DOI:
10.1039/C8RA01441H
(Paper)
RSC Adv., 2018,
8, 13364-13369
Effects of magnetic fields on the enzymatic synthesis of naringin palmitate
Received
14th February 2018
, Accepted 4th April 2018
First published on 10th April 2018
Abstract
The effects of magnetic fields on the enzymatic synthesis of naringin palmitate were studied. Both immobilized Candida Antarctica lipase B (I-CALB) and I-CALB tert-amyl alcohol solution were treated with magnetic fields of 100, 300, or 500 mT for 1, 2, or 3 h. Characteristics including the initial rate and the conversion yields after 24 h of reaction with magnetized I-CALB (M-I-CALB) and magnetized I-CALB tert-amyl alcohol solution (M-I-CALB-S) were investigated. Magnetic field application to both I-CALB and I-CALB-S influenced I-CALB activity. Enzyme activity increased for M-I-CALB and M-I-CALB-S with some intensities and durations and reached maxima at certain frequencies. Enzyme inactivation was only found with M-I-CALB exposed to a strong magnetic field (500 mT) for a long time (3 h). Unlike M-I-CALB, M-I-CALB-S exposed to a strong magnetic field for a long time (500 mT, 3 h) showed greater activity enhancement relative to I-CALB. Fourier transform infrared spectroscopy (FT-IR) results showed that the relative secondary structure content of free CALB was changed only slightly by the differing magnetic field intensities and durations. These findings should prove valuable for using magnetic fields in enzymatic reactions.
Introduction
Naringin is a flavanone glycoside primarily found in citrus fruits and exhibits a wide range of biological effects, including antioxidant, anti-inflammatory, and antibacterial activities.1,2 However, the practical application of naringin is strongly limited by its poor solubility and stability in lipidic media.3,4 An effective method to modify the lipophilicity of naringin is to introduce aliphatic groups into the naringin structure via either chemical or enzymatic esterification.5 Enzymatic esterification in organic solvents appears to be preferable for naringin acylation due to the high regioselectivity of enzymes and mild reaction conditions,5,6 whereas chemical methods suffer from inferior selectivity and more harsh reaction conditions.7 Some naringin esters such as naringin lauroyl ester, naringin octanoic acid ester, naringin decanoic acid ester and naringin palmitate have previously been synthesized through lipase-catalyzed acylation in organic solvents.3,8–10
However, the practical application of enzymatic methods is limited by low conversion yield.11,12 An external field such as a magnetic field may be used to improve enzymatic naringin derivatization in organic solvents. The influence of magnetic fields on enzymes has been extensively studied in recent years. Magnetic fields increase the activity of enzymes, such as immobilized α-amylase,13 horseradish peroxidase,14 cellulose,15 immobilized lipase, and chitinase,16 and decrease the activity of immobilized peroxidase.17 However, to date, previous investigations of the effects of magnetic fields on enzymatic reactions have mainly focused on aqueous systems, whereas little work has been performed on reactions in organic solvents using I-CALB. Furthermore, the differences in the enzymatic reactions between magnetized enzyme and magnetized enzyme solution (enzyme in solvent) are unclear. Therefore, it is necessary and important to study the effects of magnetic fields on enzymatic naringin derivatization in organic solvents and the differences in the enzymatic reactions between magnetized enzymes and magnetized enzyme solutions.
The aim of this study was to investigate the enzymatic transesterification of naringin with fatty acids in the presence of a magnetic field and to explore the mechanisms by which the magnetic field influences enzyme activity. This approach will provide theoretical guidance for practical applications. Palmitic acid was chosen as the acyl donor because it is commonly present in animals and plants and widely used in esterification processes. A sequential experimental strategy was used to evaluate the effects of naringin concentration, magnetic field intensity, and exposure time on the reaction conversion yields and enzyme structure. To the best of our knowledge, a systematic study to accelerate the enzymatic esterification of naringin with palmitic acid using magnetic pretreatment has not been reported.
Experimental
Materials
Immobilized lipase Novozyme 435 (immobilized Candida Antarctica lipase B, I-CALB) was purchased from Sigma (Germany). Naringin was acquired from Fluka (Switzerland). Palmitic acid (Fluka, Switzerland) and tert-amyl alcohol (Merck, Germany) were used as the acyl donor and solvent, respectively, during esterification. Molecular sieves of 4 Å (8–12 mesh beads) were purchased from Chemical Reagent Factory (Shanghai, China). All other reagents were of analytical grade.
Application of static magnetic fields
The static magnetic field apparatus was designed in our laboratory (Fig. 1). The maximum effective current was 12.5 A, the diameter of the iron core was 100 mm, the sectional area of the magnetic pole was 78.5 cm2, the total resistance of the coils was 8.8 Ω, and the power was 1.4 kW.
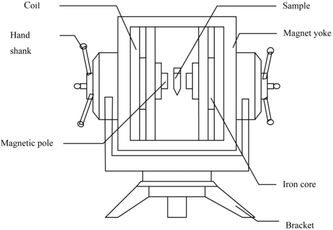 |
| Fig. 1 Schematic of the static magnetic field apparatus. | |
The magnetized I-CALB (M-I-CALB) (0.05 g) and the magnetized I-CALB tert-amyl alcohol solution (M-I-CALB-S) (0.05 g I-CALB in 5 ml tert-amyl alcohol) were generated by placing I-CALB and I-CALB solution in the center of the magnetic field apparatus (Fig. 1) with different magnetic intensities (100, 300, 500 mT) for various exposure times (1, 2, 3 h) at 25 °C. The magnetic intensity was measured with a CTS24 magnetometer from Zhenghong Electromagnetic Technology Company (Shanghai, China).
Enzymatic synthesis of naringin palmitate
The enzymatic synthesis of naringin palmitate in organic solvent was performed using the method of Zhang et al.10 Naringin (50 mM) and palmitic acid (250 mM) were mixed in M-I-CALB in tert-amyl alcohol solution or in M-I-CALB-S, which were each supplemented with molecular sieves (100 g L−1). Acylation with non-magnetized I-CALB and non-magnetized I-CALB solution was performed as the control. The concentrations of naringin and naringin palmitate were measured using HPLC as described by Zhang et al.3,10 All the experiments were performed in triplicate.
Conversion yield and initial reaction rate measurement
To characterize the effects of magnetic fields on the enzymatic synthesis of naringin palmitate, the initial reaction rates (v) and conversion yield (y, %) of the reaction were calculated using eqn (1) and (2): |
 | (1) |
|
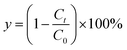 | (2) |
where v (mmol L−1 h−1) is the initial reaction rate of the reaction when the conversion yield is less than 10%, representing the enzyme activity; t1 (h) is the initial reaction time; Ct1 (mmol L−1) and Ct (mmol L−1) are the concentrations of naringin after t1 h and t h of incubation; y is the conversion yield of naringin palmitate synthesis after t h (≤24 h) of incubation; and C0 (mmol L−1) is the concentration of naringin at the beginning of the reaction.
Fourier transform infrared spectroscopy (FT-IR)
To investigate the effects of magnetic fields on the structure of the immobilized enzyme, free CALB (0.01 g) was sealed in a small test tube and fixed in the center of the magnetic field (100, 300, or 500 mT) for 1, 2, or 3 h. The IR spectra of magnetized CALB between 1215 and 1335 cm−1, which corresponds to the amide III region of the protein, were acquired with a Spectrum 2000 FT-IR spectrometer (PE, America) using KBr and compared to those of free CALB without magnetic field treatment. Analyses were performed at room temperature. Data analysis was performed with Peakfit 4.11 (Systat, America).
Statistical analysis
Triplicate analyses (n = 3) were performed for each dependent variable. Analysis of variance (ANOVA) with Duncan's multiple range test was performed with IBM SPSS Statistics 19.0 (SPSS Inc., Chicago, IL, USA). Differences were considered significant at P < 0.05. Values shown in tables are the means of values from the triplicate analyses (standard deviations).
Results and discussion
Effects of M-I-CALB on initial rate and conversion yields after 24 h
For immobilized cells/enzymes, the magnetic field leads to the enhancement of mass transfer, increased substrate utilization and product formation.18,19 M-I-CALB was obtained by treating I-CALB with magnetic fields of 100, 300, or 500 mT for 1, 2, or 3 hours. M-I-CALB was subsequently used to catalyze the esterification of naringin with palmitic acid. The initial rate and the conversion yields after 24 h (conversion yield of naringin palmitate synthesis catalyzed by M-I-CALB after 24 h of incubation) of the enzymatic reaction were calculated and are shown in Fig. 2. This rate was affected by the magnetic field strength and exposure time and was quite different from the rate of catalysis by I-CALB. When a 100 mT magnetic field was applied, the initial rate was higher than that of the untreated control and increased with exposure time (P < 0.05). However, when exposed to a higher magnetic field strength (300 or 500 mT), changes in the initial rate of the reaction with respect to exposure time followed different trends (Fig. 2). In the presence of a 300 mT magnetic field and a 1 h exposure, the initial rate increased compared to that of the untreated control (P < 0.05), but the rate decreased upon longer exposure (2–3 h). The maximum initial rate was achieved with M-I-CALB treated with a 500 mT magnetic field for 2 h, which increased the rate by 20.8% compared to that of the untreated control. However, an initial rate lower than that of the control was observed for M-I-CALB treated with 300 mT or 500 mT magnetic fields for 3 h (P < 0.05), indicating that M-I-CALB activity decreases in the presence of stronger magnetic fields (300 and 500 mT) and longer exposure times (3 h). These findings indicated that an optimum exists for the greatest positive interaction between enzyme (I-CALB) activity and magnetic field strength and that the effects of the magnetic field on the enzyme (I-CALB) can be maintained for some time after treatment. Similar results were reported for immobilized peroxidase exposed to an electromagnetic field17 and for non-magnetic ultrafine colloidal particles exposed to magnetic fields.20 The variation in the initial rate in Fig. 1 did not show simple correlation with magnetic field strength (i.e., 300 and 500 mT), which may be due to the magnetic field inducing conformational changes in I-CALB15,21 and thus influencing the active site of the enzyme molecule; such influence might have a complex impact on the initial enzymatic reaction rate.
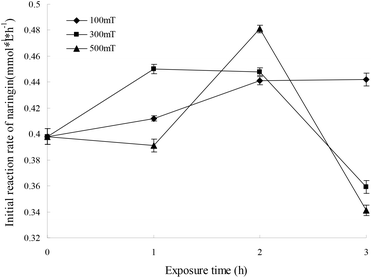 |
| Fig. 2 Effects of a magnetic field applied to I-CALB on initial reaction rate. | |
For a 100 mT magnetic field, there was no clear relationship between the initial rate and the conversion yield after 24 h; the conversion yields after 24 h decreased with exposure time (P < 0.05). As indicated previously, the initial rate increased in the presence of 300 mT and 500 mT magnetic fields, and changes in 24 h conversion yields with increased exposure time followed a similar trend as the changes in the initial rate. As shown in Fig. 3, the maximum conversion yield after 24 h (24.10%) was observed when a 500 mT magnetic field was applied for 2 h, which is a 29.2% increase compared to that of the control (18.66%). However, the 24 h conversion yields with M-I-CALB exposed to 300 mT and 500 mT magnetic fields for 3 h were much lower than those of the control (P < 0.05). These results illustrate a hysteresis effect for the application of a weak magnetic field (100 mT) to M-I-CALB, whereas more permanent effects were seen for M-I-CALB exposed to 300 mT and 500 mT magnetic fields.
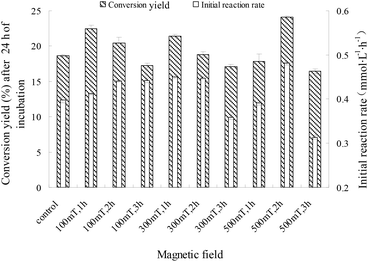 |
| Fig. 3 Conversion yields and initial rates of enzymatic reaction with M-I-CALB. | |
These findings indicated that I-CALB activity increased to a maximum level in the presence of magnetic fields of specific strength and duration, which is consistent with previous studies.13–16
Effects of M-I-CALB-S on the initial rate of enzymatic reaction
If an enzyme in an organic solvent is treated with a magnetic field, the conformation of the enzyme and the properties of the organic solvent will be influenced, which can affect the enzyme activity. The effects of magnetic fields on I-CALB in tert-amyl alcohol were investigated, and the initial rate as a function of magnetic field strength (100, 300, 500 mT) and exposure duration (1, 2, and 3 h) is shown in Fig. 4. The initial rate increased with increased exposure time and was higher than that of the unexposed control (P < 0.05), indicating enhanced I-CALB activity in tert-amyl alcohol. The polarizability and the dipole moment of chloroform in a 100 mT magnetic field has been reported to fluctuate in a wave-like manner as magnetic field strength increases,22 and the surface tension of pentane, hexane, heptane, n-amyl alcohol, hexyl alcohol, and heptanol exposed to magnetic fields (0.05–0.28 T) for 1.5 h decreases to a certain extent.23 Therefore, under the experimental conditions, changes in the polarizability, dipole moment, and surface tension of tert-amyl alcohol are important causes of enhanced I-CALB activity.
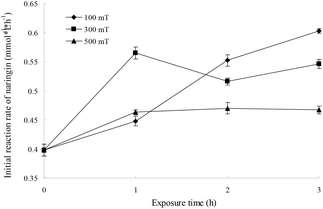 |
| Fig. 4 Effects of magnetic field application to I-CALB-S on initial reaction rate. | |
When M-I-CALB-S is used as shown in Fig. 4, with increased exposure time, the initial rate significantly increased for the 100 mT magnetic field (P < 0.05), fluctuated with the 300 mT magnetic field, and increased slightly when the magnetic field strength was 500 mT. In addition, with increasing magnetic field strength, the initial rate increased (100, 300 mT) and then decreased (500 mT) for a 1 h magnetic treatment and decreased when the exposure time increased to 2 or 3 h. These results suggested that the lower magnetic field strength (100 mT) and longer exposure time (3 h) was more favorable for improving I-CALB activity in tert-amyl alcohol, and the maximum initial rate (0.603 mmol L−1 h−1, increased by 51.5%) was obtained when M-I-CALB-S was treated with a 100 mT magnetic field for 3 h.
As shown in Fig. 2 and 4, the initial rate of the enzymatic reaction with M-I-CALB-S was higher than that with M-I-CALB, revealing that solvent exposure to a magnetic field was more effective than enzyme exposure for improving I-CALB activity. In addition, there was no enzyme inactivation in M-I-CALB-S, whereas enzyme inactivation was observed when I-CALB was exposed to a strong magnetic field for a long duration. Similar results were obtained by Wei et al.24 when a urease solution, urease, and solvent were treated with 100 mT and 205 mT magnetic fields. The urease activity in magnetized urease solution was significantly increased compared to that of the control, whereas the activity of urease treated with a magnetic field directly decreased.24 Thus, the treatment of I-CALB solution with a magnetic field is better for improving I-CALB activity.
Comparison of the effects of M-I-CALB and M-I-CALB-S on 24 h conversion yields
Due to the different initial rates of reactions with M-I-CALB and M-I-CALB-S, it is necessary to investigate the differences in conversion yields. According to the results above, the conversion yields within 24 h using the unexposed control, M-I-CALB, and M-I-CALB-S were calculated and are shown in Fig. 5.
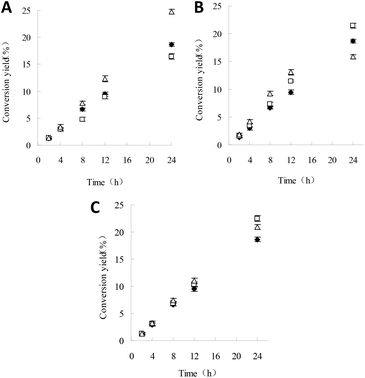 |
| Fig. 5 Effects of M-I-CALB and M-I-CALB-S on conversion yields within 24 h of reaction (A) 100 mT and 1 h; (B) 300 mT and 1 h; (C) 500 mT and 3 h. (◆, □, and △ represent control, M-I-CALB, and M-I-CALB-S, respectively). | |
When a 100 mT magnetic field is applied for 1 h, the conversion yields within 24 h for the reaction containing M-I-CALB increased with reaction time and were higher than those for the reaction with M-I-CALB-S; the yields were both higher than those of the control (P < 0.05) (Fig. 5). However, opposite trends were observed when a 500 mT magnetic field was applied for 3 h; the conversion yields within 24 h of the reaction containing M-I-CALB-S increased significantly (P < 0.05) and were higher than those of the control, whereas the yields for reactions containing M-I-CALB were lower than those of the control. When a 300 mT magnetic field was applied for 1 h, the conversion yields within 24 h of the reaction containing M-I-CALB increased with reaction time and were higher than those of the control, whereas those of the reaction containing M-I-CALB-S increased in the initial stage of the reaction (≤12 h) and then decreased (24 h) to lower than those of the control, which suggested magnetic hysteresis in M-I-CALB-S. In addition, the maximum conversion yield within 24 h of reaction was acquired with M-I-CALB-S treated by a 500 mT magnetic field for 3 h. These results indicated that treatment of I-CALB solution with a strong magnetic field (500 mT) and long exposure (3 h) was more effective for improving the conversion yield of naringin palmitate in tert-amyl alcohol.
Compared to M-I-CALB, the most favorable magnetic condition for M-I-CALB-S with regard to 24 h conversion yield only differs in exposure time. The exact mechanism involved is not clear, but most likely the altered conformation and flexibility of the enzyme due to the organic solvent and longer exposure time contributes to this discrepancy. To verify this hypothesis, the conformation of free CALB subjected to magnetic fields was assessed.
Effects of magnetic fields on conformation of free CALB
The amide III region (1215–1335 cm−1) was used to analyze the conformational changes of free CALB induced by magnetic fields. The percentages of α-helix (1288 to 1328 cm−1), β-turn (1270 to 1288 cm−1), random coil (1250 to 1270 cm−1), and β-sheet (1220 to 1240 cm−1) structure in free CALB were calculated from the area of the peaks and are listed in Table 1.
Table 1 Secondary structure percentages of free CALB determined by FT-IR
Magnetic conditions |
α-Helix (%) |
β-Sheet (%) |
β-Turn (%) |
Random coil (%) |
Control |
35.5 ± 0.4 |
22.0 ± 0.2 |
15.5 ± 0.4 |
26.9 ± 0.5 |
100 mT, 1 h |
35.1 ± 0.2 |
21.4 ± 0.1 |
16.2 ± 0.3 |
27.2 ± 0.2 |
100 mT, 2 h |
36.5 ± 0.5 |
21.4 ± 0.4 |
15.9 ± 0.6 |
26.2 ± 0.3 |
100 mT, 3 h |
35.2 ± 0.1 |
21.4 ± 0.3 |
16.2 ± 0.2 |
27.2 ± 0.4 |
300 mT, 1 h |
34.5 ± 0.6 |
22.3 ± 0.2 |
15.9 ± 0.1 |
27.3 ± 0.2 |
300 mT, 2 h |
34.6 ± 0.4 |
23.3 ± 0.5 |
15.3 ± 0.3 |
26.8 ± 0.2 |
300 mT, 3 h |
34.7 ± 0.2 |
22.5 ± 0.1 |
15.7 ± 0.4 |
27.1 ± 0.1 |
500 mT, 1 h |
36.5 ± 0.3 |
24.1 ± 0.2 |
14.2 ± 0.1 |
25.2 ± 0.2 |
500 mT, 2 h |
35.4 ± 0.1 |
22.4 ± 0.1 |
15.6 ± 0.3 |
26.7 ± 0.1 |
500 mT, 3 h |
35.2 ± 0.2 |
21.8 ± 0.5 |
16.0 ± 0.4 |
27.0 ± 0.3 |
As shown in Table 1, the α-helix content in the secondary structure of free CALB is 35.5%, which is followed in order by random coil, β-sheet, and β-turn; this composition is consistent with the relative percentages of secondary structure elements in free CALB determined by circular dichroism spectra.25
The relative α-helix, β-turn, random coil, and β-sheet content of magnetized free CALB changed slightly with the magnetic field strength and exposure time (P > 0.05), and there was no obvious correlation between the changes in secondary structure and the magnetic field strength or exposure time (Table 1). Compared with the secondary structure of the control free CALB, the differences in the relative proportions of α-helix, β-sheet, β-turn, and random coil structure in magnetized free CALB were maximally 1.0%, 2.1%, 1.3%, and 1.7%, respectively. It is known that the activities of enzymes are, in general, reduced but the stability is improved once enzymes are immobilized on particles.13 The structure of the immobilized enzyme may be further preserved.26 Due to the rigid carrier of I-CALB, thus, exposure to a magnetic field has little influence on the secondary structure of I-CALB. Watanabe et al.27 noted that the flexibility of an enzyme may be critical for its activity. There are two water molecules around the active site of CALB,28 which will affect flexibility of CALB.29 Therefore, the activation or inactivation of I-CALB by a magnetic field may result from variation in I-CALB flexibility due to the change of the water around the active site of CALB inducing by magnetic fields.
Many reports have shown no significant change in the conformation of CALB in various organic solvents.25,30 However, a relationship exists between the flexibility of CALB and the solvent.31,32 The flexibility of CALB is closely related to the solvent polarity and dielectric constant.30 Therefore, the flexibility of I-CALB in M-I-CALB-S may be altered by subtle changes in tert-amyl alcohol caused by the magnetic field, thus affecting the activity of I-CALB in tert-amyl alcohol.
Conclusions
In this study, the effects of a magnetic field on the enzymatic synthesis of naringin palmitate catalyzed by I-CALB were studied thoroughly under various magnetic conditions. The initial reaction rate and conversion yields of the reaction with M-I-CALB and M-I-CALB-S were compared. This work demonstrates that the I-CALB activity increased in a magnetic field and reached a maximum when the I-CALB solution was treated with a strong magnetic field (500 mT) for a longer time (3 h). The treatment of I-CALB solution with a strong magnetic field for a long exposure time is favorable for improving the activity of I-CALB. The changes in I-CALB activity resulting from a strong magnetic field with long exposure times are more durable than those resulting from a weak magnetic field and short exposure; a hysteresis effect appeared when the weak magnetic field was applied. FT-IR results show that the main secondary structure of CALB was α-helix (35.5%), which was followed in order by random coil, β-sheet, and β-turn; the relative secondary structure content of CALB changed slightly with magnetic intensity and exposure time. The changes in the secondary structure of CALB in a magnetic field were so slight that they are unlikely to explain the associated activity changes; instead, the activity differences may be due to changes in the flexibility of I-CALB and subtle changes to tert-amyl alcohol. These results are significant for improving the application of magnetic fields to enzymatic reactions.
Conflicts of interest
There are no conflicts to declare.
Acknowledgements
This work is supported by the National Key R&D Program of China (2016YFD0400203), the National Natural Science Foundation of China (No. 31401660), the Pearl River S&T Nova Program of Guangzhou (No. 201806010144) and the Fundamental Research Funds for the Central Universities, SCUT (No. 2017MS093 & 2015zp040).
References
- J. W. Lv, X. L. Sun, J. X. Ma, X. L. Ma, G. S. Xing, Y. Wang, L. Sun, J. B. Wang, F. B. Li and Y. J. Li, Biochem. Biophys. Res. Commun., 2015, 468, 587–593 CrossRef CAS PubMed.
- M. P. Salas, G. Céliz, H. Geronazzo, M. Daz and S. L. Resnik, Food Chem., 2011, 124, 1411–1415 CrossRef CAS.
- L. Cruz, M. Guimarães, P. Araújo, A. Évora, V. de Freitas and N. Mateus, J. Agric. Food Chem., 2017, 65, 6513–6518 CrossRef CAS PubMed.
- C. Ban, S. J. Park, S. Lim, S. J. Choi and Y. J. Choi, J. Agric. Food Chem., 2015, 63, 5266–5272 CrossRef CAS PubMed.
- S. H. Youn, H. J. Kim, T. H. Kim and C. S. Shin, J. Mol. Catal. B: Enzym., 2007, 46, 26–31 CrossRef CAS.
- X. Ma, R. Yan, S. Yu, Y. Lu, Z. Li and H. H. Lu, J. Agric. Food Chem., 2012, 60, 10844–10849 CrossRef CAS PubMed.
- P. G. Pietta, J. Nat. Prod., 2000, 63, 1035–1042 CrossRef CAS.
- H. Hattori, H. Tsutsuki, M. Nakazawa, M. Ueda, H. Ihara and T. Sakamoto, Biosci., Biotechnol., Biochem., 2016, 80, 1–7 CrossRef PubMed.
- A. Kontogianni, V. Skouridou, V. Sereti, H. Stamatis and F. Kolisis, J. Mol. Catal. B: Enzym., 2003, 21, 59–62 CrossRef CAS.
- X. Zhang, L. Li, Z. B. Xu, Z. L. Liang, J. Y. Su, J. R. Huang and B. Li, PLoS One, 2013, 8, e59106 CAS.
- M. H. Katsoura, A. C. Polydera, L. Tsironis, A. C. Polydera, L. Tsironis, A. D. Tselepis and H. Stamatis, J. Biotechnol., 2006, 123, 491–503 CrossRef CAS PubMed.
- B. M. Lue, Z. Guo, M. Glasius and X. B. Xu, J. Am. Oil Chem. Soc., 2010, 87, 55–61 CrossRef CAS.
- T. Mizuki, N. Watanabe, Y. Nagaoka, T. Fukushima, H. Morimoto, R. Usami and T. Maekawa, Biochem. Biophys. Res. Commun., 2010, 393, 779–782 CrossRef CAS PubMed.
- H. L. Ma, L. Huang and C. Zhu, J. Food Process Eng., 2011, 34, 1609–1622 CrossRef CAS.
- J. L. Zhang, S. J. Wang, B. G. Xu and M. X. Gao, J. Sci. Food Agric., 2012, 92, 1384–1388 CrossRef CAS PubMed.
- T. Mizuki, M. Sawai, Y. Nagaoka, H. Morimoto and T. Maekawa, PLoS One, 2013, 8, e66528 CAS.
- M. Portaccio, L. P. De, D. Durante, V. Grano, S. Rossi, U. Bencivenga, M. Lepore and D. G. Mita, Bioelectromagnetics, 2005, 26, 145–152 CrossRef CAS PubMed.
- Z. Al-Qodah, M. Al-Shannag, E. Assirey, W. Orfali, K. Bani-Melhem, K. Alananbeh and N. Bouqellah, Biochem. Eng. J., 2015, 97, 40–49 CrossRef CAS.
- Z. Al-Qodah, M. Al-Shannag, M. Al-Bosoul, I. Penchev, H. Al-Ahmadi and K. Al-Qodah, Rev. Chem. Eng., 2017, 5–19 Search PubMed.
- K. Higashitani, K. Okuhar and S. Hatade, J. Colloid Interface Sci., 1992, 152, 125–131 CrossRef CAS.
- Y. Liu, S. Jia, J. Ran and S. Wu, Catal. Commun., 2010, 11, 364–367 CrossRef CAS.
- G. F. Goya, T. S. Berquó, F. C. Fonseca and M. P. Morales, J. Appl. Phys., 2003, 94, 3520–3528 CrossRef CAS.
- H. Hu, H. Gao and S. Jia, J. Magn. Mater. Devices, 2000, 3, 36–41 Search PubMed.
- G. Wei, W. He, Y. Bi and M. Zhu, Acta Agric. Boreali-Occident. Sin., 1999, 2, 99–101 Search PubMed.
- R. W. Mccabe, A. Rodger and A. Taylor, Enzyme Microb. Technol., 2005, 36, 70–74 CrossRef CAS.
- M. S. M. Eldin, C. G. P. H. Schroën, A. E. M. Janssen, D. G. Mita and J. Tramper, J. Mol. Catal. B: Enzym., 2000, 10, 445–451 CrossRef.
- K. Watanabe, T. Yoshida and S. I. Ueji, Bioorg. Chem., 2004, 32, 504–515 CrossRef CAS PubMed.
- C. Li, T. W. Tan, H. Y. Zhang and W. Feng, J. Biol. Chem., 2010, 285, 28434–28441 CrossRef CAS PubMed.
- P. Trodler and J. Pleiss, BMC Struct. Biol., 2008, 8, 9–18 CrossRef PubMed.
- D. P. Teresa, J. Kirsi, I. Jarno, K. Jarno, D. Antonio and S. Carla, J. Food Eng., 2008, 87, 495–504 CrossRef.
- J. Mijović, Y. Bian, R. A. Gross and B. Chen, Macromolecules, 2005, 38, 10812–10819 CrossRef.
- J. Broos, A. J. W. G. Visser, J. F. J. Engbersen, W. Verboom, A. V. Hoek and D. N. Reinhoudt, J. Am. Oil Chem. Soc., 1995, 117, 12657–12663 CrossRef CAS.
|
This journal is © The Royal Society of Chemistry 2018 |
Click here to see how this site uses Cookies. View our privacy policy here.