DOI:
10.1039/C8RA02998A
(Paper)
RSC Adv., 2018,
8, 29578-29582
Facile microwave-assisted synthesis of In2O3 nanocubes and their application in photocatalytic degradation of tetracycline
Received
10th April 2018
, Accepted 10th August 2018
First published on 21st August 2018
Abstract
Novel In2O3 nanocube photocatalysts were successfully prepared by a facile microwave-assisted synthesis method. The obtained products are nano-sized with square corners and high crystallinity. The In2O3 nanocubes possessed high efficiency of electron–hole separation, resulting in high photocatalytic activities for the degradation of tetracycline under both visible light (λ > 420 nm) and full-range light irradiation (360–760 nm), the ratios of which are 39.3% and 61.0%, respectively. Moreover, the calculated positions of the CB and VB under our experimental conditions at the point of zero for In2O3 nanocubes are −0.60 V and +2.17 V, respectively. Note that the redox potentials of [O2/·O2−] and [·OH/OH−] are −0.33 V and +2.38 V, respectively, which means that the irradiated In2O3 nanocubes can reduce O2 into ·O2− without oxidizing OH− into ·OH. It can be concluded that ·O2− and h+ are the main active species of In2O3 in aqueous solution under visible light irradiation and full-range light irradiation.
1. Introduction
Antibiotics are a class of emerging contaminants in water because of the concern of their potential adverse effects on human health, mainly due to the increased bacterial resistance in the ecosystem. They have been found in a wide range of environmental samples including surface water, groundwater, wastewater and drinking water.1–3 Tetracycline (TC), as a group of broad-spectrum antibacterial compounds, has afforded the medical profession a number of powerful antibiotics active against a wide range of human and animal pathogens.4 Therefore, the removal of TC from the environment has become a mandatory issue.
Indium oxide (In2O3) is an n-type semiconductor. In2O3 has high conductivity and transparency, which makes it suitable for application in optoelectronics, gas sensors, photocatalysts and so on.5,6 Many methods including organic solution synthetic routes, solvothermal methods, sol–gel methods, chemical vapor deposition, template routes, hot injection techniques, hydrothermal methods and microwave-assisted synthesis have been used to synthesize In2O3 with different morphologies.6–13 Among these methods, microwave-assisted synthesis is a promising method for large-scale fabrication of nano-materials, owing to its simplicity, low cost and environmental friendliness. However, the investigations by this technique on In2O3 have been quite limited.
In our paper, the precursors In(OH)3 of In2O3 nanocubes are quickly synthesized with a microwave-assisted instrument. After annealing a In(OH)3 precursor at 400 °C for 120 min, the In2O3 nanocubes are obtained. This process is simple, clean and more economical than conventional methods. In addition, In2O3 nanocubes exhibit high photocatalytic degradation ratio of TC under visible-light irradiation and full-range light irradiation, which are 39.3% and 61.0%, respectively. Furthermore, it might present a new opportunity of the removal of TC under visible-light irradiation.
2. Experimental
2.1 Materials
All the chemicals were purchased from Sigma-Aldrich Chemical Co., Ltd (China) and used as received without further purification. Deionized (DI) water was used throughout the whole experiment.
2.2 Characterization
The crystal structure of samples was determined by X-ray diffraction (XRD) method using Cu Kα radiation (λ = 1.54178 Å). Scanning electron microscopy (SEM) images were collected on an S-4800 field emission SEM (FESEM, Hitachi, Japan). The chemical composition of the samples was determined by scanning electron microscope-X-ray energy dispersion spectrum (SEM-EDX) with an accelerating voltage of 25 kV. Transmission electron microscopy (TEM) images were collected on an F20 S-TWIN electron microscope (Tecnai G2, FEI Co.), using a 200 kV accelerating voltage. UV-vis diffused reflectance spectra of the samples were obtained from a UV-vis spectrophotometer (UV2550, Shimadzu, Japan), and BaSO4 was used as a reflectance standard.
2.3 Catalyst preparation
In a typical preparation procedure, In(NO3)3·4.5H2O (1 mmol) and urea (6 mmol) were dissolved in 100 mL deionized water. After stirring for 15 min, the obtained mixture was transferred into a quartz-glass container with capacity of 250 mL. The microwave reaction was carried out in a microwave reactor. The mixture was heated in the microwave reactor with the operating power of 800 W and working temperature of 100 °C for 120 min. After the container cooled to room temperature, the as-formed white precipitates were collected by centrifugation, washed with deionized water and absolute ethanol three times respectively, then dried in vacuum at 60 °C for 6 h. Finally, the obtained products were calcined in air at 400 °C for 2 h min with a heating rate 5 °C min−1 and cooled under air. The final products were In2O3 nanocubes.
2.4 Method of catalytic performance
The photodegradative reaction for TC was carried out at 298 K in a photochemical reactor under visible light. The photochemical reactor contains 0.1 g In2O3 photocatalyst and 20 mg L−1 of 100 mL TC solution. To determine the initial absorbance of samples, the reactor was kept into darkness for 30 min to reach absorption equilibrium. The photochemical reactor was irradiated with a 250 W xenon lamp which was located with a distance of 8 cm at one side of the containing solution. UV lights with wavelengths less than 420 nm were removed by a UV-cutoff filter in the visible-light-driven TC degradation experiment. The sampling analysis was conducted in 10 min interval. The photocatalytic degradation ratio (DR) was calculated by the following formula:
where A0 is the initial absorbance of TC that reached absorption equilibrium, while Ai is the absorbance after the sampling analysis. The TC absorbance was measured by a UV-vis spectrophotometer with the maximum absorption wavelength at 355 nm.
3. Results and discussion
3.1 Characterization of the catalyst
The crystallographic phase purity of the as-synthesized In2O3 nanocubes were characterized by X-ray diffraction (XRD). As shown in Fig. 1, all characteristic peaks of In2O3 nanocubes can be readily indexed to the monoclinic phase of In2O3, which is in good agreement with the standard card (JCPDS no. 01-0929).
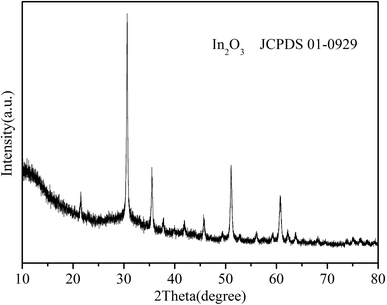 |
| Fig. 1 XRD pattern of In2O3 nanocubes. | |
The morphology and crystalline structure of the as-obtained nanocubes are visualized by SEM and TEM images in Fig. 2. In the low-magnified SEM image (Fig. 2a), the obtained samples are formed as uniform nanocubes. According to the high-magnified SEM image (Fig. 2b), the picture reveals that the thickness of the cube structure (ca.100 nm) is within nanoscale level. The TEM image (Fig. 2c) suggests that the angle of the corner is nearly 90°, which further confirms the square character. Fig. 2d shows the SEM-EDX image of the as-prepared In2O3 samples, in which the signals of In and O can be clearly observed, which well coincides with the elementary composition of In2O3. The only impurity signal of Pt is ascribed to the substrate of the SEM-EDX analysis itself. The spectrum further indicates that the as-prepared In2O3 samples are pure.
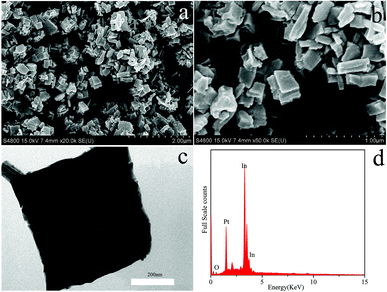 |
| Fig. 2 SEM images in (a) low and (b) high magnifications; (c)TEM image and (d) SEM-EDX spectrum of In2O3 nanocubes. | |
3.2 Crystallization process
The possible formation mechanism of In2O3 nanocubes was divided into two processes.14 Fig. 3a–c showed the morphology of the as-prepared samples with different microwave reaction time of 15 min, 30 min and 60 min, respectively. Fig. 3d shows the XRD patterns of the products obtained at 15 min, 30 min and 60 min, respectively. Firstly, the In(OH)3 precursor with nanosheet-based was prepared by a simple hydrothermal route. Then,In2O3 nanocubes were obtained under ambient pressure by the thermal decomposition of the In(OH)3 precursor at 400 °C for 2 h. In order to understand the formation of In2O3 nanocubes, we studied the influence of time parameter on the morphology of products systematically. The influence of reaction time on the formation of In2O3 nanocubes was investigated as a variable while keeping all other reaction parameters the same. We found that the reaction time played an important role in controlling the morphology of the In2O3 nanostructures. When the reaction time is less than 15 min (Fig. 3a), the nanosheet-based products were observed. Combined with the XRD results (Fig. 3d), we consider that the composition of these nanosheet-based products are mainly In(OH)3 but with a little of In2O3. When the reaction time reached 30 min, some sheet-like sheets formed gradually (Fig. 3b). Due to the ragged surface of these mutilated sheets, it can easily assume that the mutilated sheet-like structure is assembled by many In(OH)3 nanosheets. Combined with the XRD patterns (Fig. 3d), we found that the corresponding peaks of In2O3 begin to strengthen, while characteristic peak of In(OH)3 weaken gradually as the time went on. When the reaction time was increased to 60 min (Fig. 3c), the sheet structures begin to gather and nanocube-like aggregates appeared. Combined with the Fig. 3d, it is obvious that the intensity of In2O3 peaks are enhanced, but the In(OH)3 composition is still subsistent in samples. Finally, as the reaction time was prolonged to 120 min (Fig. 2), the individual nanocubes disappeared completely and the well-defined nanocubes morphology appeared. From the XRD results, we can clearly see that the In2O3 phase begin to be predominant in the XRD pattern (Fig. 1) and the final product exhibits the complete square-sheet structure (Fig. 2a), and the composition of square sheets is pure In2O3 (Fig. 1).
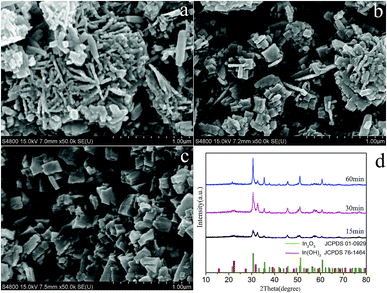 |
| Fig. 3 SEM images of obtained samples with different microwave reaction time: (a)15 min; (b) 30 min; (c) 60 min; (d) XRD patterns of the samples. | |
According to above discussion, the possible reaction mechanism for the formation of In2O3 nanocubes refers to the similar literature data,15,16 and the experimental results is as follows: First, CO(NH2)2 produce OH− in the microwave-assisted synthesis process (reaction (1)). Then, these precursors and In3+ formed In(OH)3 under the further stage of reaction (reaction (2)). Finally, they have transferred to In2O3 under a relatively longer period of thermal treatment (reaction (3)). Possible reaction formulas as followed:
|
CO(NH2)2 + 3H2O → 2NH4+ + 2OH− + CO2↑
| (1) |
|
In3+ + 3OH− → In(OH)3
| (2) |
|
2In(OH)3 → In2O3 + 3H2O
| (3) |
From the UV-vis absorption spectra (Fig. 4) of In2O3 nanocubes, the prepared In2O3 nanocubes have a strong absorption at ca. 448 nm, and the corresponding Eg of In2O3 nanocubes is 2.77 eV, resulting in the well response ability of In2O3 nanocubes to visible-light.17
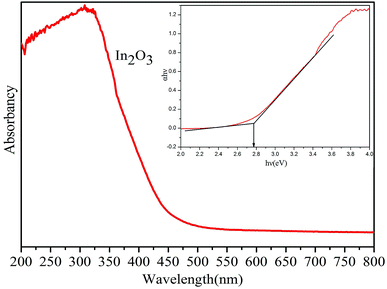 |
| Fig. 4 UV-vis absorption spectrum of as-obtained In2O3 nanocubes. | |
The band edge positions of conduction band (CB) and valence band (VB) of In2O3 nanocubes can be determined by the following equation: ECB = χ − Ee − 1/2Eg, where ECB is the conduction band edge potential, χ is the electronegativity of these semiconductor, Ee is the energy of free electrons on the hydrogen scale ca. 4.5 eV, Eg is the band gap of the semiconductor.
3.3 The kinetic study of photocatalytic degradationof TC
According to the Langmuir–Hinshelwood kinetics model,18–22 the photocatalytic process of TC can be expressed as the following apparent first-order kinetics equation (eqn (4)). |
 | (4) |
where C is the concentration of solute remaining in the solution at irradiation time of t and C0 is the initial concentration at t = 0. Kapp denotes the degradation rate constant.
The apparent rate constant (Kapp) has been chosen as the basic kinetic parameter for the different photocatalysts since it enables one to determine a photocatalytic activity independent of the previous adsorption period in the dark and the concentration of solute remaining in the solution.22
In order to further illustrate the photocatalytic reaction, the kinetic behavior is discussed. The photodegradation reaction kinetics of TC can be described by a Langmuir–Hinshelwood model according the report.23 The decomposition of TC approximated the first order kinetic.
The variations in ln(C0/C) as a function of irradiation times are given in Fig. 5. The calculated apparent rate constant Kapp values for In2O3 under visible light and full range light irradiation respectively are given in Table 1.
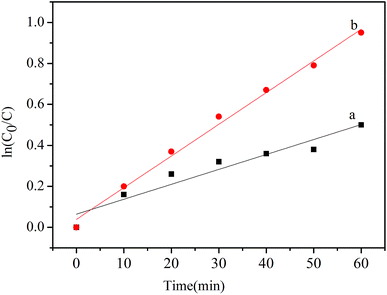 |
| Fig. 5 Kinetics of the photocatalytic degradation of In2O3 nanocubes (a) under visible light and (b) under full-range light for 60 min. | |
Table 1 Apparent rate constant values for photo-degradation of the TC solution under visible light and full range light respectively in 60 min
Condition |
Visible light |
Full range light |
Kapp (min−1) |
0.0073 |
0.0154 |
3.4 Discussion of catalytic mechanism
As can be seen in the band structures of In2O3 nanocubes (Fig. 4), the calculated positions of CB and VB in our experimental condition at the point of zero for In2O3 nanocubes are −0.60 V and +2.17 V respectively. Note that the redox potentials of [O2/·O2−] and [·OH/OH−] are −0.33 V and +2.38 V,24 respectively, which means the irradiated In2O3 nanocubes can reduce O2 into ·O2− without oxidizing OH− into ·OH. Thus, the photocatalytic oxidation process of In2O3 nanocubes can be classified as the ·O2− radical oxidation and h+.
On the basis of the article,14 we propose a plausible mechanism for the photodegradation of TC over the In2O3 nanocubes photocatalysts. The mechanistic pathway depicted in Scheme 1. As shown in Scheme 1, under the irradiation of visible light, the electron–hole pairs are excited from the VB of In2O3 nanocubes to its CB. The electron–hole pairs can then undergo further reactions with dissolved oxygen and water to form reactive radical species. The h+ can oxidize TC directly, which are found to be the ruling active species in the photodegradation of TC. Moreover, O2 in the water acts as an electron acceptor and generates ·O2− species, which are found to be the active species in the photodegradation of TC.
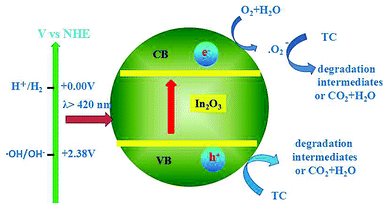 |
| Scheme 1 Mechanistic transfer of electrons and holes on In2O3 nanocubes photocatalysts under visible light illumination. | |
3.5 Catalytic performance
To probe the potential application of In2O3 nanocubes in photocatalytic degradation of TC, we evaluated the photocatalytic degradation of TC by In2O3 nanocubes. As shown in Fig. 6, the DR (60 min) of In2O3 nanocubes are 39.3% and 61.0% under visible light and full range light irradiation respectively.
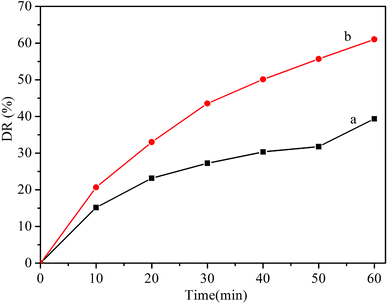 |
| Fig. 6 Photocatalytic degradation ratios of TC with In2O3 nanocubes under (a) visible-light and (b) full-range light irradiation for 60 min. | |
To evaluate the photostability of the as-prepared photocatalysts, the circulation experiments of the In2O3 for the photodegradation of TC was investigated. We have conducted four cycle experiments and obtained the photocatalytic degradation rate at the corresponding number of cycles. It was found that the degradation rate of the recycled products was only reduced by a small amount. As illustrated in Fig. 7, the photocatalytic activity of the In2O3 sample does not have noticeable change after the fourth recycles for the degradation of TC, in which the photocatalyst shows good photostability.
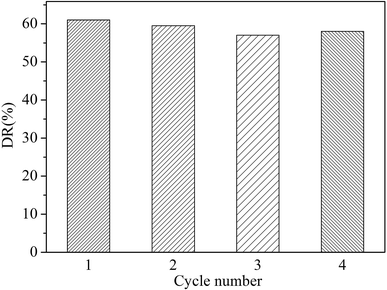 |
| Fig. 7 Photodegradation rate of TC in solution for four cycles using In2O3 photocatalyst. | |
4. Conclusions
In summary, as-obtained In2O3 nanocubes exhibit visible-light-driven activity for photocatalytic degradation of TC. Our work highlights the facile and fast hydrothermal synthesis of In2O3 photocatalysts and exhibits visible-light-driven activity for photocatalytic degradation of TC.
Conflicts of interest
There are no conflicts to declare.
Acknowledgements
The authors are grateful for scientific to research program funded by Shaanxi provincial education department. (Program No. 15JK1369) and Xi'an Technological University principal fund (Program No. XAGDXJJ18017)
Notes and references
- O. A. H. Jones, N. Voulvoulis and J. N. Lester, Water Res., 2014, 36, 5013 CrossRef.
- K. D. Brown, J. Kulis, B. Thomson, T. H. Chapman and D. B. Mawhinney, Sci. Total Environ., 2006, 366, 772 CrossRef PubMed.
- A. Pena, D. Chmielova, C. M. Lino and P. Solich, J. Sep. Sci., 2007, 30, 2924 CrossRef PubMed.
- A. Pena, J. R. Albertgarcia, L. J. G. Silva, C. M. Lino and J. M. Calatayud, J. Hazard. Mater., 2010, 179, 409 CrossRef PubMed.
- D. Caruntu, K. Yao, Z. X. Zhang, T. Austin, W. L. Zhou and C. J. O' Connor, J. Phys. Chem. C, 2010, 114, 4875 CrossRef.
- S. E. Lin and W. C. J. Wei, J. Am. Ceram. Soc., 2006, 89, 527 CrossRef.
- J. Du, M. Yang, S. N. Cha, D. Rhen, M. Kang and D. J. Kang, Cryst. Growth Des., 2008, 8, 2312 CrossRef.
- H. Kominami, T. Nakamura, K. Sowa, Y. Nakanishi, Y. Hatanaka and G. Shimaoka, Appl. Surf. Sci., 1997, 113/114, 519 CrossRef.
- D. G. Shchukin and R. A. Caruso, Chem. Mater., 2004, 16, 2287 CrossRef.
- W. Yin, M. Cao, S. Luo, C. Hu and B. Wei, Cryst. Growth Des., 2009, 9, 2173 CrossRef.
- Z. Q. Liu, D. H. Zhang, S. Han, C. Li, T. Tang, W. Jin, X. L. Liu, B. Lei and C. W. Zhou, Adv. Mater., 2013, 15, 1754 CrossRef.
- C. H. Liang, G. W. Meng, Y. Lei, F. Phillipp and L. D. Zhang, Adv. Mater., 2010, 13, 1330 CrossRef.
- F. V. Motta, R. C. Lima, A. P. A. Marques, E. R. Leite, J. A. Varela and E. Longo, Mater. Res. Bull., 2010, 45, 1703 CrossRef.
- M. M. Wu, C. Wang, Y. Zhao, L. S. Xiao, C. Zhang, X. Q. Yu, B. F. Luo, B. Hu, W. Q. Fan and W. D. Shi, CrystEngComm, 2015, 17, 2336 RSC.
- D. P. Dutta, M. Roy and A. K. Tyagi, Dalton Trans., 2012, 41, 10238 RSC.
- G. H Zhang, X. Zhang, Y. Wu and W. D. Shi, Micro Nano Lett., 2013, 8, 177 CrossRef.
- T. C. M. Muller, T. M. H. Tran, B. E. Pieters, A. Gerber, R. Carius and U. Rau, Appl. Phys. Lett., 2013, 103, 262108 CrossRef.
- S. Valencia, F. Cataño, L. Rios, G. Restrepo and J. Marín, Appl. Catal., B, 2011, 104, 300 CrossRef.
- Z. Y. Lu, Y. Y. Luo, M. He, P. W. Huo, T. T. Chen, W. D. Shi, Y. S. Yan, J. M. Pan, Z. F. Ma and S. Y. Yang, RSC Adv., 2013, 3, 18373 RSC.
- G. H. Zhang, W. S. Guan, H. Shen, X. Zhang, W. Q. Fan, C. Y. Lu, H. Y. Bai, L. S. Xiao, W. Gu and W. D. Shi, Ind. Eng. Chem. Res., 2014, 53, 5443 CrossRef.
- C. Y. Lu, J. J. Dang, C. T. Hou, Y. J. Jiang and W. S. Guan, Desalin. Water Treat., 2018, 104, 250 CrossRef.
- C. Zhao, M. Pelaezb, X. D. Duan, H. P. Deng, K. O'Shea, D. Fatta-Kassinos and D. D. Dionysiou, Appl. Catal., B, 2013, 134 –135, 83–92 CrossRef.
- J. F. Niu, S. Y. Ding, L. W. Zhang, J. B. Zhao and C. H. Feng, Chemosphere, 2013, 93, 1–8 CrossRef PubMed.
- M. R. Hoffmann, S. T. Martin, W. Choi and D. W. Bahnemann, Chem. Rev., 1995, 95, 69 CrossRef.
|
This journal is © The Royal Society of Chemistry 2018 |