DOI:
10.1039/C8RA02349B
(Paper)
RSC Adv., 2018,
8, 20576-20584
In situ hybridization of an MXene/TiO2/NiFeCo-layered double hydroxide composite for electrochemical and photoelectrochemical oxygen evolution†
Received
17th March 2018
, Accepted 28th May 2018
First published on 5th June 2018
Abstract
Electrochemical and photoelectrochemical (PEC) oxygen evolution reactions (OER) are receiving considerable attention owing to their important roles in the overall water splitting reaction. In this contribution, ternary NiFeCo-layered double hydroxide (LDH) nanoplates were in situ hybridized with Ti3C2Tx (the MXene phase) via a simple solvothermal process during which Ti3C2Tx was partially oxidized to form anatase TiO2 nanoparticles. The obtained Ti3C2Tx/TiO2/NiFeCo-LDH composite (denoted as TTL) showed a superb OER performance as compared with pristine NiFeCo-LDH and comercial IrO2 catalyst, achieving a current density of 10 mA cm−2 at a potential of 1.55 V versus a reversible hydrogen electrode (vs. RHE) in 0.1 M KOH. Importantly, the composite was further deposited on a standard BiVO4 film to construct a TTL/BiVO4 photoanode which showed a significantly enhanced photocurrent density of 2.25 mA cm−2 at 1.23 V vs. RHE under 100 mW cm−2 illumination. The excellent PEC-OER performance can be attributed to the presence of TiO2 nanoparticles which broadened the light adsorption to improve the generation of electron/hole pairs, while the ternary LDH nanoplates were efficient hole scavengers and the metallic Ti3C2Tx nanosheets were effective shuttles for transporting electrons/ions. Our in situ synthetic method provides a facile way to prepare multi-component catalysts for effective water oxidation and solar energy conversion.
1. Introduction
Over the past years, considerable efforts have been devoted to the exploration of clean and renewable energy sources. The electrochemical and photoelectrochemical (PEC) splitting of water is highly anticipated for the sustainable production of hydrogen and/or oxygen.1,2 The oxygen evolution reaction (OER), as one of the two half reactions of water splitting, involving complex electron and ion transfer that usually leads to sluggish kinetics and poor energy conversion efficiency.3,4 Traditional precious-metal-based oxides such as RuO2 and IrO2 are among the most active electrocatalysts towards the OER.5,6 However, their scarcity and accompanying high cost have limited their mass production and wide application. Recently, transition-metal-based compounds, such as sulfides,7,8 (oxy)hydroxides,9,10 oxides11–13 and layered double hydroxides (LDHs)14–17 have shown great potential for OER. The transition metal LDHs, which consist of positively charged layers where divalent and trivalent metal cations (e.g., Ni2+, Co2+, Mg2+, Al3+ and Fe3+) are coordinated to hydroxyl anions14–16 and charge-balancing anions (e.g., CO32−, Cl−, and/or SO42−)14,17 inserted between adjacent layers, are particularly attractive, because of their versatility in both chemical composition and morphology as well as relatively low costs. Several works have indicated that compared to binary LDHs, ternary ones exhibited further improved OER performance.18–20 For example, introducing Fe to NiCo-LDH could boost its OER performance, most likely due to the enhanced conductivity and increased level of structural disorder.9,18 More importantly, many LDHs are found to be semiconductors with suitable bandgaps in the visible-light range (e.g., Co2Fe-LDH, Ni0.75Fe0.25-LDH, CoAl-LDH, CuCr-LDH) and therefore show promises in PEC water splitting.21–25 However, LDHs generally suffer from poor carrier mobilities and aggregation during film forming,26,27 which hinder charge separation and transfer. Therefore, LDHs have been combined with conductive additives such as graphene-based materials and carbon nanotubes.28–30 Unfortunately, these conductive materials generally show poor surface hydrophilicity which may restrict easy access of aqueous electrolyte.
Recently, MXenes – a large family of layered materials have drawn considerable attention, which are commonly produced by the extraction of A from the ternary carbides or nitrides with a formula of Mn+1AXn, where M is an early transition metal, A is an A-group element and X is C and/or N.31–33 As one of the most widely studied MXene, Ti3C2Tx (Tx represents the terminal groups such as –(OH)x and –Fx),34 has demonstrated outstanding performance in various electrochemical applications,35,36 thanks to its good conductivity and hydrophilicity, as well as high electronegativity.37–39 Importantly, previous studies found that Ti3C2Tx nanosheets/flakes could provide Ti source for the surface growth of TiO2 for solar energy harvesting.40–42 Therefore, combining Ti3C2Tx/TiO2 and co-catalyst such as LDHs is expected to produce high performance PEC photocatalyst.
In this work, a composite of Ti3C2Tx/TiO2/NiFeCo-LDH (denoted as TTL) was prepared by growth of NiFeCo-LDH nanoplates on surfaces of Ti3C2Tx nanosheets solvothermally, during which TiO2 nanoparticles were simultaneously formed. The hybrid material showed excellent electrocatalytic activity toward OER and achieved a current density of 10 mA cm−2 in 0.1 M KOH at 1.55 V vs. RHE, which is among the best reported OER catalysts. In addition, the composite material was combined with BiVO4 for PEC-OER and exhibited the much enhanced activity in comparison with the pristine BiVO4. The excellent performance for both OER and PEC-OER resulted from a synergistic effect from all its components.
2. Experimental section
2.1 Chemicals
Titanium aluminum carbide (Ti3AlC2, ≥98%) was purchased from Tianmazhihui Technology Company (Beijing, China). Potassium hydroxide (KOH, 85%), hydrogen fluoride (HF, 40%) and Ni(II) chloride hexahydrate (99.9%) were purchased from J&K Chemical (Beijing, China). 1,4-Naphthalene dicarboxylic acid (1,4-H2NDC, 95%) was purchased from Aladdin (Shanghai, China). N,N-Dimethylformamide (DMF, 99.5%) and ethanol (99.7%) were purchased from Shanghai Chemical Reagent CO., LTD (Shanghai, China). Iridium oxide (IrO2, 99.9%), Nafion and Co(II) chloride hexahydrate (99.9%) were purchased from Sigma-Aldrich (Beijing, China). Iron(III) chloride hexahydrate (97%) was purchased from Stream Chemicals, INC. (Newburyport, USA). N,N-Dimethyltetradecylamine (90%) was purchased from Tokyo Chemical Industry CO., LTD. (Tokyo, Japan). n-Butanol (99%) was purchased from Shanghai Shenbo Chemical CO., LTD. (Shanghai, China). BiVO4 coated fluorine-doped SnO2 (FTO) glass substrates with a BiVO4 mass loading of 0.5 mg cm−2 were purchased from TOEI Technology (Hangzhou, China). The deionized (DI) water was purified using a Milli-Q3 System (Millipore, France). All chemicals were used without further purification.
2.2 Preparation of Ti3C2Tx nanosheets
5 mg of Ti3AlC2 powder was mixed with 30 mL of ethanol and ball-milled to form a homogeneous slurry. The slurry was then centrifuged and dried at 60 °C for 12 h before being ground into a fine powder. Then, 180 mg of this powder, 600 mg of 1, 4-H2NDC, 3 mL of 40% HF, and 60 mL of DI water were mixed in a 150 mL Teflon, heated and maintained at 180 °C for 6 h. After being cooled down to room temperature, the collected precipitate was washed with DMF for several times, and then sonicated in DMF for 16 h to yield isolated Ti3C2Tx nanosheets.
2.3 Preparation of NiFeCo-LDH nanoplates
NiFeCo-LDH nanoplates were synthesized using a solvothermal method in a reverse microemulsion system based on a previous report.42 Typically, 1.65 mL of n-butanol and 2.65 mL of N,N-dimethyltetradecylamine were mixed under sonication to form a reverse emulsion solution, into which, 450 μL of NiCl2 aqueous solution (0.2 mol L−1), 150 μL of FeCl3 aqueous solution (0.2 mol L−1), and 75 μL of CoCl2 aqueous solution (0.2 mol L−1) were added. The resulting mixture was then heated and maintained at 120 °C for 12 h in a 5 mL Teflon. After being cooled down to room temperature, the product was collected by centrifugation and washed with ethanol for at least 5 times.
2.4 Preparation of Ti3C2Tx/TiO2/NiFeCo-LDH composite
The preparation method of Ti3C2Tx/TiO2/NiFeCo-LDH was similar to that of NiFeCo-LDH nanoplates, except that 4 mg of Ti3C2Tx nanosheets were mixed with the aforementioned growth solution for NiFeCo-LDH and then vibrated at 850 rpm in an oscillator for 2 h before being transferred to a 5 mL Teflon and heated at 120 °C for 12 h.
2.5 Characterizations
X-ray diffraction (XRD, SmartLab Rigaku) was performed with Cu Kα radiation (λ = 1.54 Å) as the X-ray source. Scanning electron microscopy (SEM, Hitachi S-4800) was used for the morphological analysis. To gain the microstructure and composition information, transmission electron microscopy (TEM, Hitachi HT7700), high resolution transmission electron microscopy (HRTEM, JEOL 2100F), and energy-dispersive X-ray (EDX) spectroscopy analyses were performed. The elemental analysis and oxidation state study of the samples were carried out by X-ray photoelectron spectroscopy (XPS, PHI 5000 VersaProbe), and the binding energies were corrected for specimen charging effects using the C 1s level at 284.6 eV as the reference.
2.6 Electrochemical measurements
Typically, 2.5 mg of the active material, i.e. TTL, NiFeCo-LDH, Ti3C2Tx or IrO2, was mixed with 400 μL deionized water, 100 μL ethanol and 10 μL Nafion, followed by sonication to form a uniform dispersion. Then, 3 μL of such dispersion was drop-casted onto the surface of a pre-polished glassy carbon (GC) electrode (with a diameter of 3 mm) and then dried naturally at room temperature overnight. Cyclic voltammetry (CV) and linear sweep voltammetry (LSV) measurements were conducted in a three-electrode system on an electrochemical station (Autolab 302N). A Pt foil was used as the counter electrode, a 3 M Ag/AgCl electrode as the reference electrode, and the catalyst-modified GC rotating disk electrode (RDE) as the working electrode. All measured potentials versus Ag/AgCl were converted to the reversible hydrogen electrode (RHE) based on the Nernst equation below:45 |
E (vs. RHE) = E (vs. Ag/AgCl) + 0.059 × pH + EAg/AgCl
| (1) |
where E (vs. RHE) is the potential referred to RHE, E (vs. Ag/AgCl) is the applied potential against 3 M Ag/AgCl reference electrode, and EAg/AgCl is the standard potential of Ag/AgCl reference electrode. All the measurements were conducted in O2-saturated 0.1 M KOH solution (pH = 13). The CV and LSV curves were measured at scan rate of 100 mV s−1 and 2 mV s−1, respectively, at a rotating speed of 1600 rpm. Electrochemical impedance spectroscopy (EIS) measurements were conducted at a fixed potential of 1.57 V (vs. RHE) by applying an AC voltage with the amplitude of 5 mV over a frequency range of 100 kHz to 0.01 Hz. The electrical double-layer capacitance (Cdl) of the catalyst was measured from the double-layer charging curves using CV in a small potential range of 1.411–1.464 V (vs. RHE) without apparent faradaic processes occurring (Fig. S7†). The plot of the current density difference at 1.438 V (vs. RHE) against the scan rate (20, 40, 60, 80, 100 mV s−1) was linearly fitted, and its slope was the Cdl of the tested catalyst (Fig. 4c).
The rotating ring-disk electrode (RRDE) measurements were carried out in a three-electrode cell (CHI 700e, Shanghai, China) using a Pt foil as the counter electrode, a 3 M Ag/AgCl electrode as the reference electrode, and a catalyst-modified RRDE (Garmy RDE710, Beijing, China) as the working electrode with a rotating speed of 1600 rpm in O2-saturated 0.1 M KOH solution. The RRDE includes a glassy carbon disk with a diameter of 5 mm and an area of 0.1963 cm2, and a Pt ring with an area of 0.1859 cm2.
2.7 Photoelectrochemical measurements
All PEC-OER tests were conducted on an electrochemical workstation (CHI 660E, CH Instruments, Shanghai) in a three-electrode cell using a 3 M Ag/AgCl standard electrode as the reference and a Pt foil (1.5 cm × 1.5 cm) as the counter electrode. Typically, 2.5 mg active material such as LDH, Ti3C2Tx/TiO2 or TTL composite was mixed with 800 μL deionized water, 200 μL ethanol and 20 μL Nafion, followed by sonication to form a uniform dispersion. The concentration of Ti, Ni, Fe and Co elements in LDH, Ti3C2Tx/TiO2 and TTL dispersions were measured by inductively coupled plasma optical emission spectrometer (ICP-OES), respectively (Table S1†). For fabrication of a typical photoanode, the loading of TTL onto the 1 cm × 1 cm BiVO4/FTO substrate was 175 μg by drop-wise casting 70 μL of 2.5 mg mL−1 dispersion, which contained ∼142 μg LDH and ∼33 μg Ti3C2Tx/TiO2. In our control experiments, the loading of LDH on BiVO4/FTO was then ensured to be ∼142 μg (i.e. 57 μL of 2.5 mg mL−1 dispersion) and that of Ti3C2Tx/TiO2 was ∼33 μg (i.e. 13 μL of 2.5 mg mL−1 dispersion). After the dispersion was drop-casted onto BiVO4/FTO, the photoanode was dried naturally at room temperature overnight.
Fluorine-doped SnO2 (FTO) glass substrates with surface deposited BiVO4 (BiVO4/FTO, purchased from TOEI, Hangzhou, China) and TTL/BiVO4/FTO (both with a film area of 1 cm × 1 cm) acted as the photoanode. All measurements were performed at room temperature in 0.5 M potassium phosphate (pH = 7) solution. A 300 W xenon lamp (PLX-SEX300, PerfectLight, Beijing) was used to irradiate the photoanode from the back at an intensity of 100 mW cm−2 determined by a power meter (PL-MW2000, PerfectLight, Beijing). The photo energy conversion efficiency (η) of the photoanodes was calculated by the LSV curves using the following equation:46,47
|
η = [1.23 − E (vs. RHE) × J/Plight] × 100%
| (2) |
where 1.23 V is the equilibrium potential for OER,
E (
vs. RHE) is the applied potential
vs. the RHE,
J is the photocurrent density at the measured potential, and
Plight (100 mW cm
−2) is the power density of illumination.
EIS measurements of the photoanodes under illumination were performed on the electrochemical workstation with a 5 mV amplitude perturbation between 100 kHz and 0.01 Hz at open circuit potential.
The incident photon-to-current efficiency (IPCE) was calculated using the equation below:46
|
IPCE = [[1240 × (Jlight − Jdark)]/(P × λ)] × 100%
| (3) |
where
Jlight and
Jdark are the measured photocurrent and dark current density (mA cm
−2) obtained at 1.23 V
vs. RHE, respectively.
P is the measured irradiance at a specific wavelength (mW cm
−2), and
λ is the wavelength of the incident light (nm).
3. Results and discussion
3.1 Preparation and characterization of Ti3C2Tx/TiO2/NiFeCo-LDH composite
In a typical process, Ti3C2Tx nanosheets were firstly prepared by selectively removing Al layers in bulk Ti3AlC2 crystals with HF to obtain the layered structures as shown in the scanning electron microscopy (SEM) image in Fig. 1a, followed by sonication to produce exfoliated Ti3C2Tx nanosheets (Fig. 1b and c), and a typical nanosheet showed a thickness of ∼6 nm (Fig. S1†). The Ti3C2Tx nanosheets and Ti3AlC2 were further characterized by X-ray diffraction (XRD, Fig. 1d). The diffraction peak at 39°, which corresponds to the (104) planes of Ti3AlC2,48 is not observed in the pattern of Ti3C2Tx, suggesting the complete removal of Al layers. In addition, the (002) peak of Ti3C2Tx compared to that of Ti3AlC2 shifted from 9.3° to 8.6° and became broadened, indicating an enlarged interlayer spacing (from 0.94 nm to 1 nm) and possibly a reduced thickness. The chemical composition and oxidization states of the as-exfoliated Ti3C2Tx nanosheets were studied by X-ray photoelectron spectroscopy (XPS) (Fig. S2†), and surface-terminated groups like –(OH)x and –Fx were identified,49,50 which rendered the Ti3C2Tx nanosheets hydrophilic and negatively charge.
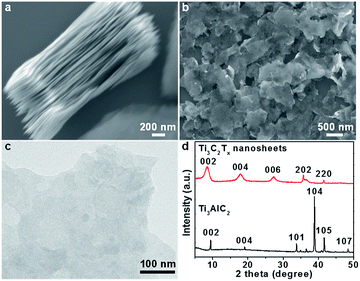 |
| Fig. 1 (a) SEM image of layered Ti3C2Tx. (b) SEM and (c) TEM images of isolated Ti3C2Tx nanosheets after sonication. (d) XRD patterns of Ti3C2Tx nanosheets and Ti3AlC2 bulk crystals. | |
The as-prepared Ti3C2Tx nanosheets were then used as synthetic templates for the growth of NiFeCo-LDH via a solvothermal reaction as illustrated in Scheme 1a. The synthetic process began with mixing Ni2+, Fe3+ and Co2+ precursors as well as Ti3C2Tx in a reverse microemulsion system,44,51 in which N,N-dimethyltetradecylamine and n-butanol were mixed with a volume ratio of 8
:
5. The surface-terminated groups (i.e. –(OH)x and –Fx) of Ti3C2Tx nanosheets might absorb Ni2+, Fe3+, and Co2+ ions via the electrostatic interaction. After the mixture was heated at 120 °C in an autoclave for 12 h, NiFeCo-LDH nanoplates together with TiO2 nanoparticles were formed on the surfaces of Ti3C2Tx nanosheets (Fig. 2a–c). Importantly, different from the NiFeCo-LDH nanoplates synthesized directly in solution and deposited randomly on a surface (e.g. on a copper grid) as shown in Fig. S3,† the NiFeCo-LDH nanoplates tend to stand up on the surfaces of Ti3C2Tx with edges largely exposed. Differently, TiO2 nanoparticles show spindle-like morphology (Fig. 2c). Our control experiment result indicated that Ti3C2Tx nanosheets which underwent a similar solvothermal treatment also showed surface deposited TiO2 nanospindles (Fig. S4†). It has been reported previously that surface defects might form on Ti3C2Tx nanosheets as a result of HF treatment, which provided preferential nucleation sites and Ti source for the growth of TiO2 in presence of air at elevated temperatures.41,52,53 Moreover, EDX mapping of a typical hybrid material in Fig. 2d shows the uniform distribution of Ti, Ni, Co, Fe and O elements, further indicating the successful preparation of the composite. The EDX spectrum in Fig. S5a† shows that the atomic ratio of Ni
:
Fe
:
Co in LDH was about 3
:
1
:
0.5, and Cl element was also detected. In addition, from the FT-IR spectrum of LDH (Fig. S5b†), a strong vibration of CO32− at ∼1377 cm−1 was observed.56,57 These imply that both Cl− and CO32− ions may act as the charge-balancing anions in the LDH nanoplates. The crystal structure of the composite was analyzed by XRD and compared with pristine Ti3C2Tx and LDH (Fig. 2e). The observed diffraction peak at 11.1° can be assigned to the (003) planes of NiFeCo-LDH (JCPDS no. 51-0463) based on previous reports as well as our control experiment (Fig. S6†).54,55 The peak present at 25.2° can be assigned to the (101) planes of TiO2 with the anatase phase (JCPDS no. 21-1272).58,59 It is interesting to note that the peak for the (002) planes of Ti3C2Tx shifts from 8.6° to 6.3° after hybridization, indicating an increased interlayer spacing (from 1 nm to 1.4 nm). This can be attributed to the intercalation of metal ions and surface oxidation of Ti3C2Tx during the solvothermal process. Selected area electron diffraction (SAED) and high resolution transmission electron microscopy (HRTEM) characterization were further applied to study the microstructure of the composite. As shown in Fig. 2f, the SAED pattern on a typical hybrid material lying flatly on a copper grid clearly shows the (100) and (110) spots for Ti3C2Tx with the six-fold symmetry. Besides, discontinued rings for (200) TiO2, (220) TiO2, (006) LDH and (009) LDH planes are also observed. Fig. 2g shows a typical square lattice pattern of anatase TiO2 along the [001] zone axis, with a lattice spacing of 3.5 Å for (101) planes. On the same image, a folded Ti3C2Tx could also be observed which shows enlarged interlayer spacing of ∼1.4 nm, consistent with the XRD peak at ∼6.3° (Fig. 2e). A side-view HRTEM image of NiFeCo-LDH nanoplates reveals a lattice spacing of 2.5 Å for the (009) planes as shown in Fig. 2h. From this image, the thickness of the LDH nanoplates can be estimated to be ∼3 nm.
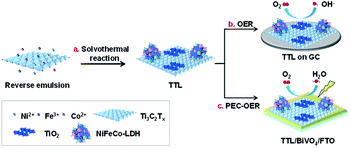 |
| Scheme 1 Illustration of the preparation of TTL for electrochemical and photoelectrochemical oxygen evolution reactions. | |
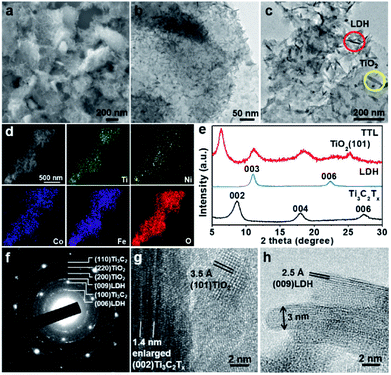 |
| Fig. 2 (a) SEM image of TTL. (b), (c) TEM images of the TTL. Inset: The LDH nanosheets (red circle) and TiO2 nanospindles (yellow circle). (d) STEM image and EDX mapping of TTL. (e) XRD patterns of TTL, LDH and Ti3C2Tx nanosheets. (f) Selected area electron diffraction of TTL. (g) HRTEM image of TiO2 and folded Ti3C2Tx edge. (h) A side-view HRTEM image of LDH nanoplates. | |
The composition and oxidization states of the hybrid material were further analyzed with XPS and shown in Fig. 3. The Ti 2p spectrum (Fig. 3a) shows a doublet at binding energies of 458.3 eV and 464 eV, which can be assigned to TiO2. The other peak at 455.2 eV is attributable to C–Ti–Tx (T is O, OH or F).60–62 It is important to note that compared with the Ti 2p spectrum of Ti3C2Tx nanosheets before hybridization (Fig. S1a†), the amount of oxide greatly increased after the solvothermal process. The Ni 2p spectrum shows two sets of doublets for Ni2+ (855.4 eV and 872.8 eV) and Ni3+ (857 eV and 874.5 eV), along with two satellite peaks (denoted as Sat.) at 861.5 eV and 879.4 eV, respectively (Fig. 3b).63–65 For the Fe 2p spectrum, the binding energies of 712.4 eV and 724.6 eV are corresponding to Fe 2p3/2 and Fe 2p1/2 bands of Fe3+, respectively (Fig. 3c).66 Similarly, the deconvolution of the Co 2p spectrum suggests the presence of Co2+ (782.5 eV and 797.8 eV) and Co3+ (780.6 eV and 796.4 eV) species, along with two satellite peaks at 786.1 eV and 804 eV, respectively (Fig. 3d).63,65 As a result, Co and Ni exist as multiple valence state and Fe provides +3 species in the hybrid material which are consistent with the pure LDH (Fig. S7†). Based on the XPS and ICP-OES results, the concentrations of the various components in TTL were calculated as shown in Tables S1–S3.†
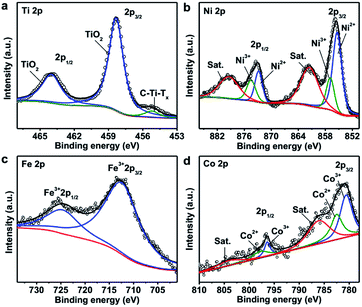 |
| Fig. 3 XPS (a) Ti 2p, (b) Ni 2p, (c) Fe 2p and (d) Co 2p spectra of TTL. | |
3.2 Electrochemical measurements
We first examined the electrocatalytic activity of the TTL composite toward OER in O2-saturated 0.1 M KOH solution. For comparison, the performance of NiFeCo-LDH, Ti3C2Tx, and commercial IrO2 towards OER was also tested on the basis of equal loading of active catalysts (i.e. 0.21 mg cm−2). Fig. 4a shows the linear-sweep voltammetry (LSV) measurements of the various catalysts deposited on glassy carbon (GC) electrode. The TTL modified electrode showed an oxidation peak at 1.4–1.5 V vs. RHE, which can be assigned to the Ni2+/3+ to Ni3+/4+ as well as the Co2+/3+ to Co3+/4+ redox processes,67,68 consistent with their corresponding cyclic voltammetry (CV) curves (Fig. S8†). Above this potential, current density rises sharply with O2 evolution.67,69–71 In addition, the TTL hybrid catalyst achieved a current density of 10 mA cm−2 at a potential of 1.55 V vs. RHE, which outperforms the state-of-the-art IrO2 catalyst (1.67 V vs. RHE) and is comparable among the currently best OER catalysts under the same measurement conditions (Table S4†). Furthermore, the Tafel slope for the TTL composite catalyst (98.4 mV dec−1) was lower than that of NiFeCo-LDH (114.8 mV dec−1), IrO2 (120.9 mV dec−1), and Ti3C2Tx (230.8 mV dec−1) (Fig. 4b), suggesting a more favored OER kinetics of the TTL hybrid catalysts.
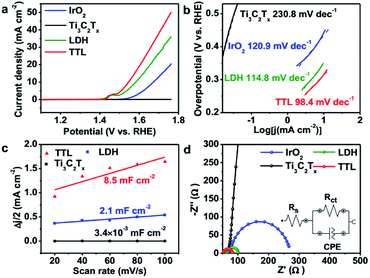 |
| Fig. 4 (a) LSV curves for electrodes modified by TTL, LDH, IrO2 and Ti3C2Tx for OER at the scan rate of 2 mV s−1. (b) The corresponding Tafel plots. (c) Current density difference at 1.438 V (vs. RHE) plotted against scan rate to give the double-layer capacitance (Cdl). (d) Nyquist plots for the various electrodes measured at a potential of 1.57 V (vs. RHE). The inset is the equivalent circuit. Rct is the charge transfer resistance, Rs is solution resistance, and CPE is the constant phase element. | |
The much enhanced performance of TTL catalyst compared to their individual components can be attributed to the synergistic coupling effect. The effective surface areas for the various electrocatalysts for OER were first analyzed based on the double-layer capacitance (Cdl), which was measured by CV with different scan rates over 1.411–1.464 V vs. RHE, that is, a potential range with no apparent faradaic processes (Fig. S9†). Fig. 4c shows the current density plotted against scan rate at a potential of 1.438 V vs. RHE, from which Cdl can be calculated. As expected, the as exfoliated Ti3C2Tx nanosheets showed a low Cdl of 3.4 × 10−3 mF cm−2, whereas NiFeCo-LDH nanoplates showed a slightly larger Cdl of 2.1 mF cm−2, and compared to the LDH, the TTL composite exhibited a more than 4 times increased Cdl of 8.5 mF cm−2, suggesting that the Ti3C2Tx nanosheets not only supported the NiFeCo-LDH nanoplates but also facilitated the easy access of aqueous electrolyte to the active surfaces of LDH nanoplates.28 Furthermore, the metal-like Ti3C2Tx nanosheets could enable fast charge/ion transport within the hybrid catalyst film, as indicated by the electrochemical impedance spectroscopy (EIS) measurement results (Fig. 4d). In addition, rotating ring-disk electrode (RRDE) measurement was carried out on the TTL-based electrode. A much smaller current was measured on the ring electrode compared to that on the disk electrode (Fig. S10†), suggesting a four-electron pathway for water oxidation (4OH− → O2 + 2H2O + 4e−) with negligible formation of peroxide intermediate.50,72 The Nyquist plots of the various electrodes were fitted by the RC circuit model as shown in the inset of Fig. 4d, which includes a solution resistance (Rs), a charge transfer resistance (Rct) and a constant phase component (CPE). The obtained Rct values are listed in Table S5†. As consistent with the LSV curves and Tafel slopes, the Rct of TTL is smaller than NiFeCo-LDH and IrO2, demonstrating a faster charge transfer during OER.
3.3 Photoelectrochemical measurements
Considering the fact that TiO2 is a typical semiconductor for light harvesting46,73 and transition metal LDHs have been known as promising co-catalysts or hole scavengers for PEC-OER,46,74 the simultaneous formation of TiO2 and NiFeCo-LDH on Ti3C2Tx is expected to generate an efficient hybrid catalyst for PEC-OER. Therefore, we used BiVO4 as a model PEC-OER catalyst to study the performance of TTL composite by casting it on pristine BiVO4/FTO as a photoanode. The PEC-OER tests were performed in 0.5 M potassium phosphate buffer (pH = 7). As shown in the photocurrent–potential curves in Fig. 5a, under 100 mW cm−2 illumination, the TTL/BiVO4 photoanode showed a much higher photocurrent density compared with the pristine BiVO4 over a potential window of 0.2 to 1.4 V (vs. RHE), and achieved a current density of 2.25 mA cm−2 at 1.23 V (vs. RHE), which is about 5 times higher than that of the pristine BiVO4 (0.39 mA cm−2). This also outperforms some previously reported LDH-based hybrids like N-deficient C3N4/N-doped graphene/NiFe-LDH hybrid and reduced titania@LDH hybrid.60,75 Additionally, the onset potential (potential at which photocurrent exceeds to 0.02 mA cm−2)76 shifted from 0.51 V for the pristine BiVO4 to 0.25 V vs. RHE, indicating the enhanced PEC-OER performance in TTL/BiVO4 photoanode. To further study the photoresponse of TTL/BiVO4 in comparison with pristine BiVO4, chronoamperometry measurements were carried out at 1.23 V (vs. RHE) under on–off illumination cycles (Fig. 5b). The current density of TTL/BiVO4 is at least 5 times higher than that of the pristine BiVO4, in addition to a prompt and steady response over consequent cycles. However, a spike was observed at the beginning of each illumination cycle, and quickly reduced to a steady state plateau, which can be attributed to a sudden generation of charge carriers and partial recombination.21,77 This can be due to the fact the TTL was drop-casted onto pristine BiVO4, which might result in poorer interface compared to those directly grown on BiVO4 as reported previously.33,43,78 To explore the charge transport properties of the PEC catalysts, EIS analysis was conducted at open circuit potential. From the obtained Nyquist plots in Fig. 5c, it can be seen that the semicircle for TTL/BiVO4 photoanode under illumination is smaller than that of the pristine BiVO4 photoanode, pointing to a much improved charge transfer between the anode and electrolyte, which is in line with the trend observed in Fig. 5a. Furthermore, the applied bias photon-to-current efficiency (ABPE) of pristine BiVO4 and TTL/BiVO4 photoanodes was plotted as a function of applied potential vs. RHE (Fig. 5d), which was derived from the LSV curves in Fig. 5a. It is obvious that the photoconversion efficiency of TTL/BiVO4 is much higher than that of the pristine BiVO4 photoanode over the bias window of 0.2–1.23 V vs. RHE. The maximum efficiency of TTL/BiVO4 is 0.5% (at 0.84 V vs. RHE) which is over 20 times higher than that of pristine BiVO4 photoanode (0.02% at 1.0 V vs. RHE). We further measured the incident photon-to-current efficiency (IPCE) of the various photoanodes at a constant applied potential of 1.23 V vs. RHE under monochromatic irradiation from 350 nm to 600 nm (Fig. S11†). All the hybrid photoanodes demonstrated much higher IPCEs than the pristine BiVO4 photoanode. The TTL/BiVO4 achieved the highest performance with a maximum efficiency of 44.6% at 380 nm. It is worth noting that the efficiency for both the Ti3C2Tx/TiO2/BiVO4 and TTL/BiVO4 photoanodes peaked at ∼380 nm, which agreed with the fact that TiO2 with a band-gap of ∼3.2 eV acted as a UV responsive photocatalyst.
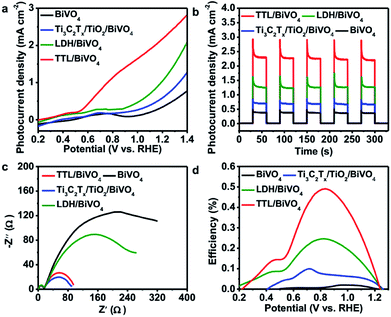 |
| Fig. 5 (a) Photocurrent–potential curves of pristine BiVO4 and TTL/BiVO4 photoanodes measured under 100 mW cm−2 illumination. (b) Chronoamperometry curves of BiVO4 and TTL/BiVO4 photoanodes performed under on–off illumination cycles at a potential of 1.23 V (vs. RHE). (c) EIS plots of BiVO4 and TTL/BiVO4 measured under illumination at open circuit potential over a frequency range from 100 kHz to 0.01 Hz. (d) Applied bias photo-to-current conversion efficiency (ABPE) curves of pristine BiVO4 and TTL/BiVO4 photoanodes derived from (a). All measurements were conducted in 0.5 M potassium phosphate solution (pH = 7). | |
The possible charge-transfer pathways in the TTL/BiVO4 photoanode during PEC-OER process was proposed as shown in Fig. 6. The much improved PEC performance of TTL/BiVO4 photoanode can be attributed to the following reasons. First, the anatase TiO2 nanoparticles with a wide bandgap of ∼3.2 eV absorbed light mostly in the UV region (Fig. S12†),25,43,79 which supplement the absorption of BiVO4 with a relatively narrow bandgap (∼2.4 eV).78–80 This was also evidenced by the IPCE results (Fig. S11†). Besides, LDHs have also shown weak semiconducting properties for light harvesting.21,23–25,79 Second, the conductive and hydrophilic Ti3C2Tx nanosheets might act as effective shuttles for electron/ion transport, which was also reflected in the EIS analysis results (Fig. 5c). Most importantly, considering the fact that the valence band levels of most CoFe or NiFe-based LDHs (about −5 to −6 eV)21,46,81 are higher than that of TiO2 (about −7.2 eV)44 and BiVO4 (about −7.1 eV)82,83 (all the band level are related to the vacuum level), holes generated in BiVO4 or TiO2 upon light irradiation could be effectively scavenged by the LDH nanoplates for the oxidation reactions of Ni2+/3+ to Ni3+/4+ as well as the Co2+/3+ to Co3+/4+ to take place.
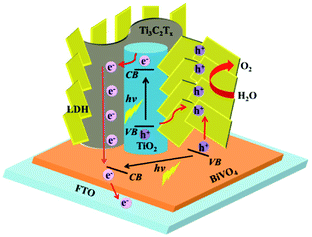 |
| Fig. 6 Schematic illustration of the proposed charge-transfer pathways in TTL/BiVO4 photoanode. | |
4. Conclusions
In summary, exfoliated Ti3C2Tx nanosheets were used as synthetic templates for the growth of NiFeCo-LDH nanoplates, and also provided Ti source for the formation of anatase TiO2 nanoparticles. The resulting composite showed excellent performance in OER. The NiFeCo-LDH nanoplates were mostly standing up on Ti3C2Tx with their ∼3 nm thick edges largely exposed, leading to a high active surface area for redox reactions. In addition, the Ti3C2Tx nanosheets were highly conductive and hydrophilic, allowing for the easy access of electrolyte and transport of electrons/ions. When the composite was further combined with the standard BiVO4 film, excellent performance in PEC-OER was also achieved. In addition to the high catalytic activity of NiFeCo-LDH nanoplates and conductivity of Ti3C2Tx, TiO2 nanoparticles which were uniformly distributed on Ti3C2Tx provided additional light-harvesting ability. We believe that high performance electrochemical and photoelectrochemical OER catalysts could be achieved by rational design and combination of dissimilar functional 2D materials.
Conflicts of interest
There are no conflicts to declare.
Acknowledgements
This research was supported by the National Natural Science Foundation of China (Grant No. 51322202 and No. 21671106).
References
- T. R. Cook, D. K. Dogutan, S. Y. Reece, Y. Surendranath, T. S. Teets and D. G. Nocera, Chem. Rev., 2010, 110, 6474–6502 CrossRef PubMed.
- I. Roger, M. A. Shipman and M. D. Symes, Nat. Rev. Chem., 2017, 1, 0003 CrossRef.
- A. Kumar, F. Ciucci, A. N. Morozovska, S. V. Kalinin and S. Jesse, Nat. Chem., 2011, 3, 707–713 CrossRef PubMed.
- S. Chen, J. Duan, M. Jaroniec and S. Z. Qiao, Adv. Mater., 2014, 26, 2925–2930 CrossRef PubMed.
- K. A. Stoerzinger, L. Qiao, M. D. Biegalski and Y. Shao-Horn, J. Phys. Chem. Lett., 2014, 5, 1636–1641 CrossRef PubMed.
- Y. Lee, J. Suntivich, K. J. May, E. E. Perry and Y. Shao-Horn, J. Phys. Chem. Lett., 2012, 3, 399–404 CrossRef PubMed.
- B. T. Yonemoto, G. S. Hutchings and F. Jiao, J. Am. Chem. Soc., 2014, 136, 8895–8898 CrossRef PubMed.
- H. Zhu, J. Zhang, R. Yanzhang, M. Du, Q. Wang, G. Gao, J. Wu, G. Wu, M. Zhang and B. Liu, Adv. Mater., 2015, 27, 4752–4759 CrossRef PubMed.
- F. Dionigi and P. Strasser, Adv. Energy Mater., 2016, 6, 1600621 CrossRef.
- Y. Pi, Q. Shao, P. Wang, F. Lv, S. Guo, J. Guo and X. Huang, Angew. Chem., Int. Ed., 2017, 56, 4502–4506 CrossRef PubMed.
- H. Wang, S. Zhuo, Y. Liang, X. Han and B. Zhang, Angew. Chem., Int. Ed., 2016, 55, 9055–9059 CrossRef PubMed.
- M. Gorlin, P. Chernev, J. F. De Araujo, T. Reier, S. Dresp, B. Paul, R. Krahnert, H. Dau and P. Strasser, J. Am. Chem. Soc., 2016, 138, 5603–5614 CrossRef PubMed.
- M. K. Bates, Q. Jia, H. Doan, W. Liang and S. Mukerjee, ACS Catal., 2015, 6, 155–161 CrossRef.
- Y. Wang, Y. Zhang, Z. Liu, X. Chao, S. Feng, D. Liu, M. Shao and S. Wang, Angew. Chem., Int. Ed., 2017, 56, 5867–5871 CrossRef PubMed.
- O. Diazmorales, I. Ledezmayanez, M. T. M. Koper and F. Callevallejo, ACS Catal., 2015, 5, 5380–5387 CrossRef.
- Q. Wang and D. O'Hare, Chem. Rev., 2012, 112, 4124–4155 CrossRef PubMed.
- G. Fan, F. Li, D. G. Evans and X. Duan, Chem. Soc. Rev., 2014, 43, 7040–7066 RSC.
- L. Qian, Z. Lu, T. Xu, X. Wu, Y. Tian, Y. Li, Z. Huo, X. Sun and X. Duan, Adv. Energy Mater., 2015, 5, 1500245 CrossRef.
- Z. Lu, L. Qian, Y. Tian, Y. Li, X. Sun and X. Duan, Chem. Commun., 2016, 52, 908–911 RSC.
- Z. Lu, L. Qian, W. Xu, Y. Tian, M. Jiang, Y. Li, X. Sun and X. Duan, Nano Res., 2016, 9, 3152–3161 CrossRef.
- S. M. Xu, H. Yan and M. Wei, J. Phys. Chem. C, 2017, 121, 2683–2695 Search PubMed.
- Y. Zhao, X. Jia, G. I. N. Waterhouse, L. Wu, C. Tung, D. Ohare and T. Zhang, Adv. Energy Mater., 2016, 6, 1501974 CrossRef.
- Y. Zhao, Y. Zhao, G. I. N. Waterhouse, L. Zheng, X. Cao, F. Teng, L. Z. Wu, C. H. Tung, D. O'Hare and T. Zhang, Adv. Mater., 2017, 29, 1703828 CrossRef PubMed.
- Y. Zhu, J. Ren, X. Yang, G. Chang, Y. Bu, G. Wei, W. Han and D. Yang, J. Mater. Chem. A, 2017, 5, 9952–9959 Search PubMed.
- Y. Dou, S. Zhang, T. Pan, S. Xu, A. Zhou, M. Pu, H. Yan, J. Han, M. Wei and D. G. Evans, Adv. Funct. Mater., 2015, 25, 2243–2249 CrossRef.
- D. H. Youn, Y. B. Park, J. Y. Kim, G. Magesh, Y. J. Jang and J. S. Lee, J. Power Sources, 2015, 294, 437–443 CrossRef.
- B. Li, Y. Zhao, S. Zhang, W. Gao and M. Wei, ACS Appl. Mater. Interfaces, 2013, 5, 10233–10239 Search PubMed.
- X. Long, J. Li, S. Xiao, K. Yan, Z. Wang, H. Chen and S. Yang, Angew. Chem., Int. Ed., 2014, 126, 7714–7718 CrossRef.
- M. Gong, Y. Li, H. Wang, Y. Liang, J. Z. Wu, J. Zhou, J. Wang, T. Regier, F. Wei and H. Dai, J. Am. Chem. Soc., 2013, 135, 8452–8455 CrossRef PubMed.
- M. Zhao, Q. Zhang, X. Jia, J. Huang, Y. Zhang and F. Wei, Adv. Funct. Mater., 2010, 20, 677–685 CrossRef.
- M. Naguib, V. Mochalin, M. W. Barsoum and Y. Gogotsi, Adv. Mater., 2014, 26, 992–1005 CrossRef PubMed.
- P. Simon, ACS Nano, 2017, 11, 2393–2396 CrossRef PubMed.
- Q. Tang, Z. Zhou and P. Shen, J. Am. Chem. Soc., 2014, 123, 16909–16916 Search PubMed.
- M. Naguib, M. Kurtoglu, V. Presser, J. Lu, J. J. Niu, M. Heon, L. Hultman, Y. Gogotsi and M. W. Barsoum, Adv. Mater., 2011, 23, 4248–4253 CrossRef PubMed.
- R. Zhao, M. Wang, D. Zhao, H. Li, C. Wang and L. Yin, ACS Energy Lett., 2018, 3, 132–140 CrossRef.
- Y. Wang, H. Dou, J. Wang, B. Ding, Y. Xu, Z. Chang and X. Hao, J. Power Sources, 2016, 327, 221–228 CrossRef.
- M. R. Lukatskaya, O. Mashtalir, C. E. Ren, Y. D. Agnese, P. Rozier, P. L. Taberna, M. Naguib, P. Simon, M. W. Barsoum and Y. Gogotsi, Science, 2013, 341, 1502–1505 CrossRef PubMed.
- O. Mashtalir, M. Naguib, V. Mochalin, Y. Dallagnese, M. Heon, M. W. Barsoum and Y. Gogotsi, Nat. Commun., 2013, 4, 1716 CrossRef PubMed.
- M. Ghidiu, J. Halim, S. Kota, D. Bish, Y. Gogotsi and M. W. Barsoum, Chem. Mater., 2016, 28, 3507–3514 CrossRef.
- C. J. Zhang, S. Pinilla, N. McEvoy, C. P. Cullen, B. Anasori, E. Long, S. H. Park, A. Seral-Ascaso, A. Shmeliov, D. Krishnan, C. Morant, X. Liu, G. S. Duesberg, Y. Gogotsi and V. Nicolosi, Chem. Mater., 2017, 29, 4848–4856 CrossRef.
- M. Naguib, O. Mashtalir, M. R. Lukatskaya, B. Dyatkin, C. Zhang, V. Presser, Y. Gogotsi and M. W. Barsoum, Chem. Commun., 2014, 50, 7420–7423 RSC.
- Y. Gao, L. Wang, A. Zhou, Z. Li, J. Chen, H. Bala, Q. Hu and X. Cao, Mater. Lett., 2015, 150, 62–64 CrossRef.
- Y. Hou, X. Li, Q. Zhao, X. Quan and G. Chen, Adv. Funct. Mater., 2010, 20, 2165–2174 CrossRef.
- X. Jia, Y. Zhao, G. Chen, L. Shang, R. Shi, X. Kang, G. I. N. Waterhouse, L. Wu, C. Tung and T. Zhang, Adv. Energy Mater., 2016, 6, 1502585 CrossRef.
- X. Li, X. Hao, A. Abudula and G. Guan, J. Mater. Chem., 2016, 4, 11973–12000 RSC.
- F. Ning, M. Shao, S. Xu, Y. Fu, R. Zhang, M. Wei, D. G. Evans and X. Duan, Energy Environ. Sci., 2016, 9, 2633–2643 Search PubMed.
- X. Zhang, R. Wang, F. Li, Z. An, M. Pu and X. Xiang, Ind. Eng. Chem. Res., 2017, 56, 10711–10719 CrossRef.
- L. Zhao, B. Dong, S. Li, L. Zhou, L. Lai, Z. Wang, S. Zhao, M. Han, K. Gao, M. Lu, X. Xie, B. Chen, Z. Liu, X. Wang, H. Zhang, H. Li, J. Liu, H. Zhang, X. Huang and W. Huang, ACS Nano, 2017, 11, 5800–5807 CrossRef PubMed.
- J. Halim, K. M. Cook, M. Naguib, P. Eklund, Y. Gogotsi, J. Rosen and M. W. Barsoum, Appl. Surf. Sci., 2016, 362, 406–417 CrossRef.
- T. Ma, J. L. Cao, M. Jaroniec and S. Z. Qiao, Angew. Chem., Int. Ed., 2016, 55, 1138–1142 CrossRef PubMed.
- G. Hu and D. Ohare, J. Am. Chem. Soc., 2015, 127, 17808–17813 CrossRef PubMed.
- Z. Li, L. Wang, D. Sun, Y. Zhang, B. Liu, Q. Hu and A. Zhou, Mater. Sci. Eng., B, 2015, 191, 33–40 CrossRef.
- O. Mashtalir, K. M. Cook, V. Mochalin, M. Crowe, M. W. Barsoum and Y. Gogotsi, J. Mater. Chem. A, 2014, 2, 14334–14338 Search PubMed.
- Z. Li, M. Shao, H. An, Z. Wang, S. Xu, M. Wei, D. G. Evans and X. Duan, Chem. Sci., 2015, 6, 6624–6631 RSC.
- H. Chen, L. Hu, M. Chen, Y. Yan and L. Wu, Adv. Funct. Mater., 2014, 24, 934–942 CrossRef.
- Z. Liu, R. Ma, M. Osada, N. Iyi, Y. Ebina, K. Takada and T. Sasaki, J. Am. Chem. Soc., 2006, 128, 4872–4880 CrossRef PubMed.
- C. J. Wang and D. O'Hare, J. Mater. Chem., 2012, 22, 21125–21130 RSC.
- F. Wang, C. Yang, M. Duan, Y. Tang and J. Zhu, Biosens. Bioelectron., 2015, 74, 1022–1028 CrossRef PubMed.
- J. Zhu, Y. Tang, C. Yang, F. Wang and M. Cao, J. Electrochem. Soc., 2016, 163, 785–791 CrossRef.
- J. Guo, C. Mao, R. Zhang, M. Shao, M. Wei and P. Feng, J. Mater. Chem. A, 2017, 5, 11016–11025 Search PubMed.
- S. Huang, L. Zhang, X. Lu, L. Liu, L. Liu, X. Sun, Y. Yin, S. Oswald, Z. Zou, F. Ding and O. G. Schmidt, ACS Nano, 2017, 11, 821–830 CrossRef PubMed.
- L. H. Karlsson, J. Birch, J. Halim, M. W. Barsoum and P. Persson, Nano Lett., 2015, 15, 4955–4960 CrossRef PubMed.
- J. Jiang, A. Zhang, L. Li and L. Ai, J. Power Sources, 2015, 278, 445–451 CrossRef.
- R. Zou, K. Xu, T. Wang, G. He, Q. Liu, X. Liu, Z. Zhang and J. Hu, J. Mater. Chem., 2013, 1, 8560–8566 RSC.
- Q. Yang, T. Li, Z. Lu, X. Sun and J. Liu, Nanoscale, 2014, 6, 11789–11794 RSC.
- L. Zhou, X. Huang, H. Chen, P. Jin, G. Li and X. Zou, Dalton Trans., 2015, 44, 11592–11600 RSC.
- S. Klaus, M. W. Louie, L. Trotochaud and A. T. Bell, J. Phys. Chem. C, 2015, 119, 18303–18316 Search PubMed.
- Y. P. Zhu, T. Ma, M. Jaroniec and S. Z. Qiao, Angew. Chem., Int. Ed., 2017, 56, 1324–1328 CrossRef PubMed.
- C. Wang, R. B. Moghaddam, M. J. Brett and S. H. Bergens, ACS Sustainable Chem. Eng., 2017, 5, 1106–1112 CrossRef.
- M. Bajdich, M. Garciamota, A. Vojvodic, J. K. Norskov and A. T. Bell, J. Am. Chem. Soc., 2013, 135, 13521–13530 CrossRef PubMed.
- D. A. Corrigan, J. Electrochem. Soc., 1987, 134, 377–384 CrossRef.
- S. Zhao, Y. Wang, J. Dong, C. T. He, H. Yin, P. An, K. Zhao, X. Zhang, C. Gao, L. Zhang, J. lv, J. Wang, J. Zhang, K. Abdul, N. Khan, Z. Wei, J. Zhang, S. Liu, H. Zhao and Z. Tang, Nat. Energy, 2016, 1, 16184 CrossRef.
- J. Schneider, M. Matsuoka, M. Takeuchi, J. Zhang, Y. Horiuchi, M. Anpo and D. W. Bahnemann, Chem. Rev., 2014, 114, 9919–9986 CrossRef PubMed.
- M. Shao, F. Ning, M. Wei, D. G. Evans and X. Duan, Adv. Funct. Mater., 2014, 24, 580–586 CrossRef.
- Y. Hou, Z. Wen, S. Cui, X. Feng and J. Chen, Nano Lett., 2016, 16, 2268–2277 CrossRef PubMed.
- T. Hisatomi, F. L. Formal, M. Cornuz, J. Brillet, N. Tetreault, K. Sivula and M. Gratzel, Energy Environ. Sci., 2011, 4, 2512–2515 Search PubMed.
- L. Zhang, E. Reisner and J. J. Baumberg, Energy Environ. Sci., 2014, 7, 1402–1408 Search PubMed.
- Y. Ma, S. R. Pendlebury, A. Reynal, F. L. Formal and J. R. Durrant, Chem. Sci., 2014, 5, 2964–2973 RSC.
- T. W. Kim and K. Choi, J. Phys. Chem. Lett., 2016, 7, 447–451 CrossRef PubMed.
- A. Iwase, S. Yoshino, T. Takayama, Y. H. Ng, R. Amal and A. Kudo, J. Am. Chem. Soc., 2016, 138, 10260–10264 CrossRef PubMed.
- G. Yilmaz, K. M. Yam, C. Zhang, H. J. Fan and G. W. Ho, Adv. Mater., 2017, 29, 1606814 CrossRef PubMed.
- Y. Tang, R. Wang, Y. Yang, D. Yan and X. Xiang, ACS Appl. Mater. Interfaces, 2016, 8, 19446–19455 Search PubMed.
- J. K. Cooper, S. Gul, F. M. Toma, L. Chen, P. A. Glans, J. Guo, J. W. Ager, J. Yano and I. D. Sharp, Chem. Mater., 2014, 26, 5365–5373 CrossRef.
Footnote |
† Electronic supplementary information (ESI) available: XPS of Ti3C2Tx nanosheets, TEM, XRD, EDX and XPS of NiFeCo-LDH nanoplates, TEM of Ti3C2Tx/TiO2, CV curves at different scan rates, RRDE measurement of TTL composite, PL and UV spectra of Ti3C2Tx/TiO2 and Ti3C2Tx. See DOI: 10.1039/c8ra02349b |
|
This journal is © The Royal Society of Chemistry 2018 |