DOI:
10.1039/C8RA02911C
(Paper)
RSC Adv., 2018,
8, 18139-18145
Degradation of tri(2-chloroethyl)phosphate by a microwave enhanced heterogeneous Fenton process using iron oxide containing waste†
Received
4th April 2018
, Accepted 8th May 2018
First published on 17th May 2018
Abstract
Iron oxide containing waste was first used in the microwave (MW) enhanced heterogeneous Fenton process for removing tri(2-chloroethyl)phosphate (TCEP) in aqueous solution. The operational parameters that affect the removal efficiency were investigated in detail. Comparing with the traditional Fenton-like reaction and heating assisted Fenton-like reaction, the MW enhanced heterogeneous Fenton process displayed superior treatment efficiency. The iron ore tailing can be reused eight times without significant decrease of catalytic efficiency. The major intermediates were identified and the possible degradation pathways of TCEP were proposed. The acute toxicity gradually decreased during the degradation process and the removal of TOC was 98.8% after 35 min reaction. This study could not only apply the waste tailing as a secondary resource to avoid the tedious preparation of the catalyst, but also proposes a rapid, low-cost and effective alternative method for the removal of polluted water containing TCEP.
1 Introduction
Organophosphate esters (OPEs) are widely used as flame retardants and plasticisers in a variety of products such as electronic equipment, building materials, and polyurethane foams.1 The increase in the trend of OPE consumption may become even greater in the future due to restrictions on the use of polybrominated diphenyl ethers (PBDEs).2 OPEs have already been detected in various environments3–8 and their levels in the environment will subsequently rise with their growing usage. The chlorinated OPEs, such as tri(2-chloroethyl)phosphate (TCEP), tri(2-chloroisopropyl)phosphate (TCPP) and tri(dichloropropyl)phosphate (TDCP), have been reported to be carcinogenic9 and neurotoxic. They are considered to be persistent pollutants10–12 and have been shown to resist biodegradation, chlorination and ozonation in treated waters.13
Owing to their widespread, toxicity and persistence, removal of the chlorinated OPEs in the contaminated environment is necessary and significant. As one of the promising techniques, advanced oxidation technology based on the combination of ultraviolet radiation (UV) with chemical oxidants has been reported for the removal of chlorinated OPEs from the environment using an UV/H2O2 system.13–16 Watts and Linden found UV/HOCl is not an effective treatment and little to no TCEP degradation was observed in the range of applied UV fluences (up to 1000 mJ cm−2), regardless of how much NaOCl was added prior to UV irradiation. A significant increase in the initial oxidant concentration and subsequent fraction of the applied UV light absorbed by H2O2 was required for UV/H2O2 for effective oxidation of TCEP.15 Ruan et al.13 had investigated the photodegradation of TCEP in aqueous solution by UV/H2O2 and the result shows that a long reaction time up to 60 min was needed for the removal of total organic carbon to reach 97%. To date, the relevant studies about OPEs are mainly to do with their pollution level in the environment, with research about their removal remaining rare.
As one of the AOPs, heterogeneous Fenton (Fenton-like) was a very effective method in removal of many hazardous organic pollutants in wastewaters, owing to its many unique advantages, e.g. no iron sludge is generated and easy separation of the catalyst from the treated stream.9 Various heterogeneous Fenton-like catalysts, such as carbon-Fe,10 Fe2(SO4)3,11 BiFeO312 and S-doped Fe2O3,7 were used for wastewater treatment. Unfortunately, the preparation of these catalysts was usually time-consuming and tedious. On the other hand, owing to the increase of industrial wastes and the fact that the use of waste tailing in the treatment of wastewater will reduce the cost of wastewater treatment, waste tailings as secondary resources have been of great importance to all countries in the world. Thus, there is an increasing interest in search of possible alternative uses of solid wastes as adsorption and catalysis for reutilization before their final discharge.14–18 Clarke et al.19 found that Mn oxide containing mine tailings from South Africa can decolorize a number of acid azo dyes and the decolorization of acid orange 7 was 42% after 2 h. However, to the best of our knowledge, the relevant research is very limited.
In addition, microwave (MW) has been widely used to promoting the oxidative degradation of biorefractory wastes11,20,21 due to its advantages of swiftness, high-efficiency and no pollution to environment. These investigations illustrated that MW was in favor of promoting the degradation efficiency of traditional treatment methods. Yang et al.11 presented MW enhanced Fenton-like processes for the treatment of high concentration pharmaceutical wastewater. The COD removal and UV254 removal reached to 57.5% and 55.1%, respectively, and BOD5/COD was enhanced from 0.165 to 0.470. Comparing with traditional Fenton-like reaction and conventional heating assisted Fenton-like reaction, MW enhanced Fenton-like process displayed superior treatment efficiency.
In the present study, the applicability of MW enhanced iron ore tailing Fenton-like process for the treatment of TCEP wastewater was investigated. The operational parameters such as MW power, initial pH, H2O2 dosage and iron ore tailings loading were investigated and optimized. The difference among the MW-Fenton-like, traditional Fenton-like reaction performed at ambient temperature and conventional heating assisted Fenton-like reaction performed at water bath was compared. The catalytic performance and reusability of the iron ore tailing waste were also investigated. The major intermediates were identified and the possible degradation pathways of TCEP were investigated. The acute toxicity of the treated compounds was evaluated by luminescent bacteria test to assess the effectiveness of the degradation samples.
2 Experimental
2.1 Reagents
Tri(2-chloroethyl)phosphate (TCEP) was obtained from Aldrich (Milwaukee, WI, USA). H2O2 (30%, v/v) was purchased from Fisher Company. HPLC-grade methanol, ethyl acetate and acetonitrile were obtained from Tedian Company and used without further purification. The luminescent bacteria Photobacterium phosphoreum T3 (P. phosphoreum) was purchased as freeze-dried reagents (0.5 g each bottle) from the Institute of Soil Science, Chinese Academy Sciences, Nanjing, China. They were stored at −20 °C and hydrated prior to testing. Ultrapure deionized water was obtained from a Milli-Q water purification system (Milli-Q system, Millipore, Beldford, MA) and was used in the preparation of all aqueous solutions. The tailing was dried at the time of collection and passed through a 200-mesh sieve. The physical and chemical properties of the iron ore tailing are shown in Table 1. A modified domestic MW oven with an intermittent power emission (Galanz, China) equipped with a condenser tube was used as experimental oven. The safety of the modified domestic MW oven was tested before experiment, and no leakage of microwave or other security question are found.
Table 1 The physical and chemical properties of the iron ore tailings
The physical properties |
Surface area(m2 g−1): 1.3 |
pH: 5.42 |
(%) Sand (2000–50 μm): 70.51 |
(%) Silt (50–2 μm): 25.86 |
(%) Clay (<2 μm): 3.63 |
— |
The chemical composition (percentage referred to oxide form) |
(%) Fe2O3: 65.89 |
(%) SiO2: 10.98 |
(%) CaO: 8.89 |
(%) ZnO: 4.46 |
(%) MgO: 1.98 |
(%) K2O: 1.2 |
(%) Al2O3: 0.96 |
(%) SO3: 0.932 |
(%) P2O5: 0.824 |
(%) Cl: 0.63 |
(%) CuO: 0.501 |
(%) BaO: 0.452 |
(%) Cr2O3: 0.428 |
(%) MoO3: 0.42 |
(%) MnO: 0.412 |
(%) TiO2: 0.268 |
(%) Nd2O3: 0.244 |
(%) Sb2O3: 0.19 |
(%) V2O5: 0.18 |
(%) ZrO2: 0.16 |
2.2 MW enhanced heterogeneous iron ore tailing Fenton-like produce
100.0 mL TCEP solution was added into a 250 mL beaker flask, and then mixed with some dosages of H2O2 and iron ore tailing. After being mixed well, the flask, equipped with a water reflux condenser connected through a communication pipe, was irradiated with maximal 800 MW output and frequency of 2450 MHz for the set time at the normal atmospheric pressure. Traditional Fenton-like reaction was performed with the same pH, H2O2 dosage and tailing dosage as MW enhanced Fenton-like reaction. Conventional heating assisted Fenton-like reaction experiment was carried out in water bath at the temperature identical to that of effluent of MW enhanced Fenton-like reaction, and the other parameters were same as the latter. All of experimental runs were performed in triplicate to diminish errors.
2.3 Analytical methods
Residual TCEP was extracted from 1 mL of UV–H2O2 treated solution with 1 mL ethyl acetate via rapidly mixed liquid–liquid extraction, separately. The extracted analytes were then separated and detected via 7890N gas chromatograph (Agilent Technologies, USA), equipped with split/splitless injector, a HP-5 capillary column (30 m × 0.32 mm i.d., 0.25 μm film thickness) and flame photometric detector (FPD). The column temperature was programmed as follows: initial oven temperature 80 °C (held for 1 min), increased to 140 °C at a rate of 20 °C min−1 (held for 2 min), and then increased to 280 °C at a rate of 4 °C min−1 (held for 6 min). The injector and detector temperatures were set at 250 °C. Helium (99.999%) was used as a carrier gas at a constant flow of 1.5 mL min−1, and nitrogen (99.999%) was used as a make-up gas at a flow rate of 20 mL min−1. Synthetic air (99.995%) and hydrogen (99.999%) were used as detector gases at flows of 100 and 65 mL min−1, respectively.
Intermediate products were analyzed by a Thermo Fisher Trace gas chromatography interfaced with a Polaris Q ion trap mass spectrometer (GC/MS, Thermo Fisher, USA) equipped with DB-5 fused-silica capillary column (30 m × 0.32 mm i.d., 0.25 mm film thickness). Prior to GC-MS analysis, the samples were extracted by the SPE cartridges Poly-Sery PSD. Before to use, all SPE cartridges were sequentially conditioned with 5 mL of methanol and water at a flow rate of 1 mL min−1. The loaded SPE cartridges were then eluted with 5 mL acetonitrile/ethyl acetate (1
:
1, v/v). The extracted solution was dehydrated using anhydrous sodium sulfate and concentrated to 1 mL by rotary evaporation. After the solvent was blown away by the gentle nitrogen, trimethylsilylation was carried out at 60 °C for 15 h using 0.2 mL of bis(trimethylsilyl)trifluoroacetamide (BSTFA). The initial temperature of the column oven was 40 °C and following 1 min hold at this temperature, and then increased up to 300 °C with a heating rate of 6 °C min−1. Helium was used as the carrier gas. Mass spectrometric detection was operated with 70 eV electron impact (EI) mode. Electron impact was used for ionization of samples. Some of the intermediates were identified by an identification program of the U.S. National Institute of Standards and Technology (NIST) library.
A Shimadzu TOC-5000A (combustion) analyzer was used to evaluate the mineralization efficiency of TCEP. The leaching of Fe2+ and Fe3+ was measured by NexIon 300 ICP-MS (PerkinElmer, USA).
3 Results and discussions
3.1 Effect of MW on removal efficiency
The effect of MW power on the removal efficiency of TCEP was studied and the result was illustrated in Fig. 1. The removal efficiency of TCEP increased with the increasing of MW power from 100 to 600 W. When MW was 600 W, TCEP was completely removed within 35 min. However, the removal efficiency of TCEP reduced with the further increasing of MW power. It may due to that the increasing of MW power result in the increasing temperature of the solution. The increasing temperature will enhance the activity of ˙OH, which will increase the removal efficiency of TCEP. In addition, when hydrogen peroxide in solution is exposed to MW irradiation generates extra hydroxyl radicals (eqn (1)) due to the excitation of the molecules to high vibrational and rotational energy levels,17,18 enhancing TCEP degradation. |
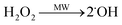 | (1) |
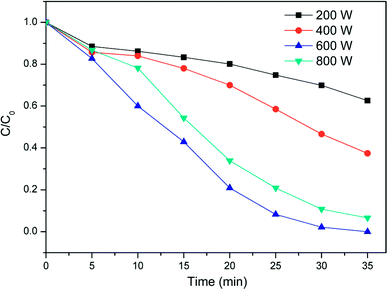 |
| Fig. 1 Effect of MW power on the degradation of TCEP in MW enhanced heterogeneous Fenton process using iron oxide containing waste. Experimental conditions: [iron ore tailing] = 250 mg L−1, pH = 3.0, [H2O2] = 1.98 mmol L−1. | |
However, when the MW power was higher than 600 W, the hydrogen peroxide can absorb sufficient energy to undergo decomposition instead of forming hydroxyl radicals17 (eqn (2)), and then the removal efficiency of TCEP reduced.
|
 | (2) |
3.2 Effect of iron ore tailing dosage on removal efficiency
The influence of the loading of iron ore tailing on the removal efficiency of TCEP was investigated. As shown in Fig. 2, the degradation efficiency of TCEP increased with the increasing of iron-containing waste dosage ranging from 50 to 250 mg L−1. It may due to the increasing dosage of catalyst will provide more active sites for the formation of ˙OH which is important for the degradation of TCEP.
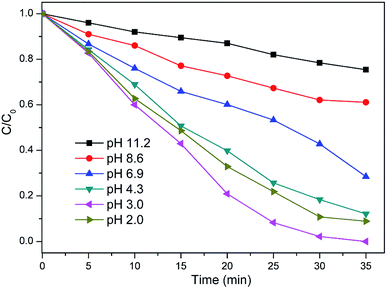 |
| Fig. 2 Influence of iron ore tailing loading on the degradation of TCEP in MW enhanced heterogeneous Fenton process using iron oxide containing waste. Other experimental conditions are as Fig. 1. | |
3.3 Effect of pH on removal efficiency
It is well-known that pH is very important for the efficiency of Fenton reaction. The previous study19 found that Fe(OH)+, which can catalytic decompose H2O2 to produce ˙OH, was formed at pH in the range from 2 to 4. Buxton et al.20 also reported that at pH around 2–3, the oxidation potential of ˙OH was 2.8 V, whereas, at pH near 7, it was 1.9 V, decreasing the removal capacity of organic substances. As shown in Fig. 3, the degradation of TCEP was faster at pH 3.0 than that of other pH. When the pH is below 3.0, the generation of hydroxyl radicals will be constrained due to the following reactions (4):21
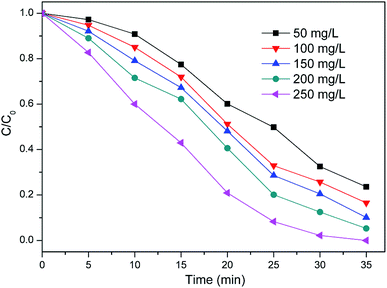 |
| Fig. 3 Effect of pH on the degradation of TCEP in MW enhanced heterogeneous Fenton process using iron oxide containing waste. Other experimental conditions are as Fig. 1. | |
It illustrates in Fig. 3 that TCEP conversion decreased when the initial pH was decreased from 3.0 to 2.0. The result was consistent with other researches that the reaction efficiency of the heterogeneous Fenton process tended to be highest at around pH 3.0 and decreased with the increasing of pH.22
3.4 Effect of H2O2 dosage on removal efficiency
Since the TCEP degradation is directly related to the concentration of the ˙OH produced by the catalytic decomposition of H2O2, the amount of H2O2 is important for the removal efficiency of TCEP. It was observed in Fig. 4 that the degradation of TCEP increased when H2O2 concentrations increased from 0.25 mM to 1.98 mM. However, when the concentration of H2O2 was 3.96 mmol L−1, the degradation of TCEP was not improved but decreased. It may due to that high concentration of H2O2 may have an inhibitory effect scavenge ˙OH to form ˙OOH and O2˙− (ref. 23) (eqn (4) and (5)): |
·OH + H2O2 → ·OOH + H2O
| (4) |
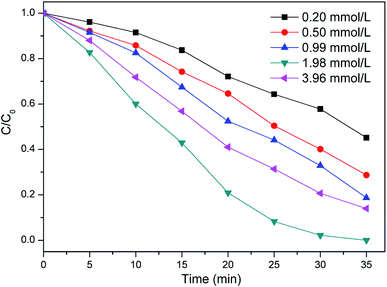 |
| Fig. 4 Effect of H2O2 dosage on the degradation of TCEP in MW enhanced heterogeneous Fenton process using iron oxide containing waste. Other experimental conditions are as Fig. 1. | |
The oxidation potentials of ˙OOH and O2˙− are much lower than that of ˙OH,24 thus the removal efficiency of TCEP decreased. The ˙OH scavenger ethanol (EtOH) was introduced into the reaction system to capture ˙OH in the process of TCEP degradation. The dosages referred to the previous studies.25,26 As shown in Fig. 5, the addition of EtOH leaded to a great decrease of the remove of TCEP. According to the above discussion, it is thought that ˙OH made a major contribution to the degradation of TCEP.
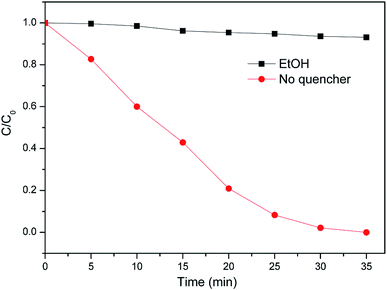 |
| Fig. 5 Effect of ˙OH scavenger on the degradation of TCEP in MW enhanced heterogeneous Fenton process using iron oxide containing waste. | |
3.5 Comparison with ambient temperature and conventional heating conditions
The removal efficiency of TCEP in MW enhanced heterogeneous Fenton system was compared with those in traditional Fenton-like reaction and conventional heating assisted Fenton-like reaction experiments. As shown in Fig. 6, the degradation efficiency of TCEP in MW enhanced heterogeneous Fenton system was higher than that in traditional Fenton-like process, which may owe to the high temperature and the production of extra ˙OH formed in MW enhanced Fenton-like process as discussed above. In addition, MW irradiation will result in the fast and selective heating of water and H2O2, which could accelerate the generation rate of ·OH27 and increase the reaction possibility between ·OH radicals and TCEP molecules. Consequently, the removal efficiency in MW enhanced heterogeneous Fenton system was higher than that in conventional heating assisted Fenton-like reaction. The removal efficiency in only MW system and MW assisted H2O2 system were also studied. As shown in Fig. 5, there was almost no degradation of TCEP in only MW system, which was due to that the energy of MW radiation (E = 0.4–40 kJ mol−1 at ν = 1–100 GHz) is insufficient to break the bonds of common organic molecules.28 The removal efficiency of TCEP was also very low (only 10%) after 35 min reaction in MW assisted H2O2 system. Based on the above-mentioned results, the synergistic effect between MW and heterogeneous Fenton process may play an important role in the high removal performance of the proposed system.
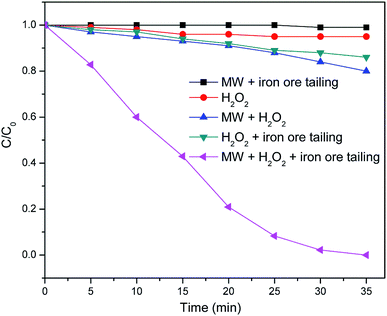 |
| Fig. 6 The degradation of TCEP in different processes. | |
3.6 The stability and reusability of iron-containing waste
The catalytic performance of iron-containing waste may decrease because of the soluble iron species generated by the action of ˙OH on the catalyst surface. The concentrations of Fe2+ and Fe3+ were not detected during the degradation and they were below the detections of ICP-MS. The result supports that heterogeneous Fenton process using iron-containing waste was carried out efficiently without significant iron leaching, which may owe to that iron species are recycled directly on the catalyst without significant diffusion into the solution phase in heterogeneous Fenton-like oxidation reaction.29 And this also may support iron-containing waste could be an excellent long-term stable catalyst. As illustrated in Fig. 7, the degradation efficiencies of TCEP are nearly the same in eighth successful cycles without obvious decrease of removal efficiency. In addition, there were no obvious differences in the physical–chemical properties of the iron ore tailings after the eighth reuse cycles (Table S1†).
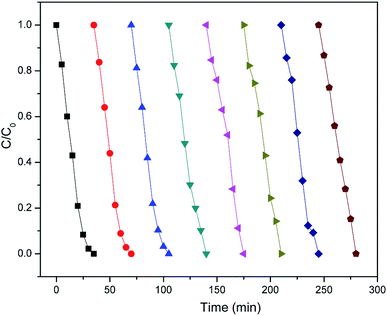 |
| Fig. 7 The catalytic activity of reused iron ore tailing on TCEP degradation. | |
3.7 Evaluation of possible degradation mechanism
As above-mentioned, hydroxyl radicals were supposed to be reactive species in the present system. The produced hydroxyl radicals attacked TCEP and the bonds of TCEP were broken gradually. The main intermediates identified by GC/MS were shown in Table S2† and the possible degradation pathway was proposed in Fig. 8. TCEP was degraded into some chlorinated small organic chemicals and then were further degraded to H3PO4, Cl− and oxalic acid which was finally mineralized to carbon dioxide and water.
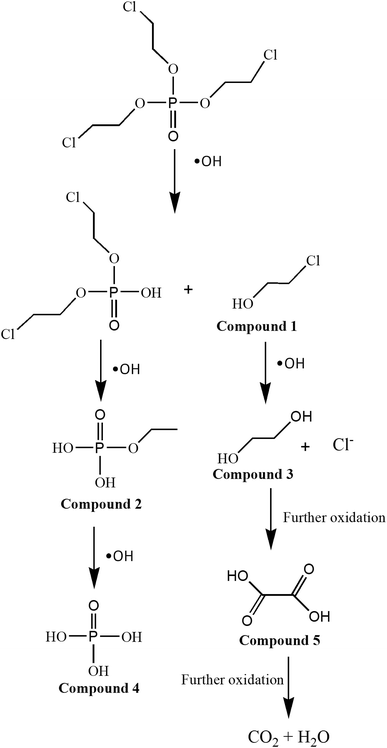 |
| Fig. 8 The possible reaction mechanism for the degradation of TCEP in MW enhanced heterogeneous Fenton process using iron oxide containing waste. | |
The mineralization and the acute toxic test were investigated during the degradation of TCEP. The potential toxicity of TCEP and its intermediates was examined by means of luminescent bacteria (Photobacterium phosphoreum T3 spp.) test following the procedures described in the national water quality standards of China.30 As shown in Fig. 9, the removal of TOC by the proposed system was 98.8% after 35 min reaction. As the oxidation process proceeded, the acute toxicity gradually decreased compared with the initial TCEP solution, indicating the toxic structures of TCEP and the immediate products were degraded into the less toxic products.
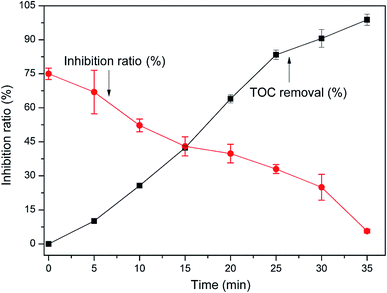 |
| Fig. 9 The change of TOC and the luminescent bacteria immobilization of TCEP in MW enhanced heterogeneous Fenton process using iron oxide containing waste. | |
4 Conclusions
Iron ore tailing was first used in MW-enhanced heterogeneous Fenton process for the removal of TCEP in aqueous solution. The degradation of TCEP was completed within 35 min in the proposed system. The synergistic effect between MW and heterogeneous Fenton process enhanced the removal of TCEP. Iron ore tailing can be reused eight times without the insignificant decrease of degradation efficiency. The removal of TOC by the proposed system was 98.8% after 35 min reaction. In addition, the acute toxicity gradually decreased as the degradation proceeded. Based on the results, the proposed system not only avoid the tedious preparation produce of the Fenton-like catalyst but also can be regarded as a fast and effective technology for the remediation of wastewaters containing TCEP.
Conflicts of interest
There are no conflicts to declare.
Acknowledgements
The authors acknowledge the key project of science and technology of Henan province (14A610010) and the training plan for young backbone teachers of universities in Henan province (2015GGJS-014).
References
- H. Carlsson, U. Nilsson, G. Becker and C. Ostman, Environ. Sci. Technol., 1997, 31, 2931–2936 CrossRef.
- A. Marklund, B. Andersson and P. Haglund, Environ. Sci. Technol., 2005, 39, 7423–7429 CrossRef PubMed.
- J. Tollbäck, D. Tamburro, C. Crescenzi and H. Carlsson, J. Chromatogr. A, 2006, 1129, 1–8 CrossRef PubMed.
- A. Möller, Z. Xie, A. Caba, R. Sturm and R. Ebinghaus, Environ. Pollut., 2011, 159, 3660–3665 CrossRef PubMed.
- J. A. Andresen, A. Grundmann and K. Bester, Sci. Total Environ., 2004, 332, 155–166 CrossRef PubMed.
- E. Martínez-Carballo, C. González-Barreiro, A. Sitka, S. Scharf and O. Gans, Sci. Total Environ., 2007, 388, 290–299 CrossRef PubMed.
- B. K. Schindler, K. Förster and J. Angerer, J. Chromatogr. B: Anal. Technol. Biomed. Life Sci., 2009, 877, 375–381 CrossRef PubMed.
- M. García-López, I. Rodríguez and R. Cela, J. Chromatogr. A, 2009, 1216, 6986–6993 CrossRef PubMed.
- T. Reemtsma, J. B. Quintana, R. Rodil, M. G. A. -López and I. Rodríguez, TrAC Trends in Analytical Chemistry, 2008, 27, 727–737 CrossRef.
- Y. Kawagoshi, S. Nakamura and I. Fukunaga, Chemosphere, 2002, 48, 219–225 CrossRef PubMed.
- Y. Yang, P. Wang, S. J. Shi and Y. Liu, J. Hazard. Mater., 2009, 168, 238–245 CrossRef PubMed.
- U. E. Bollmann, A. Möller, Z. Xie, R. Ebinghaus and J. W. Einax, Water Res., 2012, 46, 531–538 CrossRef PubMed.
- X. C. Ruan, R. Ai, X. Jin, Q. F. Zeng and Z. Y. Yang, Water, Air, Soil Pollut., 2004, 6, 599–605 Search PubMed.
- D. Santoro, M. Raisee, M. Moghaddami, J. Ducoste, M. Sasges, L. Liberti and M. Notarnicola, Environ. Sci. Technol., 2010, 44, 6233–6241 CrossRef PubMed.
- M. J. Watts and K. G. Linden, Environ. Sci. Technol., 2009, 43, 2937–2942 CrossRef PubMed.
- M. J. Watts and K. G. Linden, Water Res., 2008, 42, 4949–4954 CrossRef PubMed.
- V. Homem, A. Alves and L. Santos, Chem. Eng. J., 2013, 220, 35–44 CrossRef.
- N. Remya and J. G. Lin, Chem. Eng. J., 2011, 166, 797–813 CrossRef.
- C. E. Clarke, F. Kielar, H. M. Talbot and K. L. Johnson, Environ. Sci. Technol., 2010, 44, 1116–1122 CrossRef PubMed.
- G. Buxton, C. Greenstock and W. Helman, J. Phys. Chem. Ref. Data, 1998, 17, 513–886 CrossRef.
- M. S. Lucas and J. A. Peres, Dyes Pigm., 2006, 71, 236–244 CrossRef.
- X. B. Hu, B. Z. Liu, Y. H. Deng, H. Z. Chen, S. Luo, C. Sun, P. Yang and S. G. Yang, Appl. Catal., B, 2011, 107, 274–283 CrossRef.
- X. F. Xue, K. Hanna, M. Abdelmoula and N. S. Deng, Appl. Catal., B, 2009, 89, 432–440 CrossRef.
- J. H. Ramirez, F. J. Maldonado-Hodar, A. F. Perez-Cadenas, C. Moreno-Castilla, C. A. Costa and L. M. Madeira, Appl. Catal., B, 2007, 75, 312–323 CrossRef.
- G. Li, K. H. Wong, X. Zhang, C. Hu, J. C. Yu, R. C. Y. Chan and P. K. Wong, Chemosphere, 2009, 76, 1185–1191 CrossRef PubMed.
- Y. X. Chen, K. Wang and L. P. Lou, Journal of Photochemistry and Photobiology A, 2004, 163, 281–287 CrossRef.
- S. T. Liu, J. Huang, Y. Ye, A. B. Zhang, L. Pan and X. G. Chen, Chem. Eng. J., 2013, 215, 586–590 CrossRef.
- P. Muller, P. Klan and V. Cirkva, J. Photochem. Photobiol., A, 2003, 158, 1–5 CrossRef.
- J. He, W. H. Ma, J. J. He, J. C. Zhao and J. C. Yu, Appl. Catal., B, 2002, 39, 211–220 CrossRef.
- China, M.O.E.P., 1995.
Footnote |
† Electronic supplementary information (ESI) available. See DOI: 10.1039/c8ra02911c |
|
This journal is © The Royal Society of Chemistry 2018 |