P. sativum plants; (A) guaiacol peroxidase (GPX) activity, (B) ascorbate peroxidase (APX) activity, (C) peroxidase (POD) activity. (D) and (E) represent the accumulation of proline in various plant organs (roots, shoots, leaves and grains). Vertical bars and scatter plot represent the mean ± SD (n = 3). ANOVA significant at p ≤ 0.05. Error bars represent S.D. While, df represents the degree of freedom. | |
In a similar study, a concentration-dependent increase in proline content in various organs of Cicer arietinum plants grown under a herbicide-stressed environment has recently been reported.44
Since, lipid peroxidation is believed to act as a biomarker for oxidative stress, the assessment of MDA content in plants is inevitable. Malondialdehyde (MDA), synthesized in plants, is a decomposition product of the polyunsaturated fatty acid component of membrane lipid. It has a potential role in damaging the cell membrane of plants.45 As a substitute of ROS, the other products of stress include thiobarbituric acid reactive species (TBARS), which are measured as malondialdehyde (MDA). Therefore, the degradation of cellular membrane and other lipid structures that yield by-products causing DNA damage was assayed by measuring the level of MDA in pea plants. Therefore, the MDA content in foliage was assayed and found to significantly (p ≤ 0.05) increase with increasing concentrations of fungicides. Carbendazim, among the fungicides tested, produced the maximum amount (13.1 μmol g−1 fw) of MDA at 3000 μg kg−1, which was calculated as an increase of 84% over the control. Similarly, an enhancement of 60% and 69% in MDA content was observed at 288 μg kg−1 kitazin and 120 μg kg−1 hexaconazole, respectively, as compared to the untreated control. The increased level of MDA content with increasing concentrations of each selected fungicides confirmed the increased lipid peroxidation within the membranes of cell organelles due to the severity of stressor molecules, which may be associated with the decreased metabolic activity of essential enzymes and proteins that are essential for the growth and development of plants.
In a similar investigation, Li et al.46 reported a substantial increase in MDA content of two cultivars of kidney bean under cadmium stress.
3.2.4. Leaf electrolyte leakage, accumulation of H2O2 and antioxidant defence enzymes. Plants quite often generate ROS under stressful environments.47 H2O2 accumulation and electrolyte leakage are considered excellent biomarkers of stress-induced tissue damage.48 H2O2 is a component of oxidative breakdown and is itself a toxic ROS that demonstrates damaging effects. It has been reported to interrupt metabolism, causes a loss of cellular integrity and oxidative damage and decreases cell membrane stability.49,50 Changes in H2O2 levels in tissues can serve as a good indicator of the membrane structural integrity of plants exposed to stressed environments. The present study demonstrated that fungicide treatments badly affected the stress biomarkers (H2O2 content and electrolyte leakage) and significantly (p ≤ 0.05) enhanced their concentration with increasing fungicide dosage rates. The varying concentrations of kitazin, hexaconazole and carbendazim exhibited a significant and noticeable increase in leaf electrolyte leakage and maximum increases of 40%, 48% and 54% were recorded at 3× doses of each fungicide, respectively, over the untreated control (Fig. 5A). In agreement to this study, an increase in leaf electrolyte leakage of glyphosate-treated P. sativum plants has previously been described. Likewise, there was a substantial/significant (p ≤ 0.05) accumulation of H2O2 in root the tissues of P. sativum grown in soils treated with fungicides. For instance, a maximum increase of 42%, 31% and 26% in H2O2 concentration in plants was found at 3000, 120 and 288 μg kg−1 of carbendazim, hexaconazole and kitazin, respectively, over the untreated control. A similar increase in H2O2 accumulation in pesticide-treated seedlings of S. lycopersicum has recently been recorded.51
 |
| Fig. 5 Effect of different doses of fungicides (μg kg−1): kitazin at 96 (K1); 192 (K2) and 288 (K3); hexaconazole at 40 (H1) 80 (H2) and 120 (H3) and carbendazim at 1000 (C1), 2000 (C2) and 3000 (C3) on stress marker of P. sativum; (A) electrolyte leakage (%), (B) H2O2 accumulation and (C) MDA content. Each value is a mean of three replicates, where each replicate constituted three plants per pot. Mean values (±) followed by different letters are significantly different at p ≤ 0.05 according to DMRT test. Vertical bars represent the mean ± SD (n = 3). ANOVA significant at p ≤ 0.05. Error bars represent S.D. While, df represents the degree of freedom. | |
Reactive oxygen species (ROS) are yet another highly reactive molecule that can damage biomolecules, such as lipids, proteins and nucleic acids.52 Reactive oxygen species is a combined term that comprises both oxygen radicals, such as superoxide (O2˙−), hydroxyl (OH˙), peroxyl (RO2˙) and hydroperoxyl (HO2˙) radicals and certain nonradical oxidizing agents, such as hydrogen peroxide (H2O2), hypochlorous acid (HOCl) and ozone (O3), that can be converted easily to into radicals. Once lipid radicals are produced, they combine with oxygen dissolved in the membranes and form peroxyl radicals, which can attack membrane proteins and adjacent polyunsaturated fatty acid, propagating membrane lipid peroxidation. Mechanistically, fungicides at higher concentrations generate the maximum amounts of ROS, which in effect cause oxidative damage at the cellular level.
To minimize the effect of oxidative stress, plants have evolved efficient antioxidant systems to protect them from shocking effects. SOD, CAT, APX, GPX and GR are some of the most common ROS scavenging enzymes secreted by various cell organelles, such as chloroplast and mitochondria.53 For instance, SOD and CAT together convert [O–O]2− and H2O2 to O2 and H2O and additionally reduce ·OH radical, while POD acts as a scavenger of ROS. In our study, the level of antioxidant enzymes (POD, APX and GPX) extracted from P. sativum foliage improved markedly with increasing the concentration of fungicides. The POD, APX and GPX activity were recorded to be at a maximum in 3000 μg kg−1 carbendazim-treated plants, which were increased by 28%, 29% and 37%, respectively, compared to the untreated control (Fig. 5B). Hexaconazole at 120 μg kg−1 boosted the POD, APX and GPX activity by 20%, 16% and 24%, while kitazin at 300 μg kg−1 enhanced the activities of these enzymes by 16%, 20% and 28%, respectively, over the control. Conclusively, the data obtained in the present study confirmed a positive co-relationship (r = 0.79) between the added fungicide doses and activities of the antioxidant defence enzymes. A similar generation of ROS and increased antioxidant enzyme activity under consistently enhancing abiotic stress conditions (such as pesticides and heavy metals) have been reported in different organs of many plants,54 including those of legumes.55
3.3. Morphological distortion in root tips, cytotoxicity and cellular damage assessment
Since the selected fungicides confirmed the toxicity and suppressed the growth of P. sativum, it was decided to further assess the damaging/destructive effect of such fungicides on the morphology of water/nutrient absorbing organ (root tips) of pea plants employing SEM while growing the plants under kitazin-, hexaconazole- and carbendazim-treated soils (Fig. 6). The inhibitory effect of these fungicides to roots was more distinct at the radical regions of growing plants. Application of the fungicides showed a significant aberration, fissures, crumble, fracture and spikes on the root surface (Fig. 6B–D) compared to a clear, smooth and intact untreated root surface (Fig. 6A). The destruction of the root surface further validated the inhibitory potential of fungicides, which in turn might have impaired/impeded the uptake of water and other essential macro- and micro-nutrients from soils causing improper/altered root growth. Similarly, Shahid et al.41 observed a significant damage in the morphological structure of roots of greengram plants grown under herbicide-stressed conditions. These anti-fungal compounds, for example, carbendazim, benzimidazoles benomyl and phenyl carbamate, unambiguously precisely bind to structural protein and their subunits (tubulin) and restrict the establishment of microtubule formation, which is considered as one of the principal components in variable cellular processes and cell shape maintenance.
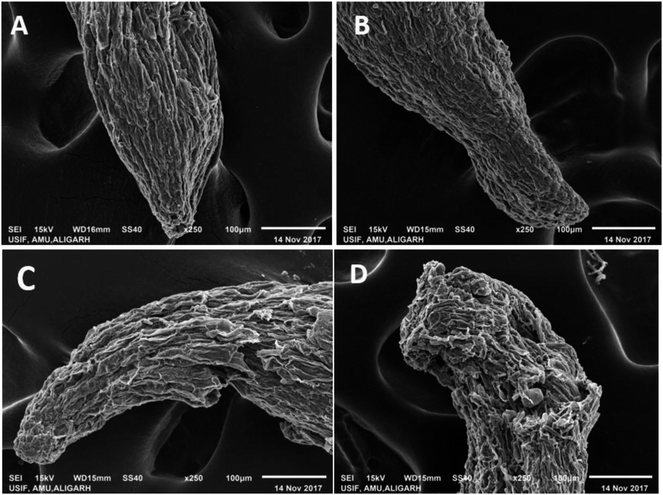 |
| Fig. 6 SEM micrograph of P. sativum roots: panel (A) represents the root tip of untreated control, whereas, panels (B), (C) and (D) show damaged/fractured and fissures in the root tips of kitazin, hexaconazole and carbendazim treated, respectively. | |
The cell membrane is yet another important organelle that is selectively permeable and that controls the transport of ions and molecules and allows cells to interact with the surrounding environment. To better understand the impact of fungicides on the membrane, the integrity of the membranes is visualized. To this end, CLSM has been found to be the most delicate and reliable technique to obtain a 3D image of the basic architecture of the tissues.56 So, acridine orange (AO) and propidium iodide (PI) were used in this study to discriminate between viable/healthy and stable cells and cells with a ruptured/broken membrane of P. sativum root tissues exposed to variable doses of kitazin, hexaconazole and carbendazim. It is well known that PI is a small hydrophilic molecule, which is impermeable to the membrane of viable cells and therefore, is rejected by living/viable cells but penetrates the membrane of dead cells with damaged membranes, whereby it interpolates the ds DNA and emits red fluorescence. Remarkably, a dose-dependent increase in the number of dead cells was observed in roots treated with various concentrations of kitazin, hexaconazole and carbendazim as compared with the untreated control (Fig. 7.1). The cellular damage caused due to the fungicides was clearly visible in the fluorescent micrograph and the intensity of red fluorescence emitted by PI increased continuously with increasing concentrations of fungicides. In order to quantify the cellular damage induced by fungicides, the red fluorescence of PI in membrane-altered cells was determined (Fig. 7.1). Compared to the fluorescence of PI in control roots, fungicides at 1×, 2× and 3× dosage rates showed the following increases: 1.6-, 3.86-, 5.46-fold for kitazin; 1.72-, 5.2-, 9.3-fold for hexaconazole; and 1.2-, 6.5-, 12.12-fold for carbendazim. These results unequivocally suggested that the dose-dependent toxic action of fungicides was facilitated by the ROS-mediated destruction of membrane lipids, which consequently increased the fluorescence of PI bound to the DNA of cells with damaged cell membranes. The increasing cellular damage signified the enhanced oxidative stress in P. sativum plants under the influence of fungicides. These results clearly suggested that fungicide exposure was arbitrated by ROS-mediated damage to membrane lipids, which therefore increased the fluorescence of DNA-bound propidium iodide in membrane-compromised cells. In accordance with this finding, Grossmann et al.57 recently reported the imaging of living plant cells using CLSM. The results obtained in this study henceforth confirmed the fact that the most decisive target of fungicides was the cell membrane as also reported for other stressor molecules by Ghezzi et al.58
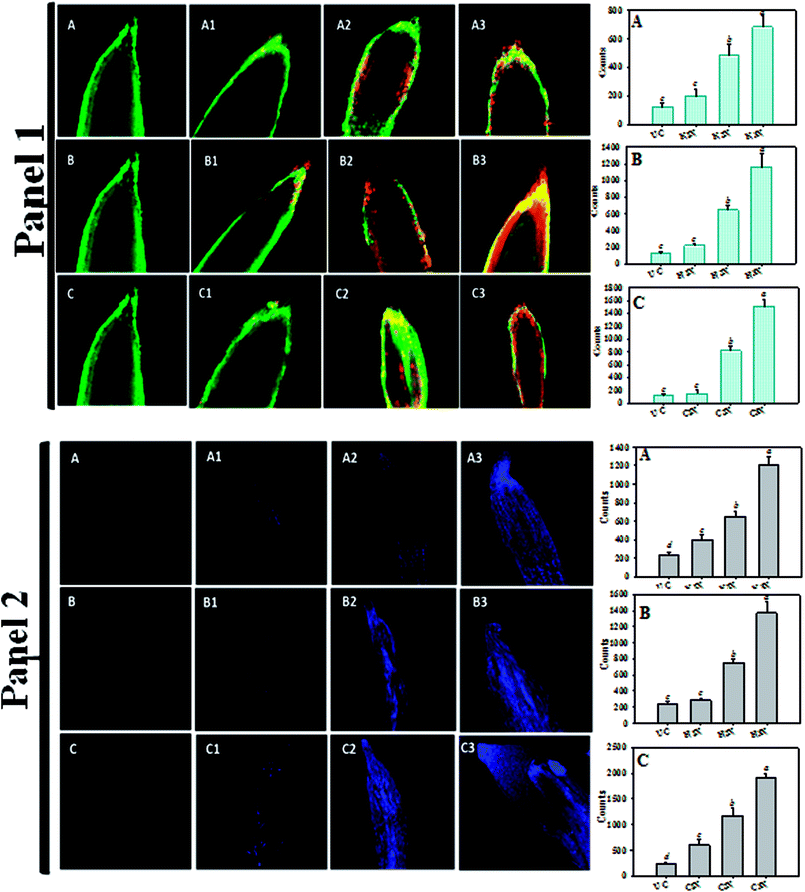 |
| Fig. 7 (1) The Z-stack image analysis of propidium iodide (PI) and acridine orange (AO) stained roots of P. sativum by using CLSM representing the oxidative stress and cellular damage induced by fungicides. Images reveal an increase in red/orange fluorescence as the concentrations of fungicides increases. Panel (2) depicts the cytotoxicity (Evans blue dye exclusion assay) assay; figures shows the uptake of Evans blue dye by root cells; (A–C) untreated control root showing no blue colour, while roots treated with various doses of kitazin (A1–A3), hexaconazole (B1–B3) and carbendazim (C1–C3) show the uptake of dye. As the conc. of fungicides increased, the intensity of blue fluorescence increased. Vertical bars represent the quantification of the emission of fluorescence using software equipped with CLSM after treating the plant roots with fungicides. Five roots per treatment (n = 5) were taken for analysis and the intensity counts were manually averaged and presented as the mean ± S.D. as a function of fungicide concentration. Mean values (±) followed by different letters are significantly different at p ≤ 0.05 according to DMRT test. ANOVA significant at p ≤ 0.05. Error bars represent S.D. | |
The loss/damage of plasma membrane in the root tissues of P. sativum under varying concentrations of fungicides was evident when the roots were stained with Evans blue dye. As the dose of fungicides increased, the uptake of dye by the root tissues increased 3–4-fold, resulting in losses in the integrity of the plasma membrane. As an example, P. sativum roots treated with 3× concentrations each of kitazin, hexaconazole and carbendazim demonstrated an increased intensity of fluorescence of blue colour compared to untreated roots. On the other hand, untreated roots did not take up the dye and hence, the root periphery remained smooth, signifying an improved and functional plasma membrane integrity (Fig. 7.2). In quantitative determination, a sharp increase in Evans blue fluorescence was recorded in the following order: 1.69-, 2.75-, 5.08-fold for kitazin; 1.19-, 3.16-, 5.77-fold for hexaconazole; and 2.36-, 5.57-, 9.11-fold for carbendazim. This is a clear reflection of the penetration of Evans blue into non-viable cells. These results suggest that, upon exposure to fungicides, root cells lost their capability of repair and therefore were stained blue. These findings further affirmed that selected fungicides while acting on roots may rupture the cells, leading eventually to the cell death. There are several mechanisms that have been proposed by meticulous scientists about how the higher rates of fungicides retard the physiological and biochemical processes of the plants and which could provide further insights into growth retardation and cyto-killing as occurred in the present study. For illustration, the presence of fungicide residues (solutes) in soil distresses the buffering capacity of soil and the thermodynamic activity of water along with essential nutrients in the surrounding soil. The presence of fungicides residues and other pollutants in soil tends to decrease the uptake of water along with nutrients as pesticides residue when contacting soil particles gets attached, thus affecting the nutrient uptake from the soil to the root. The presence of pesticides residues might affect the micronutrient transport system and plasma membrane of the root cells, which in turn disturbs the uptake of cations Fig. 8.
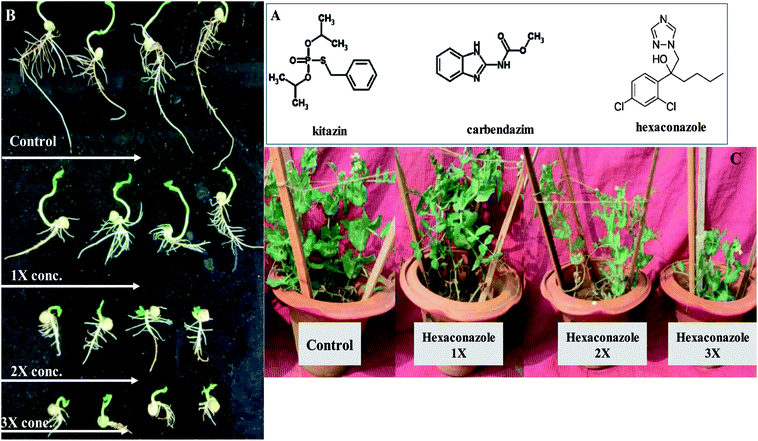 |
| Fig. 8 This figure depicts the chemical structure of fungicides (A), concentration-dependent decrease in radicle and plumule length of pea seeds germinated on 0.7% soft agar plates supplemented with 0 (control), 1×, 2× and 3× concentrations of kitazin, carbendazim and hexaconazole (B) and pea plants grown in sandy clay loam soils untreated and treated with varying (1×, 2× and 3×) doses of hexaconazole (C). | |
4. Conclusion
The fungicides used in this study exhibited a negative impact on various biological characteristics of P. sativum plants. Stress induced by fungicides caused a series of systematic changes in physiology, anatomy, cytotoxicity, cellular damage and a distortion in the root morphology of plants, as revealed under SEM and CLSM. Furthermore, the formation of photosynthetic pigments, nutrient uptake and grain production was severely hindered under fungicide stress. Also, stomatal behaviour and morpho-anatomical alterations in pea foliage were clearly visible under SEM/CLSM. The accumulation of H2O2, leaf electrolyte leakage, generation of proline, synthesis of MDA and production of antioxidant defence enzymes on the contrary was indeed encouraging features and were enhanced gradually with increasing the dosages of the fungicides. The heightened concentrations of such active biomolecules can likely assist peas to overcome fungicidal toxicity while growing under fungicides-polluted soils. Conclusively, even though this well-organized and massive study provided enough quality information to better understand the finer details of the mechanistic basis of fungicide toxicity towards legumes in general and peas in particular, extensive and inappropriate fungicide application in pulse production practices without an accurate understanding of the fungicides–legume interactions must be avoided so that the quality of pulses will be protected from the toxicity of fungicides.
Authors contributions
Mohd. Saghir Khan (MSK) conceived and designed the experiments. Mohammad Shahid (MS) performed the experiments and analyzed the data statistically. Almas Zaidi (AZ) and Bilal Ahmad (BA) edited the manuscript. MS and MSK prepared the manuscript and approved the final draft.
Funding
The author (Mohammad Shahid) would like to acknowledge the financial support received in the form of UGC Non-NET fellowship awarded by University Grants Commission, Government of India, New Delhi, India.
Conflicts of interest
The authors declare that they have no conflict of interest.
Acknowledgements
The authors would like to thank University Sophisticated Instrument facility (USIF) for providing SEM and CLSM facilities.
References
- N. B. Patil, S. Zacharia and M. Kumari, Eco-friendly management of powdery mildew of garden pea (Pisum sativum L.), Int. J. Curr. Microbiol. Appl. Sci., 2017, 6, 684–689 CrossRef CAS.
- M. Iriti and E. M. Varoni, Pulses, healthy, and sustainable food sources for feeding the planet, Int. J. Mol. Sci., 2017, 18, 255 CrossRef PubMed.
- M. N. H. Zilani, T. Sultana, S. A. Rahman, M. Anisuzzman, M. A. Islam, J. A. Shilpi and M. G. Hossain, Chemical composition and pharmacological activities of Pisum sativum, BMC Complementary Altern. Med., 2017, 17(1), 171 CrossRef PubMed.
- L. Kumar, S. C. Verma and P. L. Sharma, Studies on effect of essential oils on quality characters of pea seeds (Pisum sativum L.) damaged by Callosobruchus chinensis L. (Coleoptera: Bruchidae), J. Entomol. Zool. Stud., 2017, 5, 562–565 Search PubMed.
- J. Katan, Diseases caused by soil borne: Biology, Management and challenges, J. Plant Pathol., 2017, 99, 2 Search PubMed.
- J. S. Kang, H. Singh, G. Singh, H. Kang, V. P. Kalra and J. Kaur, Abiotic stress and its amelioration in cereals and pulses: A Review, Int. J. Curr. Microbiol. Appl. Sci., 2017, 6, 1019–1045 CAS.
- R. L. Sharma, M. Tushar, B. Rakesh and V. K. Swarnkar, Field evaluation of different new fungicides against rust disease of field pea (Pisum sativum L.), Int. J. Plant Protec, 2017, 10, 186–192 CrossRef.
- M. Shahid, A. Zaidi, M. S. Khan, A. Rizvi, S. Saif and B. Ahmed, Recent advances in management strategies of vegetable diseases, in Microbial Strategies for Vegetable Production, Springer, Cham, 2017, pp. 197–226 Search PubMed.
- T. Cui, Y. Zhang, W. Han, J. Li, X. Sun, J. Shen and L. Wang, Advanced treatment of triazole fungicides discharged water in pilot scale by integrated system: Enhanced electrochemical oxidation, up flow biological aerated filter and electrodialysis, Chem. Eng. J., 2017, 315, 335–344 CrossRef CAS.
- B. Anuradha, A. Rekhapadmini and V. Rangaswamy, Influence of tebuconazole and copper hydroxide on phosphatase and urease activities in red sandy loam and black clay soils, 3 Biotech, 2016, 6, 78 CrossRef CAS PubMed.
- T. Uthman, A. Awosanya, A. Ayanwale and T. Ayinde, The effect of fungicides on the nodulation of cowpea (vigna unguiculata L.) in a tropical rainforest, Environ. J. Sus. Dev., 2017, 7, 56–61 Search PubMed.
- V. Mishra, A. A. Lal and S. Simon, Efficacy of botanicals and bio-agents against powdery mildew disease of garden pea (Pisum sativum L.), J. Pharm. Phyto., 2017, 6, 1125–1126 Search PubMed.
- C. Ju, J. Xu, X. Wu, F. Dong, X. Liu, C. Tian and Y. Zheng, Effects of hexaconazole application on soil microbe's community and nitrogen transformations in paddy soils, Sci. Total Environ., 2017, 609, 655–663 CrossRef CAS PubMed.
- Y. D. Kengar and B. J. Patil, Ureide content of guar under influence of hexaconazole and triazophos, J. Fert. Pest., 2017, 8, 176 Search PubMed.
- B. Mourad, B. B. Eddine and B. Mokhtar, The response of antioxidant defense system of a legume green bean Phaseolus vulgaris cv. Dje exposed to a xenobiotic hexaconazole, Int. J. Adv. Eng. Manag., 2017, 2, 270–2780 Search PubMed.
- Y. M. Xiao, L. Esser, F. Zhou, C. Li, Y. H. Zhou, C. A. Qin and D. Xia, Studies on inhibition of respiratory cytochrome bc1 complex by the fungicide pyrimorph suggest a novel inhibitory mechanism, PloS one, 2014, 9, 93765 CrossRef PubMed.
- S. Ozkirimli, F. Kazan and Y. Tunali, Synthesis, antibacterial and antifungal activities of 3-(1, 2, 4-triazol-3-yl)-4-thiazolidinones, J. Enz. Inhib. Med. Chem, 2009, 24, 447–452 CrossRef CAS PubMed.
- B. Ahmed, A. Hashmi, M. S. Khan and J. Musarrat, ROS mediated destruction of cell membrane, growth and biofilms of human bacterial pathogens by stable metallic Ag-NPs functionalized
from bell pepper extract and quercetin, Adv. Pow. Technol., 2018a, 29, 1601–1616 Search PubMed.
- A. A. Abdul-Baki and J. D. Anderson, Vigor determination in soybean seed by multiple criteria, Crop. Sci., 1973, 13, 630–633 CrossRef.
- D. I. Arnon, Copper enzymes in isolated chloroplasts. Polyphenol oxidase in Beta vulgaris, Plant physiol, 1949, 24, 1 CrossRef CAS PubMed.
- J. T. O. Krik and R. L. Allen, Dependence of chloroplast pigment synthesis on protein synthesis: effect of actidione, Biochem. Biophy. Res. Comm., 1965, 21(6), 523–530 CrossRef.
- S. Sadasivam and A. Manickam, Biochemical Methods, New Age International, 1992 Search PubMed.
- M. L. Jackson, Soil chemical analysis, Prentice-Hall of India, New Delhi, 1976 Search PubMed.
- R. C. Lindner, Rapid analytical methods for some of the more common inorganic constituents of plant tissues, Plant physiol, 1944, 19, 76 CrossRef CAS PubMed.
- O. H. Lowry, N. J. Rosebrough and A. L. Farr, Protein measurement with the Folin-phenol reagent, J. Boil. Chem., 1951, 193, 265–275 CAS.
- L. S. Bates, R. P. Waldren and I. D. Teare, Rapid determination of free proline for water stress studies, Pl. Soil., 1973, 39, 205–208 CrossRef CAS.
- A. M. Ismail and A. E. Hall, Reproductive-stage heat tolerance, leaf membrane thermostability and plant morphology in cowpea, Crop Sci, 1999, 39, 1762–1768 CrossRef.
- B. D. Patterson, E. A. Mac Rae and I. B. Ferguson, Estimation of hydrogen peroxide in plant extracts using titanium (IV), Ana. Biol., 1984, 139, 487–492 CAS.
- B. Ahmed, M. S. Khan and J. Musarrat, Toxicity assessment of metal oxide nano-pollutants on tomato (Solanum lycopersicon): A study on growth dynamics and plant cell death, Environ. Pol., 2018b, 240, 802–816 Search PubMed.
- J. Zhang and M. B. Kirkham, Antioxidant responses to drought in sunflower and sorghum seedlings, New Phytol., 1996, 132, 361–373 CrossRef CAS PubMed.
- S. S. Leonard, G. K. Harris and X. Shi, Metal-induced oxidative stress and signal transduction, Free Rad. Bio. Med., 2004, 37, 1921–1942 CrossRef CAS PubMed.
- R. Hammerschmidt, E. M. Nuckles and J. Kuć, J, Association of enhanced peroxidase activity with induced systemic resistance of cucumber to Colletotrichum lagenarium, Physio. Plant Path., 1982, 20, 977–1082 Search PubMed.
- B. Ahmed, S. Dwivedi, M. Z. Abdin, A. Azam, M. Al-Shaeri, M. S. Khan, Q. Saquib, A. A. Al-Khedhairy and J. Musarrat, Mitochondrial and chromosomal damage induced by oxidative stress in Zn2+ ions, ZnO-bulk and ZnO-NPs treated Allium cepa roots, Sci. Rep., 2017, 7, 40685 CrossRef CAS PubMed.
- M. Shahid and M. S. Khan, Fungicide tolerant Bradyrhizobium japonicum mitigate toxicity and enhance greengram production under hexaconazole stress, J. Environ. Sci., 2018 DOI:10.1016/j.jes.2018.07.007.
- M. P. Gomes, F. V. da Silva Cruz, E. M. Bicalho, F. V. Borges, M. B. Fonseca, P. Juneau and Q. S. Garcia, Effects of glyphosate acid and the glyphosate-commercial formulation (Roundup) on Dimorphandra wilsonii seed germination: interference of seed respiratory metabolism, Environ. Pol., 2017, 220, 452–459 CrossRef CAS PubMed.
- T. J. Rose, L. Van Zwieten, A. Claassens, C. Scanlan and M. T. Rose, Phytotoxicity of soil borne glyphosate residues is influenced by the method of phosphorus fertiliser application, Plant Soil, 2018, 422, 455–465 CrossRef CAS.
- L. Guidi, M. Tattini and M. Landi, How does chloroplast protect chlorophyll against excessive light?, in Chlorophyll, In Tech, 2017 Search PubMed.
- B. Sankar, K. Karthishwaran and R. Somasundaram, Photosynthetic pigment content alterations in Arachis hypogeae L. in relation to varied irrigation levels with growth hormone and triazoles, J. Eco. Biotechnol., 2017, 5, 7 Search PubMed.
- T. Parween, S. Jan and T. Fatma, Evaluation of oxidative stress in Vigna radiata L. in response to chlorpyrifos, Int. J. Environ. Sci. Tech., 2012, 9(4), 605–612 CrossRef CAS.
- V. B. Junqueira, A. C. Costa, T. Boff, C. Müller, M. A. C. Mendonça and P. F. Batista, Pollen viability, physiology, and production of maize plants exposed to pyraclostrobin+ epoxiconazole, Pest. Bio. Phys., 2017, 137, 42–48 CrossRef CAS PubMed.
- M. Shahid, B. Ahmed and M. S. Khan, Evaluation of microbiological management strategy of herbicide toxicity to greengram plants, Biocat. Agric. Biotech., 2018, 1(14), 96–108 Search PubMed.
- J. E. Fox, J. Gulledge, E. Engelhaupt, M. E Burow and J. A. McLachlan, Pesticides reduce symbiotic efficiency of nitrogen-fixing rhizobia and host plants, Proc. Nat. Acad. Sci., 2007, 104(24), 10282–10287 CrossRef CAS PubMed.
- K. Nahar, M. Hasanuzzaman, T. Suzuki and M. Fujita, Polyamines-induced aluminium tolerance in mung bean: A study on antioxidant defence and methylglyoxal detoxification systems, Ecotoxicol, 2017, 26, 58–73 CrossRef CAS PubMed.
- M. Shahid and M. S. Khan, Glyphosate induced toxicity to chickpea plants and stress alleviation by herbicide tolerant phosphate solubilizing Burkholderia cepacia PSBB1 carrying multifarious plant growth promoting activities, 3 Biotech, 2018b, 8, 131 Search PubMed.
- R. Abedini, F. Ghane Golmohammadi, R. Pishkam Rad, E. Pourabed, A. Jafarnezhad, Z. S. Shobbar and M. Shahbazi, Plant dehydrins: Shedding light on structure and expression patterns of dehydrin gene family in barley, J. Plant Res., 2017, 130, 747–763 CrossRef CAS PubMed.
- D. Li, G. Chen, Q. Lu, Y. Li, J. Wang and H. Li, Responses of two kidney bean (Phaseolus vulgaris) cultivars to the combined stress of sulphur deficiency and cadmium toxicity, Bio. Metals., 2017, 1, 12 Search PubMed.
- S. A. Kim, Y. M. Lee, J. Y. Choi, D. R. Jacobs and D. H. Lee, Evolutionarily adapted hormesis-inducing stressors can be a practical solution to mitigate harmful effects of chronic exposure to low dose chemical mixtures, Environ. Pol., 2018, 233, 725–734 CrossRef CAS PubMed.
- M. Arasimowicz-Jelonek, J. Floryszak-Wieczorek and J. Kubiś, Involvement of nitric oxide in water stress-induced responses of cucumber roots, Plant Science, 2009, 1(177), 682–690 CrossRef.
- Y. Imahori, J. H. Bai and E. Baldwin, Antioxidative responses of ripe tomato fruit to postharvest chilling and heating treatments, Sci. Hort., 2016, 198, 398–406 CrossRef CAS.
- B. Ahmed, M. Shahid, M. S. Khan and J. Musarrat, Chromosomal aberrations, cell suppression and oxidative stress generation induced by metal oxide nanoparticles (MONPs) in onion (Allium cepa) bulb, Metallomics, 2018, 10(9), 1315–1327 RSC.
- S. K. Shakir, S. Irfan, B. Akhtar, S. Rehman, M. K. Daud, N. Taimur and A. Azizullah, Pesticide-induced oxidative stress and antioxidant responses in tomato (Solanum lycopersicum) seedlings, Ecotoxicol, 2018, 1–17 Search PubMed.
- S. Yilmaz, E. Kaya and M. A. Kisacam, The effect on oxidative stress of aflatoxin and protective effect of lycopene on aflatoxin damage, in Aflatoxin-Control, Analysis, Detection and Health Risks, In Tech, 2017 Search PubMed.
- S. Tiwari, S. Tiwari, M. Singh, A. Singh and S. M. Prasad, Generation mechanisms of reactive oxygen species in the plant cell: An Overview, Revisiting the role of reactive oxygen species (ROS) in plants: ROS Boon or Bane for Plants, 2017, pp. 1–22 Search PubMed.
- M. I. Dar, M. I. Naikoo, F. A. Khan, F. Rehman, I. D. Green, F. Naushin, and A. A. Ansari, An introduction to reactive oxygen species metabolism under changing climate in plants, in: Reactive oxygen species and antioxidant systems in plants: Role and Regulation under Abiotic Stress, Springer, 2107, pp. 25–52 Search PubMed.
- K. Kodin, Effect of drought stress on the oxidative enzyme activity in Medicago sativa, Doctoral dissertation, University of Nebraska at Kearney, 2017 Search PubMed.
- A. Le Marois and K. Suhling, Quantitative live cell FLIM imaging in three dimensions, in Multi-Parametric Live Cell Microscopy of 3D Tissue
Models, Springer, Cham, 2017, pp. 31–48 Search PubMed.
- G. Grossmann, M. Krebs, A. Maizel, Y. Stahl, J. E. Vermeer and T. Ott, Green light for quantitative live-cell imaging in plants, J. Cell Sci., 2018, 209270 CrossRef PubMed.
- P. Ghezzi, L. Floridi, D. Boraschi, A. Cuadrado, G. Manda, S. Levic, F. D'Acquisto, A. Hamilton, T. J. Athersuch and L. Selley, Oxidative stress and inflammation induced by environmental and psychological stressors: A biomarker perspective, Anti. Redox Sig., 2018, 28, 852–872 CrossRef CAS PubMed.
|
This journal is © The Royal Society of Chemistry 2018 |
Click here to see how this site uses Cookies. View our privacy policy here.