DOI:
10.1039/C8RA00708J
(Paper)
RSC Adv., 2018,
8, 12588-12596
CO2–C evolution rate in an incubation study with straw input to soil managed by different tillage systems
Received
23rd January 2018
, Accepted 24th March 2018
First published on 3rd April 2018
Abstract
A laboratory incubation experiment was conducted to assess the impact of straw input on CO2–C emissions. After the winter wheat (Triticum aestivum L.) growing season, soil samples were collected from the 0–20 cm soil layer. The experiment was conducted on a brown loam soil, classified as a Udoll according to the U. S. soil taxonomy. Treatment levels consisted of three tillage practices: sub-soiling (ST), no-till (NT) and the conventional tillage (CT), two straw management (with and without input of straw), three temperature (25, 30 and 35 °C), and three moisture regimes (55%, 65% and 75% of field moisture capacity or FMC). The results showed that the rate of straw decomposition was the highest in the soil under NT management. The relationship between rate of cumulative CO2–C and straw decomposition was significant under NT (R2 = 0.52). The soil CO2–C release under incubation was significantly higher with than without the input of straw (R2 = 0.95). Furthermore, soil respiration increased with increases in incubation temperature and FMC. At 75% FMC, the rate of CO2–C release increased by 21.9 mg kg−1 d−1 at 30 °C and 32.0 mg kg−1 d−1 at 35 °C compared with that at 25 °C. At 35 °C, the rate of CO2–C release increased by 43.6 mg kg−1 d−1 at 65% FMC and 51.2 mg kg−1 d−1 at 75% FMC incubation than that of at 55% FMC under ST. The degree of control on the CO2–C evolution rate during the pre-incubation period and with higher incubation temperature and FMC was better for the soil from NT than that from CT and ST, and better yet for the soil from ST than that from CT.
1. Introduction
The North China Plain (NCP) is one of China's major agricultural areas (3.3 × 105 km2) with a population of approximately 130 million.1 Winter wheat (Triticum aestyum L.) and summer maize (Zea mays) are the predominant grain crops in the NCP. Maintaining increasing soil organic carbon (SOC) stock and reducing CO2–C emissions are essential for the long-term sustainability of agriculture in this area. CO2–C emissions from farmland soil is closely related to the straw decomposition rate.2,3 Therefore, the challenge lies in adopting management systems that would result in increasing SOC stock while reducing CO2–C emissions.
SOC is certainly the source of soil CO2–C emissions, but also the main existing C form in the farmland C bank. Thus, rational soil management is the key to enhance soil C sequestration.4,5 Tillage accelerates soil organic matter (SOM) oxidation by improving soil aeration, increasing the contact between soil and crop residues, and exposing aggregate-protected organic matter to microbial attack.6 Crop residues are an important source of potential depletion of SOC stocks and partially as the source of CO2–C emissions offset the environmental suitability.7 Different incubation temperatures and field moisture capacity (FMC) conditions may alter the soil C release rate under different tillage treatments.8 In general, gaseous fluxes are highly significantly (P < 0.01) or significantly (P < 0.05) related to temperature either at the soil surface or at 5 cm depth under both conventional tillage (CT) and no-till (NT) systems.9 The incubation temperatures and FMC might be crucial factors in diurnal variation of gaseous emissions under soil incubation.10,11
The nutrients released from straw decomposition play an important role in soil fertility. It is widely accepted that straw retention provides a good environment for enhancing microbial activity and SOC stock by improving soil physical and chemical properties.2,12 Therefore, the study of the effects of straw retention on soil fertility, soil respiration, and dynamic changes in soil quality are of great interest in addressing global issues. However, the results of incubation studies are strongly influenced by straw retention, soil temperature and soil moisture which are well documented as fundamental parameters in model simulations and predictions of soil respiration.13 Controlling the CO2–C emissions and using the straw effectively are a priority researchable themes. Modeling the influences of farmland soil with varying C-substrates, incubation temperatures, and soil moisture regimes can be useful in identifying the most appropriate strategies of tillage methods and management options. In addition, on-going research experiments are focused on the effects of straw retention with NT or CT on soil C sequestration but not on sub-soiling (ST).1,2,14,15 Thus, there are few, if any, reports available regarding the mechanisms of the evolution of CO2–C emissions from soil in relation to different organic substrates, incubation temperatures, and soil moisture conditions for different tillage methods.16
The present study was conducted to determine whether there is a best management practice (BMPs, i.e., rational combination of tillage management with straw input), that can be used to identify strategies or methods of mitigating CO2–C evolution. The present study is based on a 12 years ongoing soil management experiment involving different tillage systems in a wheat (Triticum aestivum L.)-maize (Zea mays) cropping system in NCP, China. The objective of this study was to understand how crop residues, incubation temperature, and soil moisture regime affect soil respiration under long-term management using diverse tillage systems.
2. Materials and methods
2.1. Experimental site
The field experiment was located at Tai'an (Northern China, 36°10′19′′ N, 117°9′03′′ E; altitude 134.0 m), which is a typical grain producing area in NCP. This experiment was established in a long-term tillage experiment site of Shandong Agricultural University. The average annual precipitation and temperature were 697 mm and 13.0 °C, respectively. The annual frost-free period was approximately 170–196 d and the annual sunlight duration was 2627 h. The soil is classified as Udoll according to the U. S. soil taxonomy,17 and it is a brown loam in texture. The soil textural composition consisted of 40% sand, 44% silt, and 16% clay. Some additional characteristics of the surface layer (0–20 cm) were as follows: pH = 6.8; soil bulk density = 1.43 g cm−3; SOM = 1.36%; soil total nitrogen = 0.13%; and soil total phosphorous = 0.13%.
2.2. Experimental design
The study was initialed in 2002, and consisted of three tillage practices: sub-soiling (ST), no-till (NT) and the conventional tillage (CT) as the control. The tillage treatments were laid out in a randomized block design (RBD) with three replications. The plot sizes for the main plots and subplots were 60 × 15 m and 15 × 15 m, respectively. The three tillage methods used in this experiment were implemented just before wheat (Triticum aestivum L.) was seeded. Maize was seeded directly in an un-ploughed seedbed. The CT treatment comprised of furrow ploughing to 25 cm depth, and ST of subsoiling to 40 cm depth. Both CT and ST plots were disked twice to 10 cm depth before seeding. Winter wheat (Jimai-22) was sown at a seed rate of 90 kg ha−1, around October 12, 2012 and was harvested on June 13, 2013. The basal fertilizer applied before sowing comprised of 225 kg N ha−1, 150 kg P2O5 ha−1, and 105 kg K2O ha−1. 100 kg N ha−1 was used as a topdressing during the jointing stage, along with 160 mm of supplemental irrigation. Maize (Zhengdan-958) was sown on June 18, 2013 at a rate of 66
600 plants ha−1 and was harvested on October 8, 2013. For maize, 120 kg N ha−1, 120 kg P2O5 ha−1, and 100 kg K2O ha−1 were used as a basal fertilizer, and 120 kg N ha−1 was used as topdressing during the jointing stage.
2.3. Sampling and culture design
Soil samples were collected after the wheat harvest in June 2013; each sample was a composite from five random positions in each plot from the 0–20 cm soil layer. Soil samples were air dried, gently ground and passed through a 2 mm sieve.
A three-factor-factorial experiment was established comprising of soil samples obtained from three tillage systems, three incubation temperatures, three moisture regimes, and two residue treatments either with or without straw input. Fifty grams of soil was mixed with the 0.67 g of winter-wheat straw powder. The three incubation temperatures used were 25 °C, 30 °C, and 35 °C, and the three soil moisture regimes were 55%, 65%, and 75% of the maximum field moisture capacity.18,19 The soil moisture content of the composite sample was determined using a Theta Probe soil moisture sensor (Delta T Devices, UK).
Fifty grams of dried soil from each plot was placed into a 50 ml polyvinyl tube (30 mm internal diameter) after mixing with 0.67 g winter-wheat straw powder (at a rate of 5 g C kg−1 soil). The control consisted of soil without the straw powder. The straw of winter wheat consisted of 507 g C and 18.5 g N kg−1 (C
:
N ratio of 27.4).
A soil-filled tube was gently tapped by hand against the wall, which resulted in a soil bulk density of approximately 1.2 g cm−3 (typical value of the field conditions). The water content in the soil was adjusted to 55%, 65%, and 75% of the soil water-holding capacity and was maintained by the method of Mahmood-ul-Hasan.18 Soil-filled tubes with open tops were immediately transferred to the incubator. Each soil-filled tube was then placed into an enclosed 280 cm3 jar containing a vial with 10 ml 0.5 M NaOH for trapping the respired CO2. These jars were incubated in the dark for up to 30 d under constant incubation temperatures and moisture regimes. The CO2-trapped vials were replaced with new vials every 3 d.
2.4. Measurements
The straw decomposition rate was determined based on a suppose that adding straw does not affect the original SOC mineralization. The decomposition rate was computed by comparing the SOC content in the soil with and without straw input using the following equation:20,21
Decomposition rate = (CO2–Ct − CO2–C0)/C × W × 100% |
where CO2–Ct is the amount of SOC released with straw input at time, and CO2–C0 is the amount of SOC released without straw: C is the SOC of straw and W is the straw dry matter.
The amount of CO2–C released from each tube was determined by titrating the residual NaOH solution with 0.5 M HCl in the presence of excess BaCl2 to a phenolphthalein end point.19 The release rate of CO2–C for different treatments was calculated as the function of incubation duration in days. Three blank jars without soil but with a vial of NaOH solution were also included as control during the incubation, period. The soil respiration rate was calculated as the difference in the amount of HCl consumed. The CO2–C evolution rate and cumulative CO2–C evolution of different treatments were calculated as the function of incubation duration in days.
2.5. Statistical analysis
In order to describe the correlation among soil respiration rate and the straw decomposition rate for different incubation temperatures, moistures and straw inputs, several multivariate statistical analyses were applied with a minimum number of parameters.6 The analysis consisted of three main steps. First, outliers were eliminated, because they did not follow a normal distribution when assessed using statistical tests. Second, the normality of residues was analyzed by using SPSS 19.0 statistical software and was classified by a cluster analysis to eliminate duplications of highly correlated properties. Third, a factor analysis was applied to obtain a small number of homoscedasticity (orthogonal properties). The figures were drawn using Sigma Plot 10.0 and Excel 2003. The standard deviation (SD) and least significant difference (LSD) were calculated to compare the treatment means. LSD was used to identify differences between treatments at P < 0.05.
3. Results
3.1. Percentage increase in CO2–C evolution with straw input
A significant interaction between tillage methods and straw input on soil respiration was observed throughout the incubation period (S1). Compared with the treatments without straw, input of straw significantly (P < 0.05) increased the soil respiration rate. In the treatment without straw input, the rate of soil CO2–C evolution peaked on the 6th day and remained at the steady state until the 24th day. (Fig. 1A). In comparison, the treatment with straw input reached the maximum CO2–C evolution on the 9th day and remained at the steady state rate until the 27th day of incubation (Fig. 1B). Regardless of the straw input, soil CO2–C evolution rate was the lowest under NT (Fig. 1A and B).
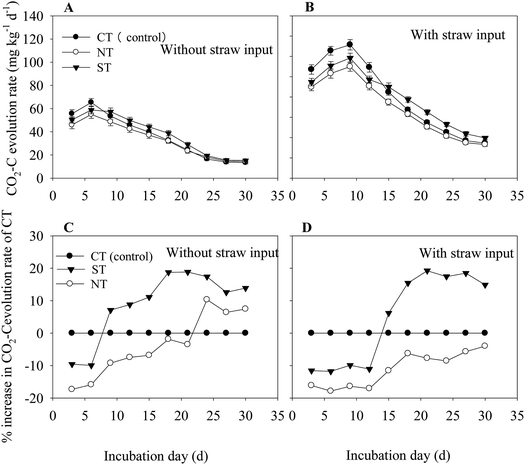 |
| Fig. 1 Rate of CO2–C evolution in soils under the input of with (A), and without (B) straw, and the percentage increase in CO2–C evolution under straw input compared with the treatment of without straw input (C) and with straw (D). Bars in (A)–(C) are ± standard errors of means (n = 3), which come from the standard deviation of systematic error. CT: conventional tillage; ST: sub-soiling; NT: no tillage. | |
ST reduced the CO2–C evolution rate in the first incubation period, and the CO2–C evolution rate gradually increased during the later incubation period, with or without the input of straw (Fig. 1C and D). However, NT increased the percentage of the CO2–C evolution rate without straw input during the late incubation period (Fig. 1C). As the soil C substrate was enhanced with the straw input, the process of soil respiration intensity was also enhanced. Soil from NT significantly reduced the percentage of CO2–C evolution rate (P < 0.01) at all the times, and that under ST significantly reduced the CO2–C evolution rate during the first 15 d. Meanwhile, the percentage of CO2–C evolution rate increased at the end of the incubation period and was approximately 14.9% of that under CT (Fig. 1D).
3.2. Straw decomposition rate
Within the same incubation temperature and FMC regime, the straw decomposition rate (Fig. 2) was the highest under NT followed by that under ST. Within the same incubation temperature, straw decomposition rate under NT peaked at 55% FMC. Within the same moisture regime, straw decomposition rate was the highest at 30 °C under CT and at 35 °C under ST. After incubation at 25 °C, straw decomposition rate under CT and ST were the highest under 75% FMC. The evolution of CO2–C was the highest under NT at 55% FMC. Within the condition of 25 °C and 55% FMC, the straw decomposition rate increased by 58.8% and 47.6% under NT compared with that under CT and ST, respectively.
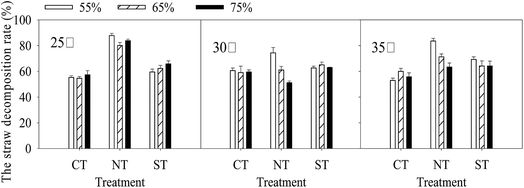 |
| Fig. 2 The straw decomposition rate after different incubation temperature and FMC. Bars represent standard error (n = 3), which come from the standard deviation of systematic error. CT: conventional tillage; ST: sub-soiling; NT: no tillage. | |
The correlation between the cumulative CO2–C evolution and straw decomposition rate varied under different treatments. A significant negative correlation between the cumulative CO2–C evolution rate and straw decomposition rate was observed under NT (P < 0.05). However, no significant relationship was observed between the cumulative CO2–C evolution rate and straw decomposition rate under CT and ST.
3.3. Soil CO2–C evolution rate under straw input
The rate of CO2–C release from the soil with the straw input differed among different incubation temperatures and moisture regimes. The overall trend was a reduction in the rate after the initial increase before attainment of the steady state. The CO2–C respiration rate was the highest under CT during the first 12 d. In addition, the CO2–C respiration rate was higher under ST than it was under CT, and it was the lowest under NT at all the times.
Regarding to the effects of environmental variables, the interactive effect of incubation temperature and moisture regime significantly affected the soil respiration rate throughout the incubation period in treatments with straw input (P < 0.01). The CO2–C respiration rate was the lowest under NT at all times. Compared with CT, the soil respiration rate under ST significantly increased in treatments with higher temperature and moisture regimes (P < 0.01). The higher the temperature and the moisture regime during incubation, the higher was the soil respiration rate (Fig. 3A–I).
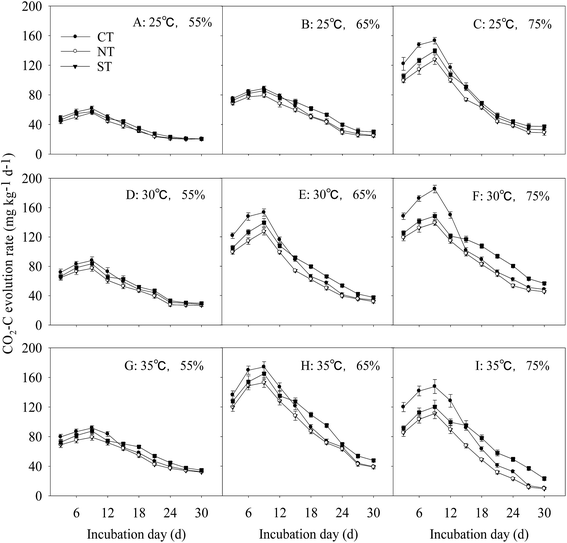 |
| Fig. 3 Rate of soils CO2–C evolution in different incubation temperatures and FMC under straw input. A to I: the soil treatments with different incubation temperatures and FMC. Bars in (A)–(I) are ± standard errors of means (n = 3), which come from the standard deviation of systematic error. CT: conventional tillage; ST: sub-soiling; NT: no tillage. | |
In soil with 65% and 75% FMC, the soil respiration rate significantly increased with the increase in incubation temperature. Under 65% FMC incubation, the soil respiration rate under ST was increased by 27.8% and 58.8% at incubation temperatures of 30 °C and 35 °C, respectively, incubation compared with that at 25 °C; the incremental increase in CO2–C evolution under ST was the lowest among the three treatments. Under the same incubation temperature but different moisture regimes, the soil respiration rate increased as FMC increased. At 35 °C, the respiration rate for soil from ST with FMC of 65% and 75% was increased by 37.9% and 82.5%, respectively, compared with that under 55% FMC during the latter part of incubation.
At 75% FMC and 35 °C, the peak time of the respiration rate for soil under ST exceeded 3–5 d, compared with that for 30 °C and 25 °C. Therefore, higher incubation temperatures and moisture regimes increased the soil respiration rate. On the contrary, soil under NT had a significantly lower CO2–C evolution rate than soil under CT and ST at all incubation time. However, the CO2–C evolution rate under ST was significantly lower than that under CT for the earlier incubation time under higher temperature and moisture regimes.
3.4. Percentage increase in soil cumulative CO2–C evolution with straw input
The cumulative CO2–C evolution, as affected by tillage, also changed with straw input and incubation time (Fig. 4A–D). Without straw input, the cumulative CO2–C evolution from soil under ST and NT was generally lower than that under CT during the first 15 d. Thereafter, the cumulative CO2–C evolution under ST gradually exceeded that under CT (Fig. 4A and C). The cumulative CO2–C evolution was significantly (P < 0.05) low under NT and was the lowest among the three treatments. With straw input, the cumulative CO2–C evolution in soil under ST and NT was significantly lower than that under CT throughout the incubation period, and the rate under NT was lower by 13.3% compared with that under CT by the end of the incubation period (Fig. 4B and D).
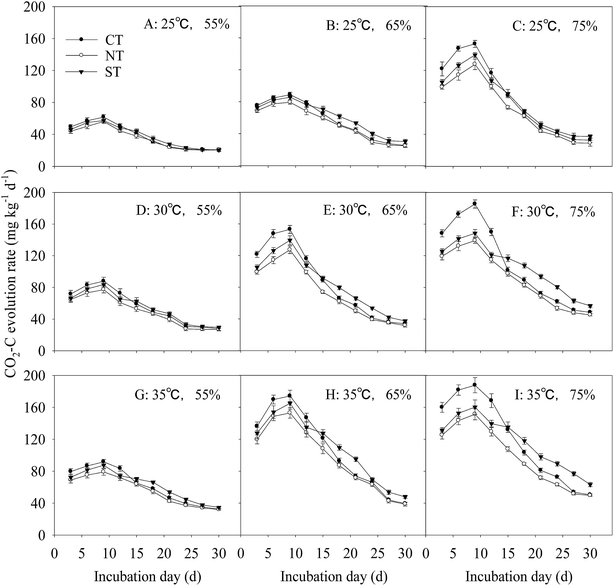 |
| Fig. 4 Cumulative CO2–C evolution in soils under different incubation temperatures and FMC. Bars in (A)–(I) are ± standard errors of means (n = 3), which come from the standard deviation of systematic error. CT: conventional tillage; ST: sub-soiling; NT: no tillage. | |
3.5. Soil cumulative CO2–C evolution under straw input
The cumulative CO2–C evolution, as affected by soil incubation temperature and moisture regimes, also changed with the incubation time (Fig. 5A–I). The cumulative CO2–C evolution was the lowest under NT at all times and was lower under ST than under CT during the early incubation period. As the incubation time increased, the cumulative CO2–C evolution changed with the change in incubation temperatures and moisture regimes.
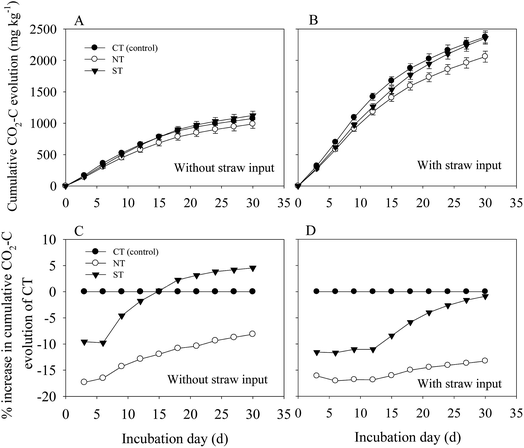 |
| Fig. 5 Cumulative CO2–C evolution in soils under the input of with and without straw, and the percentage increase in CO2–C evolution of with straw input compared with without straw input soil (control). Bars in (A)–(D) are ± standard errors of means (n = 3), which come from the standard deviation of systematic error. CT: conventional tillage; ST: sub-soiling; NT: no tillage. | |
For soil under the same FMC and different incubation temperatures, the cumulative CO2–C evolution increased as incubation temperature increased, revealing significant (P < 0.05) differences in 65% and 75% FMC. With 65% FMC, the cumulative CO2–C evolution was lower under ST and NT than that under CT during the first 21 d under 25 °C and 35 °C. At 30 °C, the cumulative CO2–C evolution was lower under ST and NT than that under CT at all times. At 75% FMC, the cumulative CO2–C evolution was significantly (P < 0.01) lower under ST and NT than that under CT. Incubating the soil under ST at 30 °C and 35 °C increased the cumulative CO2–C evolution by 30.2% and 43.8%, respectively, compared with that at 25 °C. The cumulative CO2–C evolution for soil under NT at 30 °C and 35 °C increased by 25.5% and 36.8%, respectively, than that at 25 °C. Under the same incubation temperature and different FMC, the cumulative CO2–C evolution increased as FMC increased.
Under the condition of 65% and 75% FMC, the cumulative CO2–C evolution increased significantly with the increase in incubation temperature. At 35 °C incubation, the cumulative CO2–C evolution for soil under ST at 65% and 75% FMC increased by 74.0% and 86.9%, respectively, compared with that at 55% FMC. At 35 °C incubation, cumulative CO2–C evolution for soil under NT at 65% and 75% FMC decreased by 71.8% and 75.7%, respectively, compared with that at 55% FMC. The incremental increase of cumulative CO2–C evolution for soil under CT was the greatest, and that under NT was the least among the three treatments. At the end of the incubation period, the cumulative CO2–C evolution for soil under ST and NT at 75% FMC was lower than that of the soil under CT by 2.2% and 17.6%, respectively.
4. Discussions
This study was conducted under laboratory conditions to better understand the mechanisms that control CO2–C evolution in relation to changes in straw input, temperature, and soil moisture for soil samples obtained from sites under different long-term tillage systems. The short-term incubation period could decrease the bias caused by the loss of soil structure under in situ field conditions.22,23
4.1. Tillage management systems and straw input effects on soil CO2–C evolution rate
A review of the available literature showed that straw input significantly increase soil respiration rate.24,25 However, the data presented in this study did not demonstrate a significant relationship between the soil CO2–C evolution rate and straw decomposition rate, except in soil under NT. This trend of negative correlations observed in this study can be attributed to the assessment of soil respiration using an incubation experiment for determining the rate of straw decomposition. Furthermore, an interaction was observed between tillage and straw input on the soil CO2–C evolution rate and straw decomposition rate.26 In another study, a 30-d micro system test also demonstrated the significant effects of different incubation temperatures, moisture regimes, and organic material added to incubation soil had effect on the respiration rate under different tillage methods.27,28
Similar results were reported from field studies conducted by Han et al.,2 indicating that ST and NT methods can reduce the soil CO2–C evolution rate compared with that by CT. This trend is attributed to the low level of soil disturbance under ST and NT compared with that under CT, and it leads to frequent disruption and breakdown of soil aggregates.11 Thus, the rate of soil CO2–C evolution is faster in soil under CT than that under the conversation tillage. Similar results were also reported who conducted the field studies.8,29 Furthermore, the reduction in the soil CO2–C evolution rate under tillage with incubation time suggests that the impact of BMPs can be identified even from a short-term laboratory incubation.
Several studies have reported that input of straw substrate increases the soil CO2–C evolution rate2,10 due to the effects of tillage on the soil microbial community8,25 and on soil desiccation.30 Microbial communities quickly proliferate with the start of incubation under favorable incubation temperature and moisture regimes.31 Thus, both the soil CO2–C evolution rate and cumulative CO2–C evolution increase significantly in the initial incubation period. A rapid proliferation of microbial communities might have subsequently allocated more substrates to their proliferation and growth than to respiration thereby decreasing the CO2–C evolution in soil with straw compared with those without straw input, especially during the earlier incubation period. Thereafter, the soil respiration rate and cumulative CO2–C evolution reached a steady state equilibrium.
Due to differences in the straw decomposition rate under different treatments, the effects of straw on the soil respiration rate under different treatments also varied. The straw decomposition rate was higher under NT and ST than under CT. These results differ from a research experiment conducted by Jiang32 which demonstrated that the input of maize straw under NT decomposed at a slower rate than that under other tillage method. However, straw retention can significantly increase soil CO2 release,33 as straw provides C substrates and increases soil porosity34 which accelerates the release of CO2–C. However, the process of C absorption and release by soil with the input of straw requires further research.
4.2. Temperature and moisture effects on soil CO2–C evolution rate
The relationship of the soil CO2–C evolution rate with temperature and moisture involves complex interactions dependent on the relative limitation of variable microbial activity and soil substrate supply. These interactions among environments, and thus the relationships may vary accordingly. Similar to the results of an infrared warming experiment35 and a cropland study,36 soil respiration increased as temperature increased in the present study. However, some reports showed that the soil CO2–C evolution rate might be decoupled from temperature under certain soil moisture levels, resulting in a soil CO2–C evolution rate that is unaffected by temperature under water stress. For example, Chen37 and Balogh et al.28 observed that the soil CO2–C evolution rate decreased with increasing soil moisture content. However, the results of the present study show that the soil CO2–C evolution rate increased as soil moisture content increased. The discrepancy between the aforementioned results and those of the present study might be due to differences in the examined soils regarding their chemical, physical, and microbial properties. The decrease in the soil CO2–C evolution rate at lower soil moisture has been attributed to soluble substrate limitation, whereas at higher soil moisture level—especially at saturation—the soil CO2–C evolution rate was primarily limited by oxygen. However, the high moisture and temperature incubation could be due to the persistently high microbial activity, as this was the optimal moisture level and high microbial activity might override the influence of substrate limitation.
In general, previous studies documented that the response of the soil CO2–C evolution rate to warming35 and moisture regimes36 is important for reducing the greenhouse effect and improving soil fertility.38 With increasing global warming and a rapidly falling groundwater table, understanding the response of soil and straw input to high incubation temperature and drought stress is critical. The effect of the farmland soil CO2–C evolution rate on soil fertility, crop production, and warming are major global issues. Our understanding of the soil CO2–C evolution rate still needs to be improved by examining different rates of straw input and a wider range of incubation temperatures and moisture regimes.
4.3. Variations in soil CO2–C evolution rate with incubation time
A laboratory incubation study by Li et al.20 demonstrated that the soil CO2–C evolution rate increased with incubation time, which was ascribed to a change in substrate quality from labile to recalcitrant. The data from the present study for relatively short-term incubation experiment show that the soil CO2–C evolution rate changes with incubation time, i.e., a temporal effect of soil tillage on soil C dynamics was observed. The present study has demonstrated that the effect of soil tillage on the soil CO2–C evolution rate increased with incubation time initially but declined during later incubation stages at different soil temperature and moisture regimes, as has also been shown by other studies.35 Over the 30 d incubation period, with 65% FMC, the cumulative CO2–C evolution was lower under ST and NT than under CT during the first 21 d under 25 °C and 35 °C. At 30 °C, cumulative CO2–C evolution was lower under ST and NT than under CT at all times. The underlying mechanisms were ascribed to substrate depletion:17 the longer the incubation time, the more time the microbes had to consume labile C, leaving less of it in the soil. This occurrence was likely due to more confounding factors and less incubation time involved in the present incubation experiment. At the end of the incubation period, cumulative CO2–C evolution for soil under ST and NT at 75% FMC was lower than that of soil under CT by 2.2% and 17.6%, respectively. The data presented herein showing that the effect of soil tillage changed with incubation time suggests a strong need for extrapolating the data on C dynamics from short term laboratory conditions to large scale field-studies.
5. Conclusions
The data from incubating a long-term tillage soil under two straw management, three temperature levels and three moisture regimes and measuring straw decomposition and soil CO2–C evolution rate and cumulative CO2–C evolution throughout the incubation showed that:
(1) Soil management by NT could increase the straw decomposition rate more than that by ST and CT due to differences in incubation temperatures and moisture regimes. During incubation, under ST the soil CO2–C evolution rate decreased at an earlier stage and increased during the later stage;
(2) Lower incubation temperature and moisture content decreased the CO2–C evolution rate and cumulative CO2–C evolution;
(3) Straw input significantly increased CO2–C evolution rate;
(4) Thus, the data obtained from the present study indicate that, under higher incubation temperatures and moisture regimes, ST and NT management can significantly control CO2 release compared with that by CT.
These results imply that the response of the CO2–C evolution rate to future global warming may be affected by changes in soil management practices. In NT and ST, global warming and high FMC may cause less soil CO2–C evolution, but relatively more CO2–C evolution may be emitted in CT. Considering that ST and NT management can significantly control CO2–C evolution, the rise in temperature and the high FMC would mean less soil CO2–C evolution to the atmosphere through BMPs in the future.
Conflicts of interest
There are no conflicts to declare.
Acknowledgements
This work was financially supported in part by the National Nature Science Foundation of China (No. 31471453; 31771737), and by the Special Fund for Agro-scientific Research in the Public Interest of China (No. 201503117).
References
- W. X. Dong, C. S. Hu, S. Y. Chen and Y. M. Zhang, Nutr. Cycling Agroecosyst., 2009, 83, 27–37 CrossRef CAS.
- H. F. Han, T. Y. Ning, Z. J. Li and H. M. Cao, Exp. Agr., 2017, 53, 118–130 CrossRef.
- Q. R. Liu, X. H. Liu, C. Y. Bian, C. J. Ma, K. Lang, H. F. Han and Q. Q. Li, Sci. World J., 2014, 7, 1–8 Search PubMed.
- R. Lal, Nutr. Cycling Agroecosyst., 2008, 81, 113–127 CrossRef.
- A. Legrand, C. Gaucherel, J. Baudry and J. M. Meynard, Agron. Sustainable Dev., 2011, 31, 515–524 CrossRef.
- L. J. Guo, Z. S. Zhang, D. D. Wang, C. F. Li and C. G. Cao, Biol. Fertil. Soils, 2014, 51, 65–75 CrossRef.
- F. Monforti, E. Lugato, V. Motola and J. F. Dallemand, Renewable Sustainable Energy Rev., 2015, 44, 519–529 CrossRef.
- E. Lellei, E. Kovacs, Z. Botta, T. Kalapos, B. Emmett and C. Beier, Eur. J. Soil Biol., 2011, 47, 247–255 CrossRef.
- S. Z. Tian, T. Y. Ning, S. Y. Chi, Y. Wang, B. W. Wang, H. F. Han, C. Q. Li and Z. J. Li, Acta Ecol. Sin., 2012, 32, 879–888 CrossRef CAS.
- S. R. Chen, J. Li, P. L. Lu, Y. H. Wang and Q. Yu, Chin. J. Ecol., 2004, 15, 1552–1560 Search PubMed.
- S. Z. Tian, Y. Wang, N. Li, T. Y. Ning, B. W. Wang and Z. J. Li, Soil Tillage Res., 2012, 121, 57–62 CrossRef.
- T. Kautz, C. López Fandob and F. Ellmer, Appl. Soil. Ecol., 2006, 33, 278–285 CrossRef.
- Y. Luo and X. Zhou, Soil respiration and the environment, Academic Press, an imprint of Elsevier, 2006, ISBN: 9780120887828 Search PubMed.
- Y. H. Wei, X. Zhao, Y. L. Zhai, E. P. Zhang, F. Chen and H. L. Zhang, Tran. CSAE, 2013, 29, 87–95 Search PubMed.
- X. W. Chen, N. Wang, X. H. Shi, X. P. Zhang, A. Z. Liang, S. X. Jia, R. Q. Fan and S. C. Wei, Acta Ecol. Sin., 2013, 33, 2676–2683 CrossRef.
- W. P. Zhou, D. F. Hui and W. J. Shen, PLoS One, 2014, 9, e92531 Search PubMed.
- Soil Survey Staff, Keys to Soil Taxonomy, 1998, 8th edn Search PubMed.
- M. Mahmood-ul-Hasan, Pak. J. Sci. Ind. Res., 2002, 45, 145–150 Search PubMed.
- X. G. Li, X. M. Shi, D. J. Wang and Z. Wei, Biol. Fertil. Soils, 2012, 48, 597–602 CrossRef CAS.
- Q. X. Wen, Soil Organic Matter Research, Agricultural Science Press, Beijing, 1984, pp. 285–295 Search PubMed.
- X. B. Zhang, M. G. Xu and W. J. Zhang, Sci. Agric. Sin., 2011, 24, 5013–5020 Search PubMed.
- A. Chatterjee and G. D. Jenerette, Geoderma, 2011, 163, 171–177 CrossRef CAS.
- E. Lellei, E. Kovacs, Z. Bot, T. Kalapos, B. Emtamett and C. Beier, Eur. J. Soil Biol., 2011, 47, 247–255 CrossRef.
- X. S. Li, N. Wu, L. Liu, Y. P. Feng, X. Xu, H. F. Han, T. Y. Ning and Z. J. Li, Chin. J. Ecol., 2015, 6, 1765–1771 Search PubMed.
- Y. Wang, H. J. Li, B. Han, Z. Q. Shi, T. Y. Ning and Z. J. Li, Acta Ecol. Sin., 2007, 27, 3384–3390 CAS.
- S. Z. Tian, T. Y. Ning, Y. Wang, H. J. Li, W. L. Zhong and Z. J. Li, Chin. J. Ecol., 2010, 21, 373–378 CAS.
- C. J. Hu, G. H. Liu, B. J. Fu, T. T. Jin, L. Guo and C. H. Su, Acta Ecol. Sin., 2009, 29, 4700–4709 CAS.
- J. Balogh, K. Pinter, S. Foti, D. Cserhalmi, M. Papp and Z. Nagy, Soil Biol. Biochem., 2011, 43, 1006–1013 CrossRef CAS.
- S. Muhammad, T. Müller and R. G. Joergensen, Biol. Fertil. Soils, 2006, 43, 93–101 CrossRef CAS.
- M. L. Kakumanu, C. L. Cantrell and M. A. Williams, Soil Biol. Biochem., 2013, 57, 644–653 CrossRef CAS.
- D. M. Nobili, M. Contin, C. Mondini and P. C. Brookes, Soil Biol. Biochem., 2001, 33, 1163–1170 CrossRef.
- X. D. Jiang, S. Y. Chi, Y. Wang, T. Y. Ning and Z. J. Li, Trans. Chin. Soc. Agric. Eng., 2009, 25, 247–251 Search PubMed.
- J. Pramod, G. Nikita, B. L. Lakaria, A. K. Biswas and A. S. Rao, Soil Tillage Res., 2012, 121, 57–62 CrossRef.
- S. Z. Tian, T. Y. Ning, S. Y. Chi, Y. Wang, B. W. Wang, H. F. Han, C. Q. Li and Z. J. Li, Acta Ecol. Sin., 2012, 32, 879–888 CrossRef CAS.
- C. Tu, F. D. Li, Y. F. Qiao, N. Zhu, C. K. Gu and X. Zhao, J. Integr. Agric., 2017, 16, 967–979 CrossRef.
- L. T. Liu, C. S. Hu, P. P. Yang, Z. Q. Ju, J. E. Olesen and J. W. Tang, Agric. For. Meteorol., 2015, 207, 38–47 CrossRef.
- S. T. Chen, Y. Zhang, Z. H. Hu and X. S. Shen, Environ. Sci. Technol., 2012, 33, 1476–1483 Search PubMed.
- X. Yan, F. G. Zhang, L. Yang, T. Li, Y. Y. Che and S. J. Deng, Eur. J. Soil Biol., 2018, 85, 23–29 CrossRef.
|
This journal is © The Royal Society of Chemistry 2018 |