DOI:
10.1039/C8RA03229G
(Paper)
RSC Adv., 2018,
8, 21184-21190
Engineering substrate promiscuity in 2,4-dichlorophenol hydroxylase by in silico design†
Received
15th April 2018
, Accepted 4th June 2018
First published on 8th June 2018
Abstract
2,4-Dichlorophenol hydroxylase (2,4-DCP hydroxylase) is a key enzyme in the degradation of 2,4-dichlorophenoxyacetic acid in the hydroxylation step in many bacteria. Our previous study demonstrated that a 2,4-DCP hydroxylase (TfdB-JLU) exhibits broad substrate specificity for chlorophenols (CPs) and their homologues. In this study, TfdB-JLU has been engineered by rational design to further broaden its substrate scope towards CPs. We dissect the architectures of enzymes from oxidoreductase families to discover their underlying structural sources of substrate promiscuity. A homology model of TfdB-JLU has been built and docking experiments of this homology model with its natural substrate 2,4-DCP reveal that the phenyl rings of 2,4-DCP form strong interactions with residues His47, Ile48, Trp222, Pro316, and Phe424. These residues are found to be important for substrate binding in the active site. Then, the site-directed mutagenesis strategy has been applied for redesigning substrate promiscuity in TfdB-JLU. The TfdB-JLU-P316Q variant obtained shows a significant enhancement of activity (up to 3.4-fold) toward 10 CP congeners compared to wild-type TfdB-JLU. Interestingly, the active improvements of TfdB-JLU-P316Q toward CP congeners show significant difference, especially for active improvements of positional congeners such as 3-CP (1.1-fold) compared to 4-CP (3.0-fold), as well as 2,3-DCP (1.2-fold) compared to 2,5-DCP (3.4-fold). Structural analysis results indicate that the improvement in substrate promiscuity of the variant enzyme compared to the wild-type enzyme is possibly due to the increase of non-bonding interaction. The results suggest that exploiting enzyme–substrate promiscuity is promising, which would provide a starting point for designing and engineering novel biological catalysts for pollution removal.
Introduction
Enzymes are attractive catalysts because of their promiscuity and their ability to perform highly regio-, chemo- and stereoselective transformations. Enzymes that display promiscuous behavior can be classified into reaction conditions of promiscuity, substrate promiscuity, catalytic promiscuity and alternate-site promiscuity.1 Enzyme promiscuity is the engine of evolutionary innovation which can dramatically enhance the utility of biocatalysis in biotechnology. It has thus attracted significant attention from chemists and biochemists.2
Substrate promiscuity (also known as substrate ambiguity or broad substrate specificity) of enzyme refers to the catalysis of the same reaction with a range of substrates. Enzyme with substrate promiscuity displays several advantages since they can be used to transform substrates apart from the native substrates or chemocatalysis.3 Thus, substrate promiscuity provides useful starting points for exploring novel enzymes in engineered pathways for biodegradation of widely used chemicals pollutions.4
Numerous enzyme classes has been reported to exhibit substrate promiscuity including cytochrome P450s,5,6 kinase,7,8 phosphatases,9 acylaminoacyl peptidase,10 DNA methyltransferase,11 cyclic dipeptide prenyltransferase,12 glutathione S-transferases,13 laccases14 and lipases.15 Among these enzymes, 2,4-dichlorophenol (2,4-DCP) hydroxylase (EC 1.14.13.20) is the best candidate for developing a biocatalyst for chlorophenols (CPs) due to its high hydroxylation activities toward CPs compared to those of the reported cytochrome P-450s and laccases to the best of our knowledge.16 Our previous study demonstrates that a 2,4-DCP hydroxylase (TfdB-JLU) exhibits broad substrate specificity for CPs and their homologues.17 However, this enzyme exhibited high activities toward only limited CP congeners. Thus, there has been substantial interest in expanding the substrate scope of TfdB-JLU toward more CP congeners.
Protein engineering has emerged as a powerful method to improve or alter the properties of enzymes during the past two decades. Computer modelling, directed evolution, gene shuffling, site-directed mutagenesis, and rational design (or combinations thereof) have been successfully applied to engineer the substrate promiscuity of enzyme.18,19 For example, Wu et al. reported the evolution of Candida antarctica lipase B mutants with broad substrate scope toward α-chiral esters by directed evolution based on iterative saturation mutagenesis.20 Cassidy J engineered an alcohol dehydrogenase from the halophilic archaeon Haloferax volcanii by rational design to greatly broaden its substrate scope toward the conversion of a range of aromatic substrates.21 However, few studies have been performed to elucidate the substrate promiscuity mechanism of TfdBs to date.
This study mainly aims to discover underlying structural basis of substrate promiscuity of TfdB-JLU toward CPs and to engineer TfdB-JLU variants with higher activity toward CPs. The study is initialized by using SWISS-MODEL to do the homology model of TfdB-JLU. Molecular docking study has indicated that His47, Ile48, Trp222, Ppo316, and Phe424 might be the key amino acid for its substrate promiscuity. Then in order to improve the substrate promiscuity of the enzyme, active-site saturation test and site-directed mutagenesis strategy have been applied for redesigning substrate promiscuity of TfdB-JLU.
Materials and methods
Materials
CPs of analytical grade are purchased from J&K Scientific Ltd. (Shanghai, China). Other chemicals of analytical grade are obtained from Sigma. Recombinant Escherichia coli DH5α containing the TfdB-JLU gene for 2,4-DCP hydroxylase expression is from our lab.
Methods
Sequence alignment and homology modeling of TfdB-JLU. The amino acid sequence of TfdB-JLU is collected from uncultured bacterium in the NCBI protein database (GenBank no. ACV31372.1). The initial amino acid sequence is analyzed using SWISS-MODEL (http://swissmodel.expasy.org/) to predict and select the template structure of the target models.22–24 2-Hydroxybiphenyl 3-monooxygenase (PDB ID: 5brt) sharing highest sequence identity (41.90%) with TfdB-JLU is thus chosen as template protein for homology modeling.25 The automated sequence alignment of the 5brt and TfdB-JLU are carried out using the Molecular Evolutionary Genetics Analysis version 5 (MEGA5) program.26 The sequence alignment results are analyzed by Discovery Studio 4.5 Visualizer (DS 4.5) software (Accelrys, San Diego, CA). The structure of TfdB-JLU is generated using SWISS-MODEL server, and the output of model is a standard coordinate file in the PDB format. The quality of TfdB-JLU and 5brt models are validated by PROCHECK, Verify-3D Server (https://services.mbi.ucla.edu/SAVES/), ProSA web (https://prosa.services.came.sbg.ac.at/prosa.php). Molecular energy was minimized using the energy minimization module of DS 4.5.27
Molecular docking. Docking is frequently used to predict the binding orientation of small molecule candidates to their protein targets in order to in turn predict the affinity and activity of the small molecule. The Autodock 4.2 is used to estimate the potential substrate binding position for the enzyme as molecular docking program.28 The 3D structures of TfdB-JLU are built from homology modeling method. The variants of TfdB-JLU are edit by DS 4.5 software. The substrate structures of CP congeners are also generated by DS 4.5 software. To get a better statistics and clustering, each docking is performed twice, and the parameter value of maximum number of energy evaluations, initial population size and individual LGA executions is set to 25
000
000, 300 and 100, respectively.29,30 The predicted complexes are optimized and ranked according to a free-energy scoring function that is based on a linear regression analysis and the AMBER force field.31 The results of molecular docking are analyzed by DS 4.5 software.
Protein expression and purification. The recombinant E. coli is cultivated in LB medium containing 30 μg kanamycin per ml and 34 μg chloramphenicol per ml at 37 °C. Protein expression is cultivated at an OD600 of 0.6 and induced at 16 °C by the addition of 0.2 mM isopropyl-β-D-1-hiogalato-side (IPTG) (Fisher Scientific, Dingguo, BJ). After 15 h incubation, the cell pellets are harvested by centrifugation at 12
000 rpm and wash with 50 mM pH 7.5 sodium phosphate buffer for three times. For the preparation of crude extract, cells are suspended in 30–35 ml sodium phosphate buffer with 0.6 mM PMSF (phenylmethylsulfonyl fluoride) through ultrasonic breakage. Then, the lysate is centrifuged using a high speed freezing centrifuge (Fisher Scientific, Beckman Coulter, USA) at 15
000 rpm for 20 min at 4 °C. The supernatant is transferred onto a Hislink™ column rinsed with wash buffer (50 mM sodium phosphate buffer with 0.6 mM PMSF, 10 mM imidazole, pH 7.5). The protein supernatant is loaded onto a nickel–nitrilotriacetic agarose resin (Qiagen, Germany) equilibrated with the same buffer. After washing with 5 column volumes of the wash buffer (40 mM imidazole), the bound enzyme is eluted with the elution buffer (200 mM imidazole). Then the fractions are concentrated by ultrafiltration and then are diafiltered with 50 mM sodium phosphate buffer, pH 7.5, containing 10% (v/v) glycerol. Samples are stored at −80 °C for further analysis.
Enzyme assay and characterization. The enzyme assay is determined as described previously.32 One unit of activity is defined as the amount of enzyme required to consume 1 μmol substrate per min at 25 °C. Protein concentrations are determined by the BCA method (Novagen® BCA Protein Assay Kit) using bovine serum albumin as the standard. A difference with a p < 0.05 is considered significant. Results are shown as mean ± SEM of n indicated in each case.
Results and discussion
Sequence alignment, homology modeling of TfdB-JLU and model evaluation
In order to engineer the promiscuity of the enzyme toward the CPs by rational protein design, it is mandatory to understand the mechanism of this reaction and to have a reliable structural model of this enzyme. In this study, an in silico approach is used to obtain the three-dimensional structure of TfdB-JLU. While the TfdB has been investigated in many studies, the crystal structure of this enzyme has not been solved yet. Thus, it is essential to find a homologous enzyme, which has been characterized in detail. Templates with high protein sequence identity are obtained by SWISS-MODEL server (Table S.1†). Then we chose 2-hydroxybiphenyl-3-monooxygenase (PDB ID: 5brt_A) which has been successfully crystallized at 2.30 Å by Fishman et al. as our template due to its highest homology (41.9%) and its possession of binding site of both the substrate and FAD.25 Fig. S.1† shows the sequence alignment between TfdB-JLU and 5brt protein. Then TfdB-JLU model structure is made by MEGA5. Fig. S.2† shows the 3D structure alignment between the TfdB-JLU model and the 5brt with their root mean square deviation (RMSD) value of 1.85 Å. FAD is added during the docking study since 5brt structural information suggested that TfdB-JLU contains the FAD binding domain as shown in Fig. S.3.† NADPH, however, is not added in TfdB-JLU modeling, since NADPH binding domain requires strong positively charged environment, which is lacked in TfdB-JLU.33,34
Protein structure evaluation is then assessed by online server as described above. The stereochemical quality of the model is acceptable as shown in the Ramachandran plot obtained by PROCHECK.35 The amino acid residues in the TfdB-JLU and 5brt structure are 85.0% and 89.6% in most favored regions, 13.4% and 9.7% in additional allowed regions, 1.2% and 0.4% in generously allowed regions, and only 0.4% and 0.2% in disallowed regions, respectively (Table S.2† and Fig. 1A and D). To analyze the compatibility of an atomic model (3D) with its own amino acid sequence (1D) by assigning a structural class based on its location and environment (alpha, beta, loop, polar, nonpolar etc.) and comparing the results to good structures, the TfdB-JLU model is evaluated with Verify 3D.36 98.1% and 96.1% of residues had an averaged 3D-1D score ≥ 0.2 in the TfdB-JLU and 5brt model (Fig. 1B and E). This suggests that atomic model (3D) is compatible with its amino acid sequence (1D) and the structure is identified as stable conformation. Finally the TfdB-JLU model is analyzed using ProSA-web server. The Z-score is used to indicate overall model quality.37 The Z-score values are calculated by the highlighted black dot displayed in a plot (Fig. 1C and F). Similarity between −9.61 Z-score value of TfdB-JLU model and −10.36 Z-score of the 5brt model suggests high model quality. The above results show that the predicted structures conformed well to the stereochemistry, which indicates reasonably good quality.
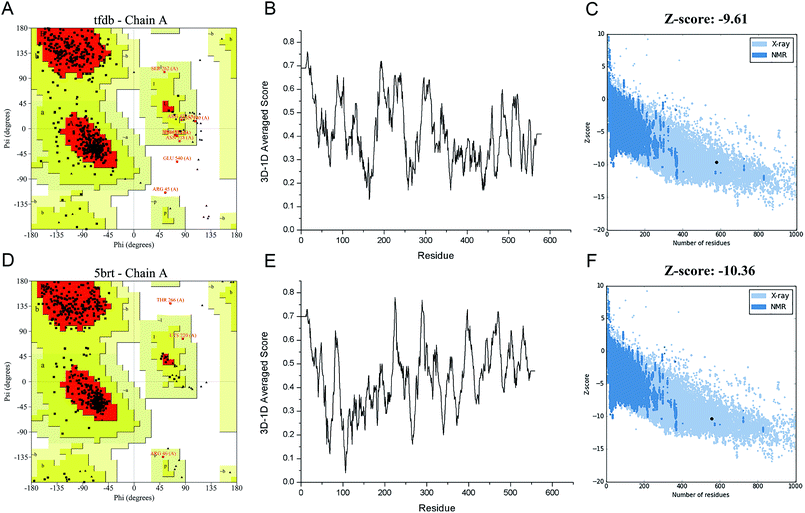 |
| Fig. 1 Structure validation of target (TfdB-JLU) model and template (5brt) model. (A and D) Ramachandran plot of TfdB-JLU and 5brt, respectively. (B and E) 3D-1D averaged score plot of TfdB-JLU and 5brt, respectively. (C and F) Z-score plot of TfdB-JLU and 5brt, respectively. | |
Key amino acid prediction affecting substrate promiscuity by molecular docking
For a better understanding of the substrate and enzyme interaction mechanism, molecular docking experiment is conducted with the modeling TfdB-JLU and 2,4-DCP. Docking results shows 2,4-DCP is located in the hydrophobic pocket of the active site, and forms hydrogen bond interaction with carbonyl oxygen atom of the isoalloxazine ring of FAD (as shown in Fig. 2A). Tables 1 and 2 gives the detail non-bond parameters of 2,4-DCP with TfdB-JLU. As Fig. 2B shows, residues His47, Ile48, Trp222, Pro316 and Phe424 of TfdB-JLU are believed to play key role in substrate binding, since they form hydropholic and halogen interactions with 2,4-DCP. These amino acid residues are thus used for further study.
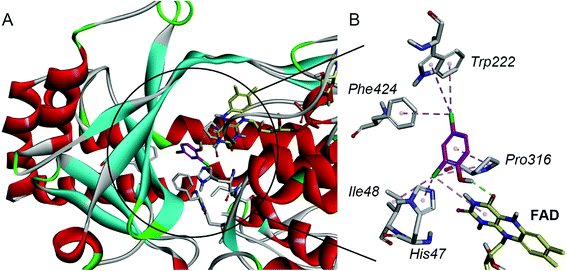 |
| Fig. 2 The interaction schematic diagram of 2,4-DCP with the active site of TfdB-JLU model. (A) 2,4-DCP (magenta) is located in the hydrophobic pocket of the active site of TfdB-JLU (gray sticks) and forms a hydrogen bond (green dashed lines) with FAD (yellow sticks). (B) The schematic diagram of the detail non-bond interaction formations of 2,4-DCP (magenta) with the active site of TfdB-JLU. It shows that 2,4-DCP forms one hydrogen bond (green dashed lines) with carbonyl oxygen atom of the FAD (yellow sticks) isoalloxazine ring, and eight non-bond interaction (pink dashed lines) with amino acid residues His47, Ile48, Trp222, Pro316 and Phe424 of TfdB-JLU (gray sticks). | |
Table 1 Hydrogen bond parameters of 2,4-DCP and TfdB-JLU_WT/TfdB-JLU_P316Q
Receptor |
Donors Atom |
Receptor Atom |
Distances (Å)a |
The length of the hydrogen bonds. |
TfdB-JLU_WT |
2,4-DCP: H |
TfdB-JLU_WT: FAD: O4 |
2.21 |
TfdB-JLU_P316Q |
TfdB-JLU_P316Q: His47: HE2 |
2,4-DCP: O |
1.81 |
|
TfdB-JLU_P316Q: FAD: H3 |
2,4-DCP: O |
2.52 |
Table 2 Hydropholic and halogen interaction parameters of 2,4-DCP with TfdB-JLU_WT
Types |
Form chemistry |
To chemistry |
Distances (Å) |
Alkyl |
2,4-DCP: Cl1 |
TfdB-JLU_WT: Ile48 |
4.14 |
Alkyl |
2,4-DCP: Cl1 |
TfdB-JLU_WT: Pro316 |
5.07 |
Pi-alkyl |
TfdB-JLU_WT: His47 |
2,4-DCP: Cl1 |
3.91 |
Pi-alkyl |
TfdB-JLU_WT: Trp222 |
2,4-DCP: Cl12 |
5.12 |
Pi-alkyl |
TfdB-JLU_WT: Trp222 |
2,4-DCP: Cl12 |
4.40 |
Pi-alkyl |
TfdB-JLU_WT: Phe424 |
2,4-DCP: Cl12 |
4.60 |
Pi-alkyl |
FAD |
2,4-DCP: Cl1 |
5.43 |
Pi-alkyl |
2,4-DCP |
TfdB-JLU_WT: Pro316 |
4.07 |
The amino acid residues His47, Ile48, Trp222, Pro316 and Phe424 of TfdB-JLU are individually mutated to alanine using DS 4.5 software. 2,4-DCP is then docked into theses protein variants, respectively. The free energy of binding of 2,4-DCP to TfdB-JLU variants increased in comparison with that of 2,4-DCP to TfdB-JLU (Table 3). The variants exhibiting the greatest increase in free energy of binding with substrate are TfdB-JLU_H47A (−4.70 kcal mol−1), TfdB-JLU_P316A (−4.80 kcal mol−1) and TfdB-JLU_W222A (−4.87 kcal mol−1). His47 in the active site is suggested to play the key role in substrate deprotonation,38 and this residue should not be mutated. Due to the above reasons, Pro316 and Trp222 are selected as key residues affecting substrate promiscuity since the mutation of these two sites to alanine results the increase of free energy, and do not change the binding site significantly.
Table 3 Estimated free energy of binding of 2,4-DCP and TfdB-JLU_WT/TfdB-JLU-mutants
Proteins |
Energy (kcal mol−1) |
TfdB-JLU_WT |
−5.14 |
TfdB-JLU_H47A |
−4.70 |
TfdB-JLU_I48A |
−5.00 |
TfdB-JLU_W222A |
−4.87 |
TfdB-JLU_P316A |
−4.80 |
TfdB-JLU_F424A |
−4.98 |
Saturation mutagenesis is employed in protein engineering and genome-editing efforts to generate libraries that span amino acid design space for functional improvements. In this study, we perform site-saturation mutations for Pro316 and Trp222. 2,4-DCP is docked into these protein variants, respectively. As the Table S.3† shows, the free energy is reduced when substrate is docked into TfdB-JLU_P316Q. Hence, this mutation has a catalytic effect on binding. In terms of TfdB-JLU_P316Q, although the non-bond interaction of substrate and TfdB-JLU_P316Q is reduced (Table S.4†), an increase in hydrogen bonding is observed (Table 1). As shown in Fig. 3, the mutation of P316Q increases steric hindrance at the active site of enzyme and pushes the substrate to the direction of H47. This mutation results in the formation of hydrogen bonding between the substrate and H47, which might be the reason for the significant decrease in the binding free energy of the substrate and the TfdB-JLU_P316Q. Docking experiments have also been performed on P316N (least change in free energy of binding) and P316I (highest increase in free energy of binding). As shown in Fig. S.5†, little bonding change has been observed in cases of TfdB-JLU_WT and TfdB-JLU_P316N. However, the interaction of substrate with TfdB-JLU-P316I differed greatly from that of TfdB-JLU_WT with the disappearance of hydrogen bond. No corresponding saturated mutants for Trp222 show reduced free energy value (Table S.3†). Thus, we select TfdB-JLU_P316Q for further experiments.
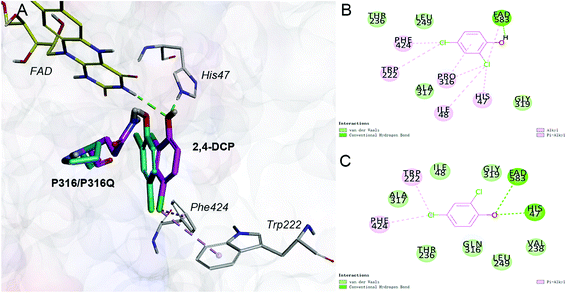 |
| Fig. 3 (A) Comparison of the interaction of 2,4-DCP-TfdB-JLU_P316Q complex with 2,4-DCP-TfdB-JLU complex. The ligand 2,4-DCP and amino acid residue P316Q in 2,4-DCP-TfdB-JLU_P316Q complex is shown by magenta stick, the ligand 2,4-DCP and amino acid residue P316 in 2,4-DCP-TfdB-JLU complex is shown by cyan stick, FAD is shown by yellow line, and the amino acid residues in TfdB-JLU_P316Q are shown by white line. (B) The schematic diagram of the detail non-bond interaction formations of 2,4-DCP with the active site of TfdB-JLU_WT. (C) The schematic diagram of the detail non-bond interaction formations of 2,4-DCP with the active site of TfdB-JLU_P316Q. The hydrogen bond interactions are shown by green dashed line, and the hydrophobic interactions are shown by pink dashed lines. | |
Substrate promiscuity and activity of TfdB-JLU_P316Q toward 10 CP congeners
In the present study, TfdB-JLU_P316Q activities toward 10 selected CP congeners are investigated at 25 °C. As summarized in Fig. 4, TfdB-JLU_P316Q exhibits high activities toward all the 10 CP congeners compared to those of TfdB-JLU. This result supports our modelling study that P316 is the key residue for enzyme functional engineering. The results in Fig. 4 shows that TfdB-JLU exhibits low activities toward 3,4-DCP, 3,5-DCP, whereas the activities of TfdB-JLU_P316Q toward 3,4-DCP and 3,5-DCP improve by 2.36-fold and 2.66-fold, respectively. This result suggests that TfdB-JLU_P316Q exhibits substrate promiscuity toward selected CP congeners and its substrate scope is thus broader than that of TfdB-JLU because of its high activity. Enzymatic activity improvement patterns observed for the mono-, di- and tri-CPs are quite different. Interestingly, enzymatic activity increases by 3.45, 2.98, 2.65, 2.36 and 2.23-fold for some CP congeners including 2,5-DCP, 4-CP, 3,5-DCP, 3,4-DCP and CP, respectively. The activity of TfdB-JLU_P316Q for natural substrate 2,4-DCP increases only by 1.91-fold compared to that of TfdB-JLU.11 The activity improvements of TfdB-JLU_P316Q for 3-CP, 2,3-DCP, 2,6-DCP, and 2,4,5-TCP are only found to be 1.12, 1.23, 1.41 and 1.36-fold higher than that of TfdB-JLU, respectively. Moreover, the enzyme activity improvement toward CP congeners is found related to the chlorine ring substitution patterns of specific CP congeners (Fig. 4). The enzyme activity of TfdB-JLU_P316Q for 3-CP are approximately 3-fold higher than that for 4-CP. However, in terms of activity improvement, the activity improvement of TfdB-JLU_P316Q for 3-CP is about 2.6-fold lower than that for 4-CP. Similar results are also observed for those of 2,5-DCP and 2,6-DCP. The above results suggest that P316 residue is a key residue for exploring substrate promiscuity of TfdB-JLU toward CPs, especially for those of unfavourable CPs. The differences between these enzymatic activities are statistically significant (p < 0.05).
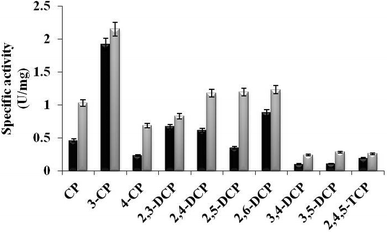 |
| Fig. 4 Specific activity of TfdB-JLU_WT (black) and TfdB-JLU_P316Q (gray) toward CPs. Abbreviations: CP, chlorophenol; DCP, dichlorophenol; TCP, trichlorophenol. | |
TfdB-JLU substrate promiscuity mechanism prediction
In this study, we would like to propose a preliminary assumption on the substrate promiscuity mechanism of TfdB-JLU toward CPs based on the structure and activity analyses. Molecular docking is used to analyze the interaction between the CPs ligands and TfdB-JLU_P316Q. An overall correlation of variation tendency is observed between the calculated free energy of binding and the substrate promiscuity. As Table S.5† shows, all ten CP congeners have the lower binding free energy with TfdB-JLU_P316Q than those with TfdB-JLU. The result in Fig. S.4† shows that the non-bond interactions between ligands such as 4-CP, 2,5-DCP and 3,5-DCP and TfdB-JLU_P316Q are stronger than those of ligands with TfdB-JLU_WT. Tables S.6–S.12† gives the detail parameters for these non-bonding interactions. Although a correlation is not observed between the calculated free energy of binding and the specific activity. Binding free energies of TfdB-JLU_P316Q with all CP congeners are lower than those of wild-type enzyme, whereas the specific activities of TfdB-JLU_P316Q toward all CP congeners are higher than those of TfdB-JLU_WT (Fig. S.6†). Thus, the higher decrease in free energy of binding might not necessarily lead to subsequent increased substrate promiscuity. It is notable that the higher decrease in free energy of binding dose not leads to subsequent increase in enzyme activity as shown in Fig. S.6.† For example, 2,4,5-TCP is shown to have the lowest free energy of binding out of the ten congeners (Table S.5†); but the specific activities of both TfdB-JLU_WT and TfdB-JLU_P316Q are the lowest for 2,4,5-TCP out of all the ten congeners (Fig. 4). In addition, the increase of free energy with the most extent does not witness the decrease of the specific activity with the most extent. For example, when 3,5-DCP is used as substrate, as the free energy slightly drops 0.03 kcal mol−1 (from −5.19 kcal mol−1 for TfdB-JLU_WT to −5.22 kcal mol−1 for TfdB-JLU_P316Q), the specific activity increases in 2.66-fold (Table S.5†). Comparatively, when 3-DCP is used as substrate, the free energy drops 0.20 kcal mol−1 (from −4.55 kcal mol−1 for TfdB-JLU_WT to −4.75 kcal mol−1 for TfdB-JLU_P316Q) (Table S.5†), but the increase in specific activity is only 1.12-fold. Therefore, the binding of the ligands to TfdB-JLU_P316Q is better than the binding to TfdB-JLU_WT due to not only its low binding free energy, but also the increase of hydrogen bond interaction, hydrophobic and halogen interactions. This result well supports the higher substrate promiscuity of TfdB-JLU_P316Q compared to TfdB-JLU_WT. And this engineered enzyme would be a good candidate for providing a starting point for designing and engineering novel biological catalyst for all CP congeners' removal.
Conclusions
This study exemplifies a site-directed mutagenesis of a 2,4-DCP hydroxylase TfdB-JLU based on rational design, and evolutionized variants exhibit broad substrate promiscuity for CPs than the wild-type TfdB-JLU. The modeling structure of TfdB-JLU is successfully used to interpret the results of in vitro evolution experiments and engineer substrate promiscuity. P316 is found to be a key amino acid associated with substrate promiscuity through saturation mutation screening and enzyme assay validation. The study of structures of TfdB-JLU and site-specific variants sheds light on the enzyme substrate promiscuity mechanism and will further aid protein engineering of biocatalysts with potential industrial applications.
Conflicts of interest
There are no conflicts to declare.
Acknowledgements
The authors gratefully acknowledge financial support from the National Natural Science Foundation of China (no. 31670796 and no. 81603459).
References
- M. S. Humble and P. Berglund, Eur. J. Org. Chem., 2011, 3391–3401 CrossRef.
- C. Li, X.-W. Feng, N. Wang, Y.-J. Zhou and X.-Q. Yu, Green Chem., 2008, 10, 616 RSC.
- K. Hult and P. Berglund, Trends Biotechnol., 2007, 25, 231–238 CrossRef PubMed.
- O. Khersonsky, C. Roodveldt and D. S. Tawfik, Curr. Opin. Chem. Biol., 2006, 10, 498–508 CrossRef PubMed.
- O. Khersonsky and D. S. Tawfik, Annu. Rev. Biochem., 2010, 79, 471–505 CrossRef PubMed.
- V. Polic and K. Auclair, Bioorg. Med. Chem., 2014, 22, 5547–5554 CrossRef PubMed.
- S. Lutz, J. Lichter and L. Liu, J. Am. Chem. Soc., 2007, 129, 8714–8715 CrossRef PubMed.
- Y. Li, H. Yu, Y. Chen, K. Lau, L. Cai, H. Cao, V. K. Tiwari, J. Qu, V. Thon, P. G. Wang and X. Chen, Molecules, 2011, 16, 6396–6407 CrossRef PubMed.
- H. Huang, C. Pandya, C. Liu, N. F. Al-Obaidi, M. Wang, L. Zheng, S. Toews Keating, M. Aono, J. D. Love, B. Evans, R. D. Seidel, B. S. Hillerich, S. J. Garforth, S. C. Almo, P. S. Mariano, D. Dunaway-Mariano, K. N. Allen and J. D. Farelli, Proc. Natl. Acad. Sci., 2015, 112, E1974–E1983 CrossRef PubMed.
- E. A. S. Brunialti, P. Gatti-Lafranconi and M. Lotti, Biochimie, 2011, 93, 1543–1554 CrossRef PubMed.
- J. Aranda, M. Roca and I. Tuñón, Org. Biomol. Chem., 2012, 10, 5395 Search PubMed.
- J. M. Schuller, G. Zocher, M. Liebhold, X. Xie, M. Stahl, S. M. Li and T. Stehle, J. Mol. Biol., 2012, 422, 87–99 CrossRef PubMed.
- J. D. Hayes, J. U. Flanagan and I. R. Jowsey, Annu. Rev. Pharmacol. Toxicol., 2005, 45, 51–88 CrossRef PubMed.
- R. Chandra and P. Chowdhary, Environ. Sci.: Processes Impacts, 2015, 17, 326–342 Search PubMed.
- A. S. de Miranda, L. S. M. Miranda and R. O. M. A. de Souza, Biotechnol. Adv., 2015, 33, 372–393 CrossRef PubMed.
- H. Ren, Q. Li, X. Fang and D. Yu, RSC Adv., 2015, 5, 86817–86824 RSC.
- H. Ren, Q. Li, Y. Zhan, X. Fang and D. Yu, Enzyme Microb. Technol., 2016, 82, 74–81 CrossRef PubMed.
- Y. C. Kao, C. Zhou, M. Sherman, C. A. Laughton and S. Chen, Environ. Health Perspect., 1998, 106, 85–92 CrossRef PubMed.
- B. F. O'Dowd, M. Hnatowich, J. W. Regan, W. M. Leader, M. G. Caron and R. J. Lefkowitz, J. Biol. Chem., 1988, 263, 15985–15992 Search PubMed.
- Q. Wu, P. Soni and M. T. Reetz, J. Am. Chem. Soc., 2013, 135, 1872–1881 CrossRef PubMed.
- J. Cassidy, L. Bruen, E. Rosini, G. Molla, L. Pollegioni and F. Paradisi, PLoS One, 2017, 12, 1–11 Search PubMed.
- M. Biasini, S. Bienert, A. Waterhouse, K. Arnold, G. Studer, T. Schmidt, F. Kiefer, T. G. Cassarino, M. Bertoni, L. Bordoli and T. Schwede, Nucleic Acids Res., 2014, 42, 252–258 CrossRef PubMed.
- S. Bienert, A. Waterhouse, T. A. P. De Beer, G. Tauriello, G. Studer, L. Bordoli and T. Schwede, Nucleic Acids Res., 2017, 45, D313–D319 CrossRef PubMed.
- P. Benkert, M. Biasini and T. Schwede, Bioinformatics, 2011, 27, 343–350 CrossRef PubMed.
- M. Kanteev, A. Bregman-Cohen, B. Deri, A. Shahar, N. Adir and A. Fishman, Biochim. Biophys. Acta, Proteins Proteomics, 2015, 1854, 1906–1913 CrossRef PubMed.
- K. Tamura, D. Peterson, N. Peterson, G. Stecher, M. Nei and S. Kumar, Mol. Biol. Evol., 2011, 28, 2731–2739 CrossRef PubMed.
- Q. Lei, H. Liu, Y. Peng and P. Xiao, Chin. Med., 2015, 10, 1–20 CrossRef PubMed.
- G. Morris and R. Huey, J. Comput. Chem., 2009, 30, 2785–2791 CrossRef PubMed.
- A. P. Norgan, P. K. Coffman, J. P. Kocher, D. J. Katzmann and C. P. Sosa, J. Cheminf., 2011, 3, 12 Search PubMed.
- W. Gai, Y. L. Zhang, L. Ai and Y. J. Qiao, Chin. J. Nat. Med., 2010, 8, 51–56 CrossRef.
- W. Forli, S. Halliday, R. Belew and A. Olson, AutoDock Version 4.2, 2012 Search PubMed.
- Y. Lu, Y. Yu, R. Zhou, W. Sun, C. Dai, P. Wan, L. Zhang, D. Hao and H. Ren, Biotechnol. Lett., 2011, 33, 1159–1167 CrossRef PubMed.
- M. H. M. Eppink, K. M. Overkamp, H. A. Schreuder and W. J. H. Van Berkel, J. Mol. Biol., 1999, 292, 87–96 CrossRef PubMed.
- B. Entsch, L. J. Cole and D. P. Ballou, Arch. Biochem. Biophys., 2005, 433, 297–311 CrossRef PubMed.
- R. A. Laskowski, M. W. MacArthur, D. S. Moss and J. M. Thornton, J. Appl. Crystallogr., 1993, 26, 283–291 CrossRef.
- T. Profiles, Methods Enzymol., 1997, 277, 396–404 Search PubMed.
- M. J. Dibley, N. Staehling, P. Nieburg and F. L. Trowbridge, Am. J. Clin. Nutr., 1987, 46, 749–762 CrossRef PubMed.
- C. Enroth, H. Neujahr, G. Schneider and Y. Lindqvist, Structure, 1998, 6, 605–617 CrossRef PubMed.
Footnote |
† Electronic supplementary information (ESI) available. See DOI: 10.1039/c8ra03229g |
|
This journal is © The Royal Society of Chemistry 2018 |
Click here to see how this site uses Cookies. View our privacy policy here.