DOI:
10.1039/C8RA02687D
(Paper)
RSC Adv., 2018,
8, 14906-14915
Synthesis of propellanes containing a bicyclo[2.2.2]octene unit via the Diels–Alder reaction and ring-closing metathesis as key steps†
Received
28th March 2018
, Accepted 3rd April 2018
First published on 19th April 2018
Abstract
The synthesis of propellanes containing bicyclo[2.2.2]octene via olefin metathesis approach is less explored. Herein, we describe a simple and convenient method to synthesize propellane derivatives containing a bicyclo[2.2.2]octene unit which are structurally similar to 11β-HSD1 inhibitors by sequential usage of the Diels–Alder reaction, C-allylation and ring-closing metathesis (RCM) as the key steps. Additionally, we expanded this approach to an endo-tricyclo[4.2.2.02,5]decene derivative which is a useful monomer for polymer synthesis and we have also synthesized basketene and anthracene-based propellanes using the same strategy.
Introduction
Propellanes are Y-shaped tricyclic molecules containing a bridgehead C–C bond. They can be classified as carbocyclic and heterocyclic frames and their physical or chemical properties vary with their ring size.1 Heterocyclic propellanes are generally found in nature whereas carbocyclic propellanes are rare and found in secondary metabolites.1c,d Synthesis of the propellane motif is difficult using conventional methods because of their quaternary nature2 and creation of vicinal quaternary carbons is not a trivial task. However, they can be assembled easily using a ring-closing metathesis (RCM) strategy.2,3 The synthesis of propellanes containing a bicyclo[2.2.2]octene system and their metathetic behaviour is worthy of systematic investigation.4
Bicyclo[2.2.2]octene is a rigid and strain free system which is present in diverse and challenging targets varying from biomolecules to natural products.5 Bicyclo[2.2.2]octene fused cyclic amides are potent bioactive molecules. 3,10-Dihydroxydielmentha-5,11-diene-4,9-dione 16a,7 is a diterpenoid isolated from Callitris macleayana heartwood and has a core of bicyclo[2.2.2]octene fused with a 6-membered carbocyclic ring (Fig. 1). Mitindomide 2 (Fig. 1) is a bicyclo[2.2.2]octene analogue which inhibits topoisomerase II and promotes DNA-interstrand cross-linking and thereby increases its antineoplastic activity.6b A water soluble derivative 3, which is structurally similar to mitindomide 2 but lacks the cyclobutane ring, exhibits antitumor activity.5,6c
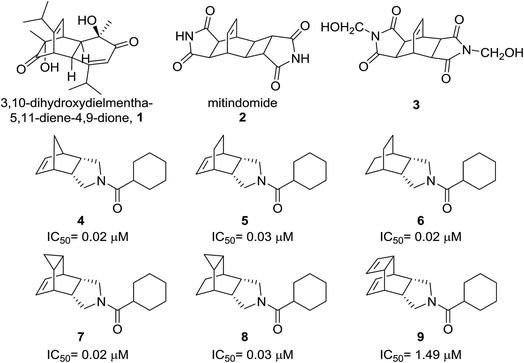 |
| Fig. 1 List of bicyclo[2.2.2]octane containing bioactive molecules (1–3) and 11β-HSD1 inhibitors (4–9). IC50 values were determined in mixed sex, human liver microsomes. | |
A recent report explains that the norbornene amide derivative 4 and the bicyclic[2.2.2]octane fused cyclic amide derivatives 5–9 are potent 11β-hydroxysteroid dehydrogenase type-I (HSD1) inhibitors and this inhibition was determined in mixed sex, human liver microsomes (Fig. 1).8 The introduction of lipophilic substituents has proven to be a successful strategy to enhance the inhibition of 11β-HSD1 activity. IC50 values of compounds 4–8 against 11β-HSD1 activity range from 0.02 to 0.03 μM. Meanwhile, compound 9 has an IC50 value of 1.49 μM which is little higher than the IC50 values of compound 4–8 with 83% of the enzyme inhibition. These results suggest that the bicyclo[2.2.2]octane amide derivatives fit well into the hydrophobic pocket of 11β-HSD1.8 In this regard, propellanes containing bicyclo[2.2.2]octene derivatives with enhanced hydrophobicity are worthy targets of investigation for human 11β-HSD1.
To the best of our knowledge, the synthesis of propellanes containing a bicyclo[2.2.2]octene system via olefin metathesis has been less explored. Ring-rearrangement metathesis (RRM) of the bicyclo[2.2.2]octene analogues 10 and 12 tethered to an endo olefin moiety is an excellent strategy for synthesizing hydrindanes 11 and 13 which are fused 7/6 and 6/6 decalines.9 However, the exo-N-phenyl bicyclo[2.2.1] derivative 14 underwent RRM to synthesize triquinane 15 and the endo-N-phenyl bicyclo[2.2.1] derivative 17 underwent ROM–RCM to synthesize propellane 18.3c Our approach is that the endo-N-phenyl bicyclo[2.2.2] derivative 19 didn’t go through ROM or RRM but underwent RCM to deliver bicyclo[2.2.2]octene propellane 20 (Scheme 1). Herein, we report a stereoselective synthesis of a new class of bicyclo[2.2.2]octene based propellane which is structurally similar to 11β-HSD1 inhibitors.
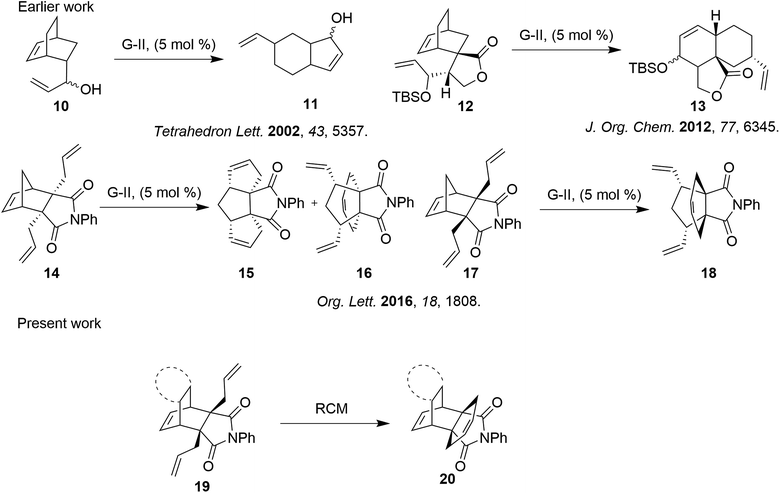 |
| Scheme 1 Metathesis of bicyclo[2.2.2]octene analogues. | |
A bicyclic monomer unit, endo-tricyclo[4.2.2.0]deca-3,9-diene (TD) 21, is extensively used in copolymerization to synthesize block copolymer 22 via ring-opening metathesis polymerization (ROMP) (Scheme 2).10 It seems that ROMP of an endo-tricyclo[4.2.2.0]deca-3,9-diene system is much faster than that of a norbornene system because of the high reactivity of the cyclobutene moiety present in the endo-tricyclo[4.2.2.0]deca-3,9-diene unit.11 Recently, Choi and co-workers have proposed a controlled ROMP of terminal alkyne monomer 23 to alkyne copolymer 24 by taking advantage of the strain in the cyclobutene ring of TD (Scheme 2).11c
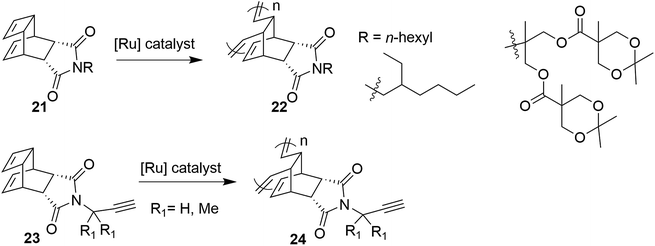 |
| Scheme 2 Polymerization of endo-tricyclo[4.2.2.0]deca-3,9-diene (TD). | |
Results and discussion
Our synthetic approach to propellane derivatives relies on understanding the metathetic behaviour of the endo-tricyclo[4.2.2.0]deca-3,9-diene derivative 29. Its synthesis starts with the preparation of the Diels–Alder (DA) adduct 27, which is easily assembled from commercially available cyclooctatetraene 25 and maleic anhydride 26 using a [4 + 2] cycloaddition reaction.11d,12 Next, anhydride 27 was treated with aniline in the presence of triethylamine in toluene at 120 °C to give compound 28 in 98% yield.3c Later, the endo adduct 28 was treated with an excess amount of allyl bromide in the presence of 1 M NaHMDS at −78 °C to deliver the diallyl compound 29 in 45% yield (Scheme 3).3c Compound 29, which contains three distinct types of olefinic moiety, is an interesting substrate for studying metathetic behaviour.
 |
| Scheme 3 Synthesis of diallyl endo-tricyclo[4.2.2.0]deca-3,9-diene 29. | |
To study the metathesis behaviour of compound 29, we screened various catalysts (Fig. 2) under different reaction conditions (Table 1).13 Among the various catalysts, only the G-I and G-II catalysts (Table 1, entries 1 and 2) gave a significant yield of the propellane derivative 30. The stereochemistry of compound 30 was further confirmed by single crystal X-ray diffraction studies (Fig. 3).14
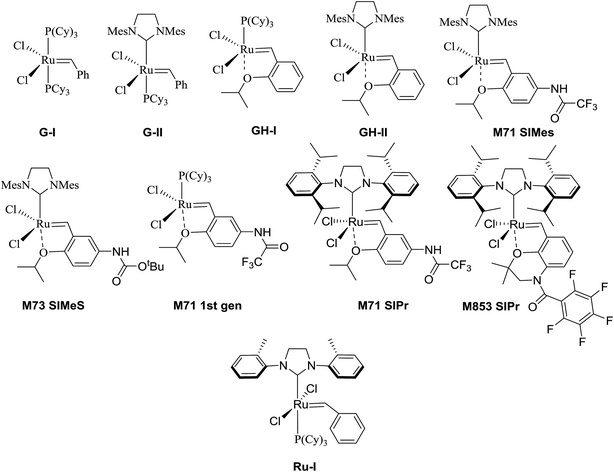 |
| Fig. 2 The various metathesis catalysts used in the present study. | |
Table 1 Metathetic behaviour of compound 29 with different catalysts
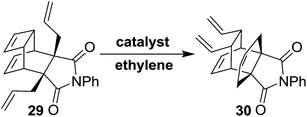
|
Entry |
Catalyst (mol%) |
Solvent |
Temp (°C)/time (h) |
Yield (%) |
1 |
G-I (5 mol%) |
CH2Cl2 |
rt/6 h |
65 |
2 |
G-II (5 mol%) |
CH2Cl2 |
rt/6 h |
58 |
3 |
GH-I (5 mol%) |
CH2Cl2 |
rt/5 h |
Trace |
4 |
GH-II (5 mol%) |
CH2Cl2 |
rt/3 h |
Trace |
5 |
G-I (10 mol%) |
Toluene |
Reflux/3 h |
10 |
6 |
G-II (10 mol%) |
CH2Cl2 |
rt/5 h |
35 |
7 |
G-I (5 mol%) + Ti(OPri)4 (catalytic) |
CH2Cl2 |
rt/4 h |
32 |
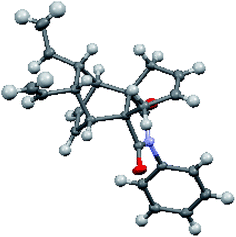 |
| Fig. 3 Crystal XRD structure of 30 with thermal ellipsoids drawn at the 50% probability level.14 | |
We were able to suppress the polymerization mode by carrying out the reaction under an ethylene atmosphere in the presence of the G-I and G-II catalysts. However, with the Hoveyda–Grubbs catalysts (Table 1, entries 3 and 4), the diallyl compound 29 was polymerized along with trace amounts of the propellane derivative 30. We didn’t observe any RRM product under these conditions. The crystal data of compound 30 indicate that due to their orientation, the vinyl groups are not favourably disposed to interact with the allyl groups to deliver the RRM product (Fig. 3).14
To understand the metathetic behaviour of the different olefinic moieties present in the bicyclo[2.2.2]octene derivative 29, we are interested in preparing compound 31 with the double bond present in the cyclobutene ring absent. In this regard, compound 28 was subjected to selective hydrogenation with the aid of 10% Pd/C–H2 to afford the partially saturated compound 31 in 96% yield.15 Later, it was subjected to allylation under 1 M NaHMDS conditions to generate the diallyl derivative 32 in 53% yield.3c Then, the diallyl compound 32 was subjected to a metathesis sequence with the G-II catalyst to obtain the propellane derivative 33 in 64% yield (Scheme 4).
 |
| Scheme 4 Synthesis of the endo-tricyclo[4.2.2.0]deca-3-ene propellane 33. | |
We have also studied the metathetic behaviour of the DA adduct 28 using various catalysts under different reaction conditions (Table 2). Among these, the G-I and G-II (Table 2, entries 1 and 2) catalysts gave the cyclobutene ring-opened product 34 in excellent yields. However, we didn’t observe the formation of the completely ring-opened product 35 under these conditions.
Table 2 The metathetic behaviour of compound 28 with different catalysts
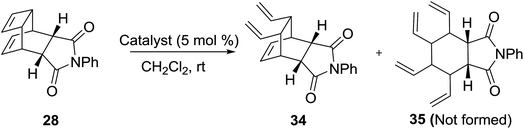
|
Entry |
Catalyst |
Time |
Product 34, yield (%) |
1 |
G-I |
6 h |
83 |
2 |
G-II |
6 h |
85 |
3 |
GH-I |
5 h |
71 |
4 |
GH-II |
12 h |
68 |
5 |
M71 SIMes |
12 h |
65 |
6 |
M71 1st gen |
12 h |
69 |
7 |
M71 SIPr |
12 h |
58 |
8 |
M853 SIPr |
12 h |
60 |
9 |
M73 SIMes |
12 h |
63 |
10 |
Ru-I |
5 h |
75 |
To understand the role of the cyclobutane ring in the metathetic behaviour of compound 29, we synthesized the DA adduct 37 from maleic anhydride 26 with 1,3-cyclohexadiene 36 and subsequently 37 was reacted with aniline in the presence of Et3N to deliver compound 38.3c,16 The metathetic behaviour of the endo adduct 38 was studied with various catalysts under different reaction conditions. Unfortunately, we didn’t obtain the expected ROM product 39. Later, the N-phenyl derivative 38 was treated with allyl bromide in the presence 1 M NaHMDS at −78 °C to afford the diallyl compound 40 in 45% yield. Subsequently, the diallyl derivative 40 was subjected to a metathesis sequence using the G-II catalyst (5 mol%) under ethylene to produce the propellane derivative 41 in 92% yield (Scheme 5). These results suggest that the double bond present in the cyclobutene of the endo-tricyclo[4.2.2.0]deca-3,9-diene system is highly reactive and the double bond present in the bicyclo[2.2.2]octene system is inert under these conditions. Our attempt to synthesize the exo derivative of 37 and 38 was not successful.
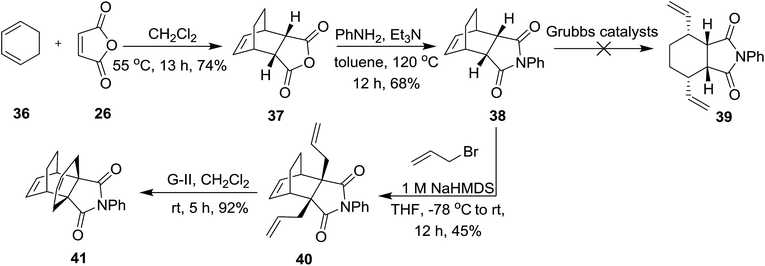 |
| Scheme 5 Synthesis of the bicyclo[2.2.2]octene propellane 41. | |
Later, this strategy was extended to design the basketene17 and anthracene-based propellane derivatives 45 and 50 respectively. Recently, our group reported the synthesis of cage propellanes,18 however, the basketene-based propellane synthesis is a worthy target for high energy systems. In this context, the DA adduct 27 was subjected to a [2 + 2] photo-cycloaddition reaction using a 450 W Hg lamp equipped with a Pyrex glass filter for 20 h to produce the cage compound 42,17d which on treatment with aniline in the presence of trimethylamine in toluene gave the cage compound 43 in 68% yield. Additionally, allylation of the N-phenyl derivative 43 with allyl bromide under 1 M NaHMDS conditions afforded the diallyl compound 44 which underwent RCM with the G-II catalyst at room temperature to produce the propellane derivative 45 in 65% yield (Scheme 6). The structure of 45 has been established on the basis of 1H NMR and 13C NMR spectral parameters and was further supported by the HRMS data. Along similar lines, anthracene derived propellane 50 was synthesized from the corresponding anhydride 4719 in three steps with a 31% overall yield (Scheme 6).
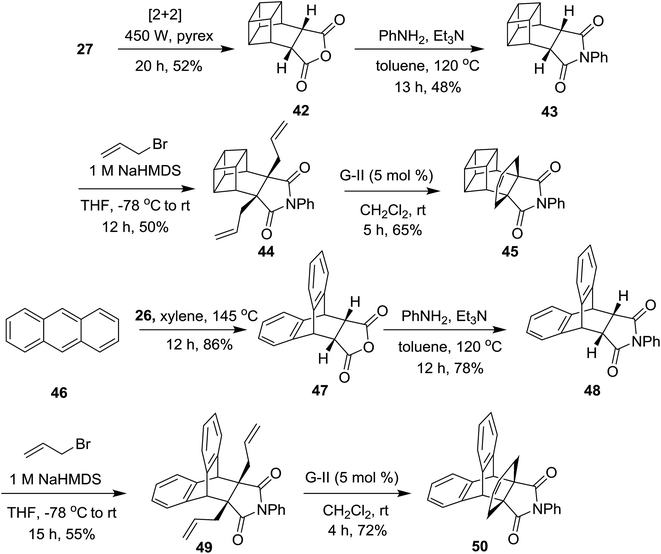 |
| Scheme 6 Synthesis of the basketene-based and anthracene derived propellane derivatives 45 and 50, respectively. | |
We have also carried out geometry optimization studies to illustrate the spatial demands and rigidity of these propellanes. Gas-phase calculations were carried out for 30, 33, 41, and 45 at the M062X/6-31G** level of theory. All calculations were carried out using the Gaussian 09 program.20 As shown by the optimized geometry study, ∠C1–C2–C7 and ∠C1–C2–C7 of the bicyclo[2.2.2]octene propellane derivatives 30, 33, and 41 are similar. However, the propellane derivative 45 was found to have larger bond angles compared to other derivatives due to distortion upon the [2 + 2] cyclo-addition reaction of 27. ∠C2–C3–C10 of the basketene derivative 45 is 89.56° which is close to the cubane bond angle of 90° (Fig. 4). Because of the rigidity of the propellane containing bicyclo[2.2.2]octene derivatives 30, 33, 41 and 45 and their structural similarity to the reported compounds 5–9, our methodology is useful for synthesizing biologically active derivatives such as these.
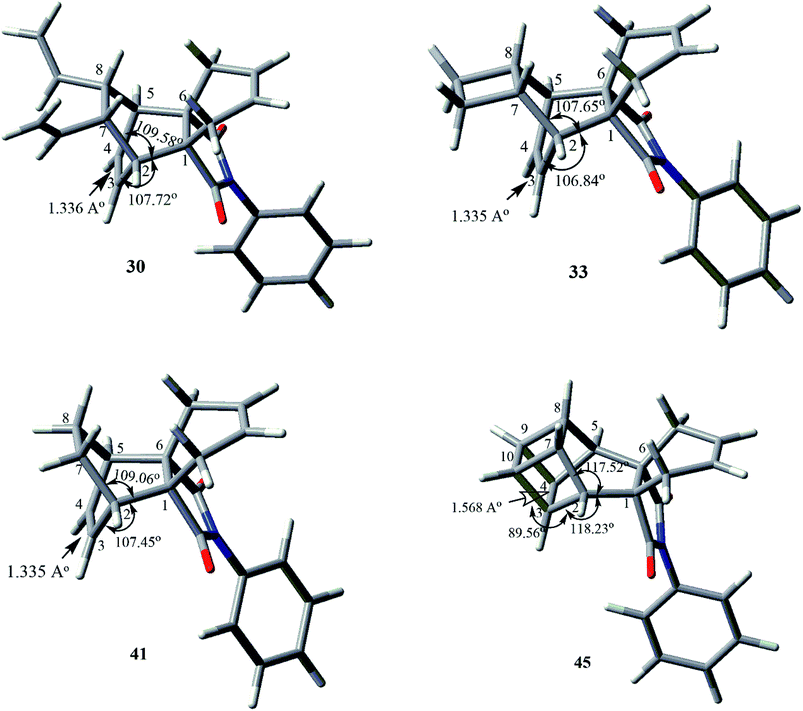 |
| Fig. 4 The optimized geometries of 30, 33, 41 and 45 at the M06-2X/6-31G** level of theory. C = grey, H = white, N = blue and O = red. | |
Experimental section
All commercially available reagents were used without further purification and the reactions involving air-sensitive catalysts or reagents were performed in degassed solvents. Moisture-sensitive materials were transferred using the syringe-septum technique and the reactions were maintained under a nitrogen atmosphere. Analytical thin-layer chromatography (TLC) was performed on (7.5 × 2.5 cm) glass plates coated with the Acme silica gel GF 254 (containing 13% calcium sulfate as a binder) using a suitable mixture of EtOAc and petroleum ether for development. Column chromatography was performed using Acme silica gel (100–200 mesh) with an appropriate mixture of EtOAc and petroleum ether. Coupling constants (J) are given in hertz (Hz) and chemical shifts are denoted in parts per million (ppm) downfield from the internal standard, tetramethylsilane (TMS). The abbreviations, s, d, t, q, m, dd, brs, td, tt and dt refer to singlet, doublet, triplet, quartet, multiplet, doublet of doublets, broad singlet, triplet of doublet, triplet of triplets and doublet of triplets, respectively. Infrared (IR) spectra were recorded on a Nicolet Impact-400 FT-IR spectrometer. Nuclear Magnetic Resonance (NMR) spectra were generally recorded on a Bruker (AvanceTM 400 or AvanceTM III 500) spectrometer operating at 400 or 500 MHz for 1H and 100.6 or 125.7 MHz for 13C nuclei. The high-resolution mass spectrometric (HRMS) measurements were carried out using a Bruker (Maxis Impact) or Micromass Q-ToF spectrometer.
The general procedure for the endo-anhydride synthesis
Compound 27. Off-white solid (190 mg starting from 150 mg of 26, 68%), Rf = 0.56 (10% EtOAc/hexane). 1H and 13C NMR spectra of compound 27 were matched with those of the reported literature.11d
Compound 37. Off-white solid (242 mg starting from 150 mg of 36, 74%), Rf = 0.48 (10% EtOAc/hexane). 1H and 13C NMR spectra of compound 37 were matched with those of the reported literature.16
Compound 42. White solid (180 mg starting from 345 mg of 27, 52%), Rf = 0.56 (10% EtOAc/hexane). 1H and 13C NMR spectra of compound 42 were matched with those of the reported literature.17d
Compound 47. White solid (650 mg starting from 490 mg of 46, 86%), Rf = 0.62 (10% EtOAc/hexane). 1H and 13C NMR spectra of compound 47 were matched with those of the reported literature.19a
The general procedure for the endo-N-phenyl adduct synthesis
Aniline (1 mmol, 1 equiv.) and trimethylamine (1 mL) were added to anhydride (1 mmol, 1 equiv.) in toluene in a sealed tube. The resulting mixture was heated at 120 °C for 12 h. After the depletion of anhydride, the extra solvent was distilled off. The residue was washed with diethyl ether (2 × 30 mL) and dried under high vacuum to yield a pure N-phenyl adduct.
Compound 28. Yield 98% (310 mg, starting from 240 mg of 27), white solid. 1H and 13C NMR spectra of compound 28 were matched with those of the reported literature.10a
Compound 38. White solid (465 mg starting from 480 mg of 37, 68%), Rf = 0.52 (10% EtOAc/hexane). 1H and 13C NMR spectra of compound 38 were matched with those of the reported literature.
Compound 43. White solid (65 mg starting from 100 mg of 42, 48%), Rf = 0.5 (10% EtOAc/hexane), mp: 165–167 °C. IR (neat): νmax C
O stretch at 1705 cm−1. 1H NMR (CDCl3, 400 MHz): δ = 7.49–7.43 (m, 2H), 7.41–7.35 (m, 1H), 7.25–7.21 (m, 2H), 3.38–3.32 (m, 4H), 3.29–3.25 (m, 2H), 3.13–3.09 (m, 2H), 2.99 (t, 2H, J = 1.9 Hz). 13C NMR (CDCl3, 100 MHz): δ = 178.50, 132.19, 129.28, 128.68, 126.65, 43.90, 38.95, 36.75, 32.79.HRMS (ESI, Q-ToF) m/z: found [M + H]+ = 278.1189, calculated = 278.1181 for C18H15NO2.
Compound 48. Pale yellow solid (295 mg starting from 300 mg of 47, 78%), Rf = 0.55 (10% EtOAc/hexane). 1H and 13C NMR spectra of compound 48 were matched with those of the reported literature.19b
Synthesis of compound 31
To compound 28 (320 mg, 1.15 mmol) in EtOAc, 10% Pd/C was added at room temperature the reaction mixture was allowed to stir for 5 h at room temperature until completion. The reaction mixture was passed through a celite column and washed with EtOAc several times to yield pure compound 31. White solid (310 mg, 96%), mp: 195–197 °C, Rf = 0.68 (5% EtOAc/hexane). IR (neat): νmax C
O stretch at 1704 cm−1. 1H NMR (CDCl3, 400 MHz): δ = 7.44 (t, 2H, J = 7.5 Hz), 7.36 (t, 1H, J = 7.5 Hz), 7.18 (d, 2H, J = 8.6 Hz), 6.43 (t, 2H, J = 3.5 Hz), 3.23 (brs, 2H), 2.83 (br. s, 2H), 2.62–2.57 (m, 2H) 2.10–2.02 (m, 2H). 13C NMR (CDCl3, 100 MHz): δ = 178.1, 132.2, 132.1, 129.1, 128.7, 126.6, 45.0, 37.0, 36.5, 22.3.
HRMS (ESI, Q-ToF) m/z: found [M + Na]+ = 302.1151, calculated = 302.1146 for C18H17NO2.
The general procedure for allylation using NaHMDS
NaHMDS (4.33 mmol, 8 eq.) and allyl bromide (4.3 mmol, 8 eq.) were added to the N-phenyl adduct compound (0.55 mmol, 1 eq.) in dry THF at −78 °C under a nitrogen atmosphere. The resulting mixture was brought to room temperature in 12 h and stirred at rt for 1 h. After the completion of the reaction, THF was removed under reduced pressure and the residue was partitioned between ethylacetate and water. The organic layer was concentrated and purified by column chromatography to yield the allylated compound.
Compound 29. Off-white solid (41 mg starting from 70 mg of 28, 45%), Rf = 0.75 (5% EtOAc/hexane), mp: 156.5–157.2 °C. IR (neat): νmax C
O stretch at 1764, 1701 cm−1. 1H NMR (CDCl3, 400 MHz): δ = 7.47–7.40 (m, 3H), 7.40–7.32 (m, 1H), 7.22–7.12 (m, 2H), 6.25–6.13 (m, 2H), 6.03 (dd, J = 2.5 Hz, 4.5 Hz, 2H), 5.82 (s, 2H), 5.27–5.17 (m, 4H), 3.13–3.03 (m, 4H), 2.71–2.50 (m, 5H). 13C NMR (CDCl3, 100 MHz): δ = 180.2, 137.6, 133.9, 132.1, 130.2, 129.2, 128.7, 126.7, 118.8, 51.8, 42.2, 38.9, 35.5. HRMS (ESI, Q-ToF) m/z: found [M + Na]+ = 380.1621, calculated = 380.1619 for C24H23NO2.
Compound 32. White solid (101 mg starting from 150 mg of 31, 53%), Rf = 0.56 (5% EtOAc/hexane), mp: 129.3–133.5 °C. IR (neat): νmax C
O stretch at 1742, 1705 cm−1. 1H NMR (CDCl3, 500 MHz): δ = 7.48–7.40 (m, 2H), 7.38–7.33 (m, 1H), 7.20–7.16 (m, 2H), 6.47 (dd, 2H, J = 3.32, 1.2), 6.20–6.07 (m, 2H), 5.19 (q, 1H, J = 1.44 Hz), 5.16–5.14 (m, 2H), 5.13 (brs, 1H), 3.01–2.97 (m, 2H), 2.82–2.75 (m, 2H), 2.57 (td, 1H, J = 4.36, 1.45 Hz), 2.53 (td, 1H, J = 5.81 Hz, 1.33 Hz), 2.42 (dd, 2H, J = 14.6 Hz, 8.3 Hz), 2.06–2.02 (m, 2H), 1.48–1.43 (m, 2H) ppm. 13C NMR (CDCl3, 125 MHz): δ = 180.3, 134.0, 133.7, 129.1, 128.6, 126.7, 50.9, 41.7, 35.6, 31.0, 22.0 ppm. HRMS (ESI, Q-ToF) m/z: found [M + H]+ = 360.1958, calculated = 360.1953 for C24H26NO2.
Compound 40. White solid (60 mg starting from 100 mg of 38, 45%), Rf = 0.8 (5% EtOAc/hexane), mp: 116.1–118.4 °C. IR (neat): νmax C
O stretch at 1705 cm−1. 1H NMR (CDCl3, 400 MHz): δ = 7.47–7.40 (m, 2H), 7.40–7.32 (m, 1H), 7.22–7.09 (m, 2H), 6.36–6.27 (m, 2H), 6.23–6.07 (m, 2H), 5.27–5.10 (m, 4H), 2.97 (brs, 2H), 2.72–2.46 (m, 4H), 1.88–1.76 (m, 2H). 13C NMR (CDCl3, 100 MHz): δ = 180.5, 134.2, 132.2, 129.2, 128.7, 126.7, 118.7, 51.5, 37.5, 35.9, 18.4. HRMS (ESI, Q-ToF) m/z: found [M + Na]+ = 356.1624, calculated = 356.1626 for C22H23NO2.
Compound 44. Colourless liquid (25 mg starting from 40 mg of 43, 50%), Rf = 0.74 (5% EtOAc/hexane). IR (neat): νmax C
O stretch at 1708 cm−1. 1H NMR (CDCl3, 500 MHz): δ = 7.47–7.43 (m, 2H), 7.39–7.37 (m, 1H), 7.20–7.18 (m, 2H), 6.17–6.06 (m, 2H), 5.18–5.12 (m, 4H), 3.28 (sex, 4H, J = 3.0 Hz), 3.27–3.23 (m, 2H), 3.15–3.12 (m, 4H), 2.65 (dd further split into triplet, 2H, J = 1.28 Hz, 6.24 Hz, 14.52 Hz), 2.43 (dd, 2H, J = 8.04 Hz, 14.52 Hz). 13C NMR (CDCl3, 125 MHz): δ = 180.0, 134.3, 129.2, 128.6, 126.7, 118.4, 46.5, 43.0, 39.2, 37.1, 37.0, 36.5. HRMS (ESI, Q-ToF) m/z: found [M + Na]+ = 380.1621, calculated = 1619 for C24H23NO2.
Compound 49. White solid (67 mg starting from 100 mg of 48, 55%), Rf = 0.75 (5% EtOAc/hexane), mp: 182.8–186.1 °C. 1H NMR (CDCl3, 400 MHz): δ = 7.50–7.13 (m, 13H), 6.53–6.40 (m, 2H), 6.34–6.17 (m, 2H), 5.33–5.00 (m, 4H), 4.65 (s, 2H), 2.42 (dd, 2H, J = 5.6 Hz, 14.4 Hz), 2.14 (dd, 2H, J = 8.5 Hz, 14.6 Hz). 13C NMR (CDCl3, 100 MHz): δ = 178.5, 140.0, 139.4, 133.7, 131.6, 129.1, 128.8, 127.1, 126.8, 126.7, 126.5, 125.3, 119.4, 55.7, 51.6, 37.3. HRMS (ESI, Q-ToF) m/z: found [M + Na]+ = 454.1777, calculated = 454.1783 for C30H25NO2.
The general procedure for metathesis
The compound (0.12 mmol) was dissolved in dry dichloromethane or toluene and degassed with nitrogen gas followed by ethylene gas. The catalyst was added to the reaction under an ethylene atmosphere and the reaction was carried out under the conditions given above. After the removal of the solvent, the residue was purified using column chromatography to obtain the corresponding compound.
Compound 30. White solid (51 mg starting from 70 mg of 29, 68%), Rf = 0.35 (5% EtOAc/hexane), mp: 177.6–181.5 °C. IR (neat): νmax C
O stretch at 1755, 1705 cm−1. 1H NMR (CDCl3, 500 MHz): δ = 7.49–7.40 (m, 2H), 7.40–7.33 (m, 1H), 7.20–7.11 (m, 2H), 6.46–6.35 (m, 2H), 6.10–6.00 (m, 2H), 5.66–5.51 (m, 2H), 5.12–4.97 (m, 4H), 3.09–2.99 (m, 2H), 2.88 (brs, 2H), 2.78–2.65 (m, 2H), 2.40 (d, 2H, J = 14.6 Hz). 13C NMR (CDCl3, 125 MHz): δ = 181.3, 139.5, 133.9, 132.3, 129.2, 128.7, 126.5, 115.6, 52.5, 43.0, 42.4, 28.3. HRMS (ESI, Q-ToF) m/z: found [M + Na]+ = 352.1317, calculated = 352.1312 for C22H19NO2.
Compound 33. White solid (42 mg starting from 70 mg of 32, 64%), Rf = 0.38 (5% EtOAc/hexane), mp: 115.2–118.3 °C. IR (neat): νmax C
O stretch at 1706 cm−1. 1H NMR (CDCl3, 500 MHz): δ = 7.44–7.38 (m. 2H), 7.37–7.31 (tt, 1H, J = 7.5 Hz, 1.4 Hz), 7.16–7.12 (m, 2H), 6.50–6.46 (dd, 2H, J = 4.3 Hz, 3.2 Hz), 5.97–5.94 (m, 2H), 2.86 (t, 4H, J = 3.0 Hz), 2.59 (dd, 1H, J = 2.45 Hz, 4.50 Hz), 2.56 (dd, 1H, J = 2.45 Hz, 4.50 Hz), 2.17 (d, 2H, J = 14.6 Hz), 2.10–2.03 (m, 2H), 1.54–1.49 (m, 2H). 13C NMR (CDCl3, 125 MHz): δ = 181.8, 134.1, 132.3, 129.1, 128.9, 128.6, 126.5, 52.1, 40.7, 32.5, 29.84, 28.6, 21.4.
Compound 34. White solid (yields given in Table 2 from 0.27 mmol of 28), Rf = 0.55 (5% EtOAc/hexane), mp: 150–152 °C. IR (neat): νmax = 2896, 2335, 1712, 1498, 1395, 1183, 913 cm−1. 1H NMR (CDCl3, 400 MHz): δ = 7.43 (tt, 2H, J = 7.2 Hz), 7.36 (tt, 1H, J = 7.32 Hz), 7.18 (td, 2H, J = 1.4 Hz), 6.33 (dd, 2H, J = 3.2 Hz, 1.0 Hz), 5.56–5.47 (m, 2H), 5.03 (dd, 2H, J = 17.0 Hz, 1.4 Hz), 4.98 (dd, 2H, J = 10.2 Hz, 1.6 Hz), 3.25 (brs, 2H), 3.10 (s, 2H), 2.75 (d, 2H, J = 7.3 Hz). 13C NMR (CDCl3, 100 MHz): δ = 177.5, 139.3, 131.9, 131.8, 129.2, 128.7, 126.5, 115.5, 46.8, 44.2, 38.3. HRMS (ESI, Q-ToF) m/z: found [M + H]+ = 306.1489, calculated = 306.1482 for C20H19NO2.
Compound 41. White solid (36 mg starting from 36 mg of 40, 92%), Rf = 0.3 (5% EtOAc/hexane), mp: 150.1–152.3 °C. IR (neat): νmax C
O stretch at 1703 cm−1. 1H NMR (CDCl3, 400 MHz): δ = 7.46–7.29 (m, 3H), 7.17–7.07 (m, 2H), 6.39–6.29 (m, 2H), 6.06–5.92 (m, 2H), 2.86–2.75 (m, 2H), 2.68–2.55 (m, 2H), 2.25 (d, J = 14.6 Hz, 2H), 1.97–1.80 (m, 2H), 1.40–1.29 (m, 2H). 13C NMR (CDCl3, 100 MHz): δ = 181.9, 131.5, 129.1, 129.1, 128.6, 126.6, 52.2, 36.6, 28.2, 19.5. HRMS (ESI, Q-ToF) m/z: found [M + Na]+ = 328.1306, calculated = 328.1313 for C20H19NO2.
Compound 45. White solid (7.1 mg starting from 10 mg of 44, 65%), Rf = 0.58 (5% EtOAc/hexane), mp: 150–152 °C. IR (neat): νmax C
O stretch at 1725, 1712 cm−1. 1H NMR (CDCl3, 500 MHz): δ = 7.43 (tt, 2H, J = 7.7 Hz, J = 1.5 Hz), 7.35 (tt, 1H, J = 7.40 Hz, 1.32 Hz), 7.16 (td, 2H, J = 7.05 Hz, 1.44 Hz), 5.95–5.92 (m, 2H), 3.32 (dd, 2H, J = 2.84 Hz, 1.44 Hz), 3.12–3.10 (m, 2H) 3.09–3.03 (m, 2H), 2.67 (dd, 2H, J = 2.6 Hz, 2.0 Hz), 2.63 (dd, 2H, J = 2.7 Hz, 1.9 Hz), 1.92 (d, 2H, J = 14.7 Hz). 13C NMR (CDCl3, 125 MHz): δ = 181.1, 132.4, 129.2, 128.6, 128.2, 126.6, 45.7, 42.2, 38.8, 37.5, 28.4. HRMS (ESI, Q-ToF) m/z: found [M + H]+ = 330.1489, calculated = 330.1488 for C22H19NO2.
Compound 50. White solid (35 mg starting from 46 mg of 49, 72%), Rf = 0.35 (5% EtOAc/hexane), mp: 132.1–136.5 °C. 1H NMR (CDCl3, 400 MHz): δ = 7.45–7.37 (m, 2H), 7.34–7.29 (m, 2H), 7.28–7.24 (m, 4H), 7.24–7.17 (m, 4H), 6.50–6.37 (m, 2H), 5.84–5.72 (m, 2H), 2.94–2.78 (m, 2H), 1.83–1.71 (m, 2H). 13C NMR (CDCl3, 100 MHz): δ = 180.0, 140.5, 140.2, 129.1, 128.8, 127.9, 127.2, 127.1, 127.0, 126.7, 126.6, 125.2, 57.3, 51.5, 30.2. HRMS (ESI, Q-ToF) m/z: found [M + Na]+ = 426.1461, calculated = 426.1470 for C28H21NO2.
Conclusion
We have developed a simple synthetic strategy to synthesize propellane containing bicyclo[2.2.2]octene derivatives which are structurally similar to 11β-HSD1 inhibitors. We have also designed interesting bicyclo[2.2.2]octene derivatives which contain a variety of unsaturated moieties to study their metathetic behaviour. The allylic groups present in compounds 40 and 49 undergo RCM to generate the corresponding propellane derivatives 41 and 50, respectively. Surprisingly, compound 40 didn’t undergo RRM due to the extra stability of the bicyclo[2.2.2]octene moiety towards metathesis. Similarly, the allyl groups present in compound 32 underwent RCM to generate the propellane derivative 33. However, compound 29 underwent RCM and ROM to generate the propellane derivative 30. We have extended this methodology to the cage compound 42 to produce the basketene-based propellane derivative 45. As shown by the geometry optimization studies, these propellane derivatives are rigid and the bond angles of the propellane derivatives 30, 33, and 41 are similar, whereas the basketene derivative 45 has larger values for its bond angles. These propellane derivatives will find useful applications in medicinal and polymer chemistry. Since cage molecules and propellanes have various applications as high energy systems, our results may be of interest in designing high-density materials.
Conflicts of interest
There are no conflicts to declare.
Acknowledgements
We are thankful to the Department of Science and Technology (EMR/2015/002053), New Delhi and CSIR (02(0272)/16/EMR-II), New Delhi for financial support. PS thanks UGC, New Delhi for financial support and the awarding of a SRF. S. K. thanks the DST for the awarding of a J. C. Bose fellowship (SR/S2/JCB-33/2010). We are also thankful to Ms Sreevani, G. and Mr Darshan Mhatre, Dept. of Chemistry, IIT Bombay for collecting the crystal data. We thank Omega Cat System for providing the M7-SIMes series of catalysts.
References
-
(a) D. Ginsburg, Acc. Chem. Res., 1969, 2, 121–128 Search PubMed;
(b) D. Ginsburg, Acc. Chem. Res., 1972, 5, 249–256 Search PubMed;
(c) A. J. Pihko and A. M. P. Koskinen, Tetrahedron, 2005, 61, 8769 Search PubMed;
(d) A. M. Dilmac, E. Spuling, A. de Meijere and S. Brase, Angew. Chem., Int. Ed., 2017, 56, 5684–5718 Search PubMed;
(e) D. Ginsburg, Top. Curr. Chem., 1987, 137, 1–17 Search PubMed;
(f) K. B. Wiberg, Acc. Chem. Res., 1984, 17, 379–386 Search PubMed;
(g) K. B. Wiberg, Acc. Chem. Res., 1996, 29, 229–234 Search PubMed;
(h) K. B. Wiberg, Chem. Rev., 1989, 89, 975–983 Search PubMed.
-
(a) L. M. Schneider, V. M. Schmiedel, T. Pecchioli, D. Lentz, C. Merten and M. Christmann, Org. Lett., 2017, 19, 2310–2313 Search PubMed;
(b) H. Torres-Gomez, K. Lehmkuhl, D. Schepmann and B. Wúnsch, Eur. J. Med. Chem., 2013, 70, 78–87 Search PubMed;
(c) R. Nakajima, N. Yamamoto, S. Hirayama, T. Iwai, A. Saitoh, Y. Nagumo, H. Fujii and H. Nagase, Bioorg. Med. Chem., 2015, 23, 6271–6279 Search PubMed;
(d) S. Kotha, D. Goyal and A. S. Chavan, J. Org. Chem., 2013, 78, 12288–12313 CrossRef CAS PubMed.
-
(a) S. Kotha, A. K. Chinnam and A. Tiwari, Beilstein J. Org. Chem., 2013, 9, 2709–2714 CrossRef PubMed;
(b) S. Kotha and R. Gunta, Beilstein J. Org. Chem., 2016, 12, 1877–1883 CrossRef CAS PubMed;
(c) S. Kotha and V. R. Aswar, Org. Lett., 2016, 18, 1808–1811 CrossRef CAS PubMed;
(d) S. Kotha and M. K. Dipak, Chem.–Eur. J., 2006, 12, 4446–4450 CrossRef CAS PubMed;
(e) S. Kotha and K. Mandal, Chem.–Asian J., 2009, 4, 354–362 CrossRef CAS PubMed.
-
(a) K. B. Wiberg, J. J. Caringi and M. G. Matturro, J. Am. Chem. Soc., 1990, 112, 5854–5861 CrossRef CAS;
(b) A. Osmont, L. Catoire and I. GÖkalp, Energy Fuels, 2008, 22, 2241–2257 CrossRef CAS.
- J. Hren, S. Polanc and M. Kočevar, ARKIVOC, 2008,(i), 209–231 CAS.
-
(a) R. M. Carman, L. K. Lambert, W. T. Robinson and J. M. A. M. Van Dongen, Aust. J. Chem., 1986, 39, 1843–1850 CrossRef CAS;
(b) H. M. Deutsch, L. T. Gelbaum, M. McLaughlin, T. J. Fleischmann, L. L. Earnhart, R. D. Haugwitz and L. H. Zalkow, J. Med. Chem., 1986, 29, 2164–2170 CrossRef CAS PubMed;
(c) B. B. Hasinoff, A. M. Creighton, H. Kozlowska, P. Thampatty, W. P. Allan and J. C. Yalowich, Mol. Pharmacol., 1997, 52, 839–845 CrossRef CAS PubMed.
- S. Dong, J. Zhu and J. A. Porco Jr, J. Am. Chem. Soc., 2008, 130, 2738–2739 CrossRef CAS PubMed.
- R. Leiva, C. Grinan-Ferre, C. Seira, E. Valverde, A. McBride, M. Binnie, B. Pérez, J. Luque, M. Pallás, A. Bidon-Chanal, S. P. Webster and S. Vázquez, Eur. J. Med. Chem., 2017, 139, 412–428 CrossRef CAS PubMed.
-
(a) T. L. Minger and A. J. Phillips, Tetrahedron Lett., 2002, 43, 5357–5359 CrossRef CAS;
(b) S. Bose, M. Ghosh and S. Ghosh, J. Org. Chem., 2012, 77, 6345–6350 CrossRef CAS PubMed;
(c) M. W. B. Pfeiffer and A. J. Phillips, J. Am. Chem. Soc., 2005, 127, 5334–5335 CrossRef CAS PubMed;
(d) P. E. Standen and M. C. Kimber, Tetrahedron Lett., 2013, 54, 4098–4101 CrossRef CAS;
(e) K.-W. Tsao, B. Devendar and C.-C. Liao, Tetrahedron Lett., 2013, 54, 3055–3059 CrossRef CAS.
-
(a) R. Charvet and B. M. Novak, Macromolecules, 2001, 34, 7680–7685 CrossRef CAS;
(b) R. Charvet and B. M. Novak, Macromolecules, 2004, 37, 8808–8811 CrossRef CAS;
(c) R. Charvet, S. Acharya, J. P. Hill, M. Akada, M. Liao, S. Seki, Y. Honsho, A. Saeki and K. Ariga, J. Am. Chem. Soc., 2009, 131, 18030–18031 CrossRef CAS PubMed.
-
(a) S. Shin, K.-Y. Yoon and T.-L. Choi, Macromolecules, 2015, 48, 1390–1397 CrossRef CAS;
(b) K. Kim, S. Shin, J. Kim and T.-L. Choi, Macromolecules, 2014, 13, 1351–1359 CrossRef;
(c) K. O. Kim, J. Kim and T.-L. Choi, Macromolecules, 2014, 47, 4525–4529 CrossRef CAS;
(d) K. O. Kim and T.-L. Choi, Macromolecules, 2013, 46, 5905–5914 CrossRef CAS.
- K. W. Glaeske and W. A. Donaldson, Mini-Rev. Org. Chem., 2012, 9, 31–43 CrossRef CAS.
- S. Kotha and M. K. Dipak, Tetrahedron, 2012, 68, 397–421 CrossRef CAS.
- The CCDC no of compound 30 is 1816911 as given in the supplementary crystallographic data for this paper.†.
- M. Rey-Carrizo, M. Barniol-Xicota, C. Ma, M. Frigole-Vivas, E. Torres, L. Naesens, S. Llabres, J. Juárez-Jiménez, F. J. Luque, W. F. DeGrado, R. A. Lamb, L. H. Pinto and S. Vazquez, J. Med. Chem., 2014, 57, 5738–5747 CrossRef CAS PubMed.
- M. Ceylan, İ. Karaman and M. Keçeci Sarıkaya, Org. Commun., 2013, 6, 102–109 CAS.
-
(a) S. Masamune, H. Cuts and M. G. Hogben, Tetrahedron Lett., 1966, 10, 1017–1021 CrossRef;
(b) W. G. Dauben and D. L. Whalen, Tetrahedron Lett., 1966, 31, 3743–3750 CrossRef;
(c) L. A. Paquette and R. S. Beckley, J. Am. Chem. Soc., 1975, 97, 1084–1089 CrossRef CAS;
(d) A. de Meijere, C.-H. Lee, B. Bengtson, E. Pohl, S. I. Kozhushkov, P. R. Schreiner, R. Boese and T. Haumann, Chem.–Eur. J., 2003, 9, 5481–5488 CrossRef CAS PubMed.
-
(a) S. Kotha, S. R. Cheekatla and D. S. Mhatre, Synthesis, 2017, 49, 5339–5350 CrossRef CAS;
(b) S. Kotha, S. R. Cheekatla and B. Mandal, Eur. J. Org. Chem., 2017, 4277–4282 CrossRef CAS;
(c) S. Kotha and S. R. Cheekatla, ChemistrySelect, 2017, 2, 6877–6881 CrossRef CAS.
-
(a) P. T. Huyen, M. Krivec, M. Kočevar, I. C. Bucur, C. Rizescu and C. Parvulescu, ChemCatChem, 2016, 8, 1146–1156 CrossRef CAS;
(b) H. Chen, E. Yao, C. Xu, X. Meng and Y. Ma, Org. Biomol. Chem., 2014, 12, 5102–5107 RSC.
-
(a) M. J. Frisch, G. W. Trucks, H. B. Schlegel, G. E. Scuseria, M. A. Robb, J. R. Cheeseman, G. Scalmani, V. Barone, B. Mennucci, G. A. Petersson, H. Nakatsuji, M. Caricato, X. Li, H. P. Hratchian, A. F. Izmaylov, J. Bloino, G. Zheng, J. L. Sonnenberg, M. Hada, M. Ehara, K. Toyota, R. Fukuda, J. Hasegawa, M. Ishida, T. Nakajima, Y. Honda, O. Kitao, H. Nakai, T. Vreven, J. A. Montgomery Jr, J. E. Peralta, F. Ogliaro, M. Bearpark, J. J. Heyd, E. Brothers, K. N. Kudin, V. N. Staroverov, R. Kobayashi, J. Normand, K. Raghavachari, A. Rendell, J. C. Burant, S. S. Iyengar, J. Tomasi, M. Cossi, N. Rega, N. J. Millam, M. Klene, J. E. Knox, J. B. Cross, V. Bakken, C. Adamo, J. Jaramillo, R. Gomperts, R. E. Stratmann, O. Yazyev, A. J. Austin, R. Cammi, C. Pomelli, J. W. Ochterski, R. L. Martin, K. Morokuma, V. G. Zakrzewski, G. A. Voth, P. Salvador, J. J. Dannenberg, S. Dapprich, A. D. Daniels, Ö. Farkas, J. B. Foresman, J. V. Ortiz, J. Cioslowski and D. J. Fox, Gaussian 09, Revision A.02, Gaussian, Inc., Wallingford, CT, 2013 Search PubMed;
(b) Y. Zhao and D. G. Truhlar, Theor. Chem. Acc., 2008, 120, 215–241 CrossRef CAS.
Footnote |
† Electronic supplementary information (ESI) available. CCDC 1816911. For ESI and crystallographic data in CIF or other electronic format see DOI: 10.1039/c8ra02687d |
|
This journal is © The Royal Society of Chemistry 2018 |
Click here to see how this site uses Cookies. View our privacy policy here.