DOI:
10.1039/C8RA00633D
(Paper)
RSC Adv., 2018,
8, 12870-12878
Characterization of CMC–LDH beads and their application in the removal of Cr(VI) from aqueous solution
Received
22nd January 2018
, Accepted 20th March 2018
First published on 5th April 2018
Abstract
In this study, CMC–LDH beads were prepared and characterized using SEM, FTIR and TG analysis. The beads were applied for the removal of Cr(VI) from aqueous solution. The effects of adsorbent dosage, initial pH and initial concentration of Cr(VI) solution on Cr(VI) uptake were investigated in detail. Moreover, adsorption isotherms and adsorption kinetic models were employed to analyze the adsorption process, and a preliminary study of the reusability of the adsorbent was performed. The experimental results showed that the CMC–LDH beads could remove Cr(VI) from aqueous solution efficiently. When the initial concentration of the Cr(VI) solution was 100 mg L−1 and the adsorbent dosage was 12 g L−1, the removal efficiency of Cr(VI) reached 96.2%. After the CMC–LDH beads were reused 10 times, the removal efficiency of Cr(VI) still remained at 89.6%.
1. Introduction
Heavy metal pollution has attracted widespread attention due to the high toxicity and irreversibility, which could cause environmental pollution even at low levels. Chromium is a toxic heavy metal which can easily contaminate soil and water and can harm people’s health. Due to its wide industrial use, chromium pollution most commonly arises from mining, electroplating, leather making, etc. Chromium mainly exists in hexavalent [CrO42−, Cr2O72− and Cr(VI)] and trivalent [Cr(OH)3 and Cr(III)] forms in the natural environment. Cr(VI) is a naturally occurring metal, the ratio of hexavalent chromium to total chromium is less than 3%. However, chromium has the characteristics of high toxicity and high solubility and is carcinogenic, which could lead to hepatic or renal damage, gastrointestinal irritation, central nervous system irritation,1,2 etc. It has been reported that Cr(VI) is much more toxic than Cr(III).3 In China, chromium salt induction is one of the most serious pollution, and the removal or disposal of heavy metal pollutants from wastewater is an urgent problem to be solved. Different technologies4 for the removal of heavy metal ions, including chemical precipitation, ion-exchange, adsorption, membrane filtration and electrochemical treatment, have been used. Among them, the adsorption process is recognized as an effective and economical method for wastewater treatment, and the application of low-cost adsorbents for wastewater treatment is an area of great interest for scientific researchers.
Carboxymethylcellulose (CMC), a derivative of natural cellulose produced via alkalization and etherification, is abundant, reproducible and biodegradable.5,6 CMC contains a large number of hydroxyl groups and carboxyl groups in its molecular chain, both of which can react with metal ions. Layered double hydroxides (LDHs) are layered materials with a chemical composition represented by the general formula [M(II)1−xM(III)x(OH)2]x+[Ax/nn−]·mH2O,7 where M(II) and M(III) are divalent and trivalent metal ions, respectively; An− represents charge compensation anions or gallery anions, such as CO32−, Cl−, or organic anions; x is the ratio of M(III) to the total amount of M(II) and M(III), and m represents the amount of crystallization water.8,9 Due to their special layered structure, low cost and ease of synthesis, LDHs can be used as adsorbents to treat heavy metal ions in contaminated water,10,11 and they exhibit good application prospects for environmental pollution control.
CMC is transparent, non-toxic, biocompatible and biodegradable. LDHs have unique characteristics, such as their facile synthesis, versatility and tunable composition, which make them attractive for the preparation of bio-nanocomposites. In the presence of Al3+ or Fe3+, a mixture of CMC and LDHs can result in the formation of uniform and small-sized spherical beads. In recent years, some research has focused on the preparation of CMC–LDH composite materials and their application in the medical field,12,13 whereas their application for wastewater treatment has rarely been reported in the literature. Considering the factors mentioned above, a coprecipitation method was employed for the preparation of LDHs in this study. Then we attempted to prepare a kind of spherical adsorbent, which is expected to be more convenient to use and recycle than pure LDHs for the adsorption of heavy metal ions. The synthesized products were characterized using scanning electron microscopy (SEM), Fourier transform infrared spectroscopy (FTIR) and thermogravimetric (TG) analysis. Furthermore, the resulting CMC–LDH beads were used for the removal of Cr(VI) from aqueous solution. The impact of various factors, such as adsorbent dosage, pH and contact time, on the removal rate of Cr(VI) during the adsorption process was investigated. Moreover, adsorption isotherms and adsorption kinetic models were employed to analyze the adsorption behavior. Finally, a preliminary study of the reusability of the adsorbent was performed.
2. Experimental section
2.1 Materials
CMC, with a degree of substitution (DS) of 0.7 and a viscosity of 50–100 mPa s (1% in H2O, 25 °C), was purchased from Aladdin Chemistry Co. Ltd, China. All of the other chemicals used in the present research were of analytical grade and were obtained from Guangzhou Chemical Reagent Factory, China.
2.2 Preparation of LDHs
Mg–Al–LDH was prepared via a coprecipitation method.14 Firstly, a nitrate solution was prepared by dissolving 0.12 mol Mg(NO3)2·6H2O and 0.04 mol Al(NO3)3·9H2O in distilled water. Then the resulting aqueous solution was made up to 100 mL. Meanwhile, an alkaline solution was made up to 160 mL with 0.32 mol NaOH and 0.08 mol NaNO3. Secondly, the alkaline solution was added dropwise to the metal nitrate solution over 2 hours (2 h) under a N2 atmosphere. The pH of the slurry was adjusted to 10 using NaOH solution, and the slurry was crystallized at 70 °C for 24 h. Afterwards, the precipitate was centrifuged and washed with distilled water to neutralise the LDH and it was then dried at 50 °C.
2.3 Preparation of CMC–LDH beads
4 g LDH was dispersed in 100 mL distilled water. Then, 2 g CMC was added into this solution with continuous stirring until a homogeneous solution was obtained. Afterwards, the homogeneous solution was added drop-wise into a 100 mL Al3+ solution (0.1 mol L−1) using a syringe. After that, the beads were crosslinked with Al3+ for 2 h. Finally, the resulting CMC–LDH beads were rinsed with deionized water to remove unreacted Al3+ and they were then dried at 50 °C.
2.4 Characterization
Infrared spectra were obtained using a FTIR spectrometer (Bruker Instruments, model VerTex70, Germany) in the 4000–400 cm−1 range at a resolution of 0.5 cm−1 with KBr pellets. Scanning electron microscopy (SEM) images were obtained using a scanning electronic microscope (Zeiss Instruments, model Merlin, Germany) operating at 5 kV. The thermal properties were measured using a simultaneous thermal analyzer (NETZSCH, model STA449 F3, Germany), and 10 mg samples were heated from room temperature to 600 °C at a heating rate of 10 °C min−1 under a N2 atmosphere.
2.5 Adsorption experiment
A stock solution of Cr(VI) was prepared using potassium dichromate, which was dried at 120 °C for 2 h in advance. All of the adsorption experiments were conducted in 250 mL beaker flasks containing 100 mL Cr(VI) solution, which were equilibrated at 180 rpm. The effect of initial pH (varied from 3 to 8) and adsorbent dosage (varied from 4 to 16 g L−1) on the removal of Cr(VI) using CMC–LDH beads was investigated, with an initial concentration of 100 mg L−1 at 35 °C. The impact of contact time was also investigated, with an initial concentration of 100 mg L−1 at 35 °C. During the kinetic adsorption experiments, samples were collected after 5, 10, 15, 20, 30, 40, 60, 80, 100, 120, 150 and 180 min of agitation. In addition, the adsorption isotherm experiments were conducted in the range 20–40 °C with various initial concentrations of Cr(VI), ranging from 100 to 500 mg L−1.
The adsorption capacity and removal rate of Cr(VI) were calculated using the following equations:
Adsorption capacity:
|
 | (1) |
Removal rate:
|
 | (2) |
where
C0 and
Ce represent the initial and equilibrium concentrations of Cr(
VI) (mg L
−1), respectively,
V is the volume of the Cr(
VI) solution and
W is the weight of the dried adsorbent.
2.6 Desorption experiment
In this study, desorption experiments were conducted using 1 mol L−1 NaNO3 solution. The adsorbed saturated CMC–LDH beads were immersed in NaNO3 solution at room temperature with a constant stirring rate of 180 rpm. Then the beads were rinsed with deionized water several times and dried. After that, the obtained beads were used to adsorb Cr(VI) from aqueous solution. The initial concentration of Cr(VI) and the contact time were 50 mg L−1 and 180 min, respectively.
3. Results and discussion
3.1 Characterization of CMC–LDH beads
In order to provide a visual illustration of the removal efficiency of Cr(VI) using the CMC–LDH beads, digital photographs of the different samples were taken, as shown in Fig. 1. All of the beads were spherical in shape, with a diameter of about 0.5–0.8 mm. The color of the Cr(VI) solution became light after treatment with the CMC–LDH beads. However, there was no distinct color loss in the Cr(VI) solution after adsorption by the CMC beads, which is consistent with the color difference between the CMC and CMC–LDH beads after adsorbing Cr(VI). It can be concluded that the CMC–LDH beads can remove Cr(VI) effectively. The morphology of the CMC beads, CMC–LDH beads and LDHs was also studied using SEM and the images are displayed in Fig. 2. According to the figures, the LDH samples exhibited a platelet-like morphology. Severe wrinkles were observed on the surface of the CMC bead, which is related to the partial collapse of the polymer network during drying.13 However, the surface of the CMC–LDH beads is smoother. Fig. 3 shows the EDS mapping images of the CMC–LDH beads. It is noted that the LDH particles were dispersed in the CMC matrix.
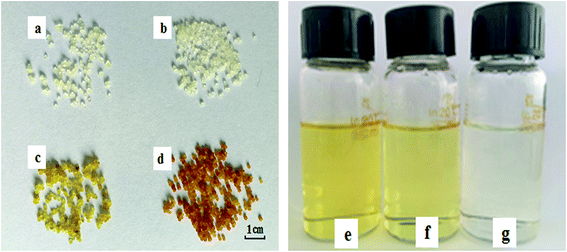 |
| Fig. 1 Digital photographs of the CMC beads (a), CMC–LDH beads (b), CMC beads after adsorbing Cr(VI) (c), CMC–LDH beads after adsorbing Cr(VI) (d), Cr(VI) solution with a concentration of 100 mg L−1 (e) and Cr(VI) solution with a concentration of 100 mg L−1 after adsorption by the CMC beads (f) and CMC–LDH beads (g). | |
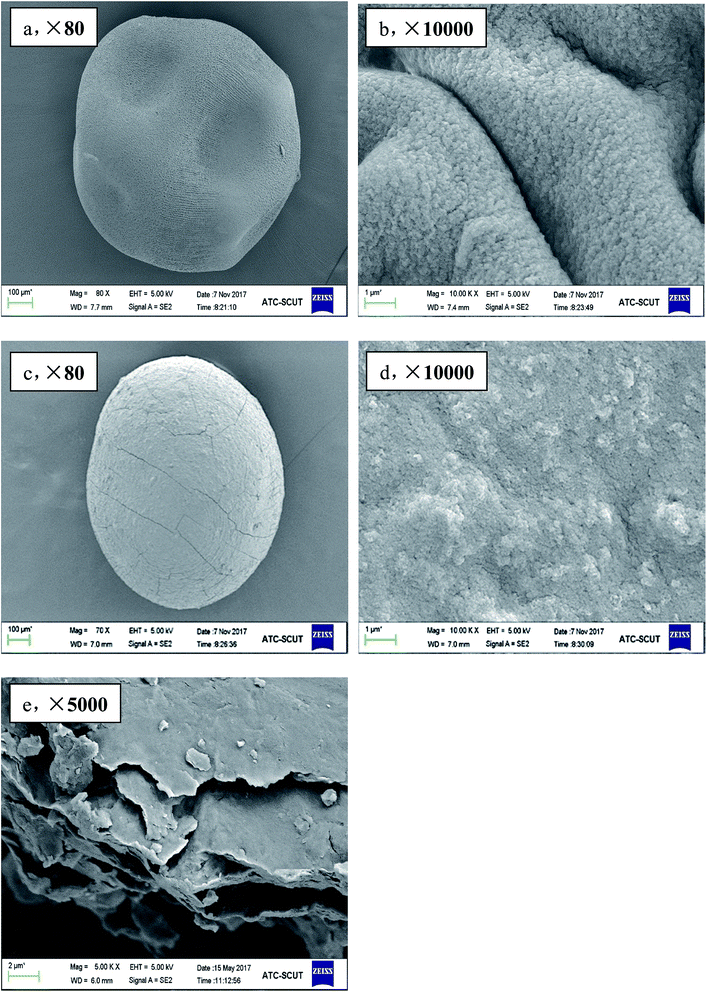 |
| Fig. 2 SEM images of the CMC beads (a and b), CMC–LDH beads (c and d) and LDHs (e). | |
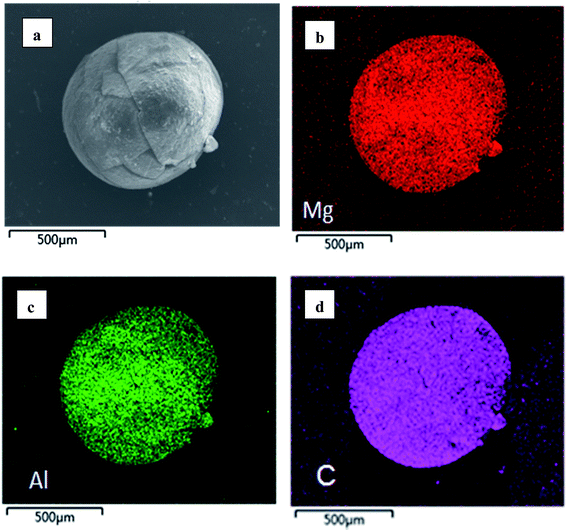 |
| Fig. 3 EDS mapping images of the CMC–LDH beads. | |
Fig. 4 shows the FTIR spectra of the different samples. The broad bands ranging from 3600 to 3400 cm−1 are ascribed to the stretching vibrations of the –OH groups. The peaks for CMC and the CMC–LDH beads at 1433 cm−1 and 1620 cm−1 are attributed to the symmetrical and asymmetrical stretching vibrations of the carboxylate group. The obvious peak for the LDHs at 1380 cm−1 is due to the NO3− stretching vibration. A new vibration peak at 910 cm−1, for the CMC–LDH beads after adsorption, was observed, revealing the presence of CrO24−.
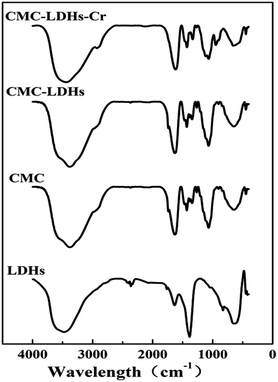 |
| Fig. 4 FTIR spectra of the samples. | |
It has been demonstrated from the TG and DTG curves of the CMC–LDH beads (Fig. 5) that there are two main weight loss steps. The first weight loss step below 240 °C is mainly ascribed to water desorption from the CMC–LDH beads, including water loss from CMC and interlayer water loss from the LDHs. It has been reported that all of the interlayer H2O molecules are removed upon heating to 250 °C,15 which is mainly related to the distinct peak in the DTG curve at around 230 °C. The second weight loss step occurred within a temperature range of 240 °C to 600 °C, and this is attributed to the decomposition of CMC and the LDHs. The rate of weight loss of the CMC–LDH beads was 52.7% at 600 °C.
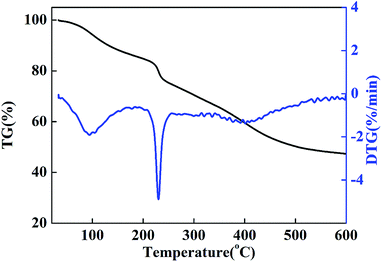 |
| Fig. 5 TG and DTG curves of the CMC–LDH beads. | |
3.2 Effect of adsorbent dosage on Cr(VI) uptake
Fig. 6 shows the effect of adsorbent dosage on the removal of Cr(VI). It was found that the removal rate of Cr(VI) increases gradually with the increase of adsorbent dosage, which is ascribed to the greater surface area of the adsorption material and the availability of the increasing adsorption sites. However, a plateau was observed when the dosage was above 14 mg L−1, which is attributed to the increase of vacant active adsorption sites. In other words, Cr(VI) didn’t make full use of all the adsorption sites during the adsorption process.
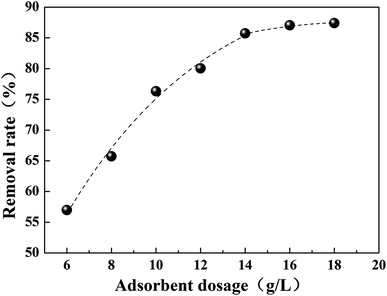 |
| Fig. 6 The effect of adsorbent dosage on Cr(VI) uptake by CMC–LDH beads. | |
3.3 Effect of contact time on Cr(VI) uptake
The impact of contact time on the removal of Cr(VI) by CMC–LDH beads was studied and the results are shown in Fig. 7. The removal rate showed a rapid increase within the first 30 min, and this then turned into a modest increase during the following adsorption. During the process of adsorption, the adsorbed Cr(VI) had a tendency to desorb into the solution. The desorption function and adsorption function would reach a dynamic equilibrium eventually. Therefore, the amount of Cr(VI) reached a near constant value when the contact time was over 120 min, suggesting that adsorption saturation had been achieved. In order to ensure that the adsorption process reached the equilibrium state, subsequent experiments were conducted for 180 min.
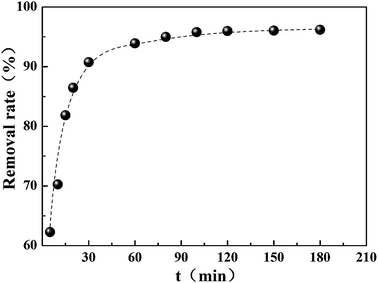 |
| Fig. 7 The effect of contact time on Cr(VI) uptake by CMC–LDH beads. | |
3.4 Effect of initial pH on Cr(VI) uptake
The effect of the initial pH of the solution on the removal of Cr(VI) was investigated. Fig. 8 shows the adsorption capacity and removal rate at different pH levels. According to the figure, it can be noted that pH had little influence on the adsorption capacity of Cr(VI) by the CMC–LDH beads, and the adsorption capacity showed a reduction from 25.1 mg g−1 to 23.2 mg g−1 along with the pH increase. The reason for this is because OH− was adsorbed on the surface of the CMC–LDH beads, resulting in the competitive adsorption of OH− and Cr(VI) from the solution. Meanwhile, it was observed that the removal rate decreased from 73.6% to 69.9%. The pH values of the Cr(VI) solution before and after adsorption are shown in Table 1. The equilibrium pH of the solution remained steady at 6.3–6.9, which may be related to the adsorption of OH− in the interlayer position of the LDHs.
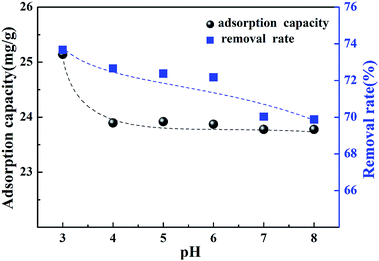 |
| Fig. 8 The effect of pH on Cr(VI) uptake by CMC–LDH beads. | |
Table 1 The pH of the Cr(VI) solution before adsorption and after adsorption
Initial pH |
3.0 |
4.0 |
5.0 |
6.0 |
7.0 |
8.0 |
Equilibrium pH |
6.3 |
6.4 |
6.4 |
6.6 |
6.8 |
6.9 |
3.5 Adsorption kinetics
Mathematical modeling is recognized as a powerful tool for the analysis of the adsorption process. In this paper, both pseudo-first-order and pseudo-second-order kinetic models were applied to investigate the kinetics of Cr(VI) adsorption at various initial concentrations.
Pseudo-first-order model:16
|
ln(qe − qt) = ln qe − k1t
| (3) |
Pseudo-second-order model:17
|
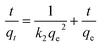 | (4) |
where
qe and
qt are the amounts of Cr(
VI) adsorbed (mg g
−1) at equilibrium and at any time, respectively. Here
t is the adsorption time (min), and
k1 (min
−1) and
k2 (g mg
−1 min
−1) are the rate constants for the pseudo-first order and pseudo-second order models, respectively.
On the basis of the equations mentioned above and the laboratory data, the kinetic parameters and the adsorption capacity could be determined using modeling techniques. The kinetic models for Cr(VI) adsorption on the CMC–LDH beads, according to pseudo-first-order and pseudo-second-order kinetics, are shown in Fig. 9. The kinetic constants and correlation coefficients (R2), obtained using linear regression, for the two models are listed in Table 2. The R2 value obtained from the pseudo-first-order model was lower than that obtained from the pseudo-second-order model, and the calculated qe obtained from the pseudo-second-order model was much closer to the experimental value of qe, around 0.999, which confirms that the pseudo-second-order model matches better with the real adsorption process of Cr(VI), in the present research, when compared with the other model. With the increase in the initial concentration of Cr(VI), k2 gradually became smaller. The higher initial concentration meant that there was a greater probability of the Cr(VI) ions colliding with each other, while the time they took to combine with the active site of the adsorption beads increased, which is consistent with the literature.18
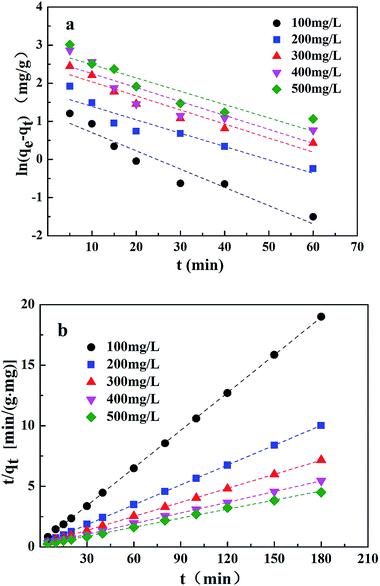 |
| Fig. 9 Adsorption kinetics for Cr(VI) uptake on CMC–LDH beads ((a) pseudo-first-order model; (b) pseudo-second-order model). | |
Table 2 The pseudo-first-order and pseudo-second-order rate parameters for Cr(VI) adsorption on CMC–LDH beads
|
100 mg L−1 |
200 mg L−1 |
300 mg L−1 |
400 mg L−1 |
500 mg L−1 |
Pseudo-first-order model |
k1 (min−1) |
0.0479 |
0.0351 |
0.0369 |
0.0366 |
0.035 |
qe (mg g−1) |
3.3 |
5.7 |
11.1 |
13.7 |
17.1 |
R2 |
0.9285 |
0.8987 |
0.9239 |
0.7956 |
0.8648 |
![[thin space (1/6-em)]](https://www.rsc.org/images/entities/char_2009.gif) |
Pseudo-second-order model |
k2 [g mg−1 min−1] |
0.0359 |
0.0154 |
0.0074 |
0.0056 |
0.0046 |
qe (mg g−1) |
9.7 |
18.3 |
25.9 |
34.0 |
40.2 |
R2 |
0.9999 |
0.9999 |
0.9999 |
0.9997 |
0.9985 |
![[thin space (1/6-em)]](https://www.rsc.org/images/entities/char_2009.gif) |
Experimental |
qe (mg g−1) |
9.5 |
18.0 |
25.1 |
33.0 |
39.9 |
3.6 Adsorption isotherms
Langmuir and Freundlich adsorption isotherms are significant for describing the interaction between the adsorbent and adsorbate. The equations are shown as follows:
Langmuir:19
|
 | (5) |
Freundlich:20
|
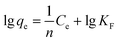 | (6) |
where
Ce represents the equilibrium ion concentration in solution,
qe is the amount of Cr(
VI) adsorbed (mg g
−1) in the equilibrium state and
qm (mg g
−1) is the maximum uptake of Cr(
VI). In addition,
b (L mg
−1) is a constant that is related to the energy of adsorption in the Langmuir adsorption isotherm,
KF is a constant that represents the adsorption capacity in the Freundlich adsorption isotherm and is related to the bond strength and

is a constant corresponding to the adsorption intensity.
The Langmuir and Freundlich adsorption isotherm models were investigated, as shown in Fig. 10. The experimental data fitted well with these two kinetic models, and the estimated parameters are displayed in Table 3. According to the results obtained using linear regression for the two models, the correlation coefficients (R2) for both the Langmuir model and the Freundlich model could reach 0.99. It has been revealed in the present research that both these models are reliable for describing the adsorption behavior. The Langmuir isotherm model is based on the assumption that the adsorption sites are identical and energetically equivalent, and only monolayer adsorption occurs in the process.21 However, the Freundlich isotherm model is based on the assumption of exponentially decaying adsorption site energy distribution and is used for heterogeneous surface energy systems.22 Therefore, both monolayer and multilayer adsorption were involved in the removal process of Cr(VI) by CMC–LDH beads.
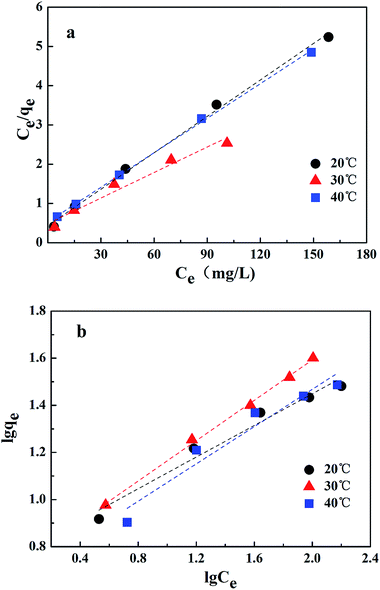 |
| Fig. 10 Adsorption isotherms for Cr(VI) uptake by CMC–LDH beads ((a) Langmuir; (b) Freundlich). | |
Table 3 Isotherm parameters for Cr(VI) adsorption by CMC–LDH beads
|
20 °C |
30 °C |
40 °C |
Langmuir |
b (L mg−1) |
0.0703 |
0.0444 |
0.0549 |
qm (mg g−1) |
32.4 |
46.1 |
34.1 |
R2 |
0.9966 |
0.9680 |
0.9992 |
![[thin space (1/6-em)]](https://www.rsc.org/images/entities/char_2009.gif) |
Freundlich |
KF [mg g−1 (L mg−1)1/n] |
0.20 |
0.16 |
0.17 |
1/n |
0.336 |
0.4283 |
0.3957 |
R2 |
0.9704 |
0.9979 |
0.9440 |
3.7 Desorption and reusability
Recyclability is an important factor to be considered when assessing an adsorbent. The reusability of the CMC–LDH beads was investigated preliminarily. The removal rate of Cr(VI) by the CMC–LDH beads after different recycling times of desorption was investigated. According to Table 4, the removal rate showed a decline from 94.5% to 89.6% after 10 cycles, which indicates that the CMC–LDH beads are suitable for practical applications.
Table 4 The reusability behavior of the CMC–LDH beads for Cr(VI) removal
Cycle time |
1 |
2 |
3 |
4 |
5 |
6 |
7 |
8 |
9 |
10 |
Removal rate (%) |
94.5 |
93.9 |
93.8 |
92.6 |
91.9 |
91.5 |
90.7 |
90.1 |
90.1 |
89.6 |
4. Conclusions
CMC–LDH beads were prepared, characterized and applied for the removal of heavy metal ions in this study. The results confirmed that CMC–LDH beads could remove Cr(VI) from aqueous solution efficiently. It was verified that the pseudo-second-order model was more appropriate for the actual adsorption process of Cr(VI), in the present research, which was in accordance with the adsorption kinetics. The adsorption mechanisms, which included both a monolayer and multilayer adsorption process, were investigated using adsorption isotherms. The CMC–LDH beads could be reused and the removal rate was 89.6% after 10 cycles. Overall, CMC–LDH beads could be recognized as a promising adsorbent for Cr(VI) uptake on account of their high removal rate, excellent reusability and environmental friendliness.
Conflicts of interest
There are no conflicts to declare.
Acknowledgements
The authors acknowledge the National Key R&D Program of China (2017YFB0308003), the Science and Technology Planning Project of Guangdong Province (2015A020215007), the Natural Science Foundation of China (No. 31670586), the Fundamental Research Funds for the Central Universities (2017ZD091 and 2017MS073), and the State Key Laboratory of Pulp and Paper Engineering Program (2016C05) for sponsoring this research.
References
- M. D. Cohen, B. Kargacin and C. B. Klein, et al., Mechanisms of Chromium Carcinogenicity and Toxicity, Crit. Rev. Toxicol., 1993, 23(3), 255–281 CrossRef CAS PubMed
. - J. E. Mclean, L. S. Mcneill and M. Edwards, et al., Hexavalent chromium review: Part 1, health effects, regulations, and analysis, J. Am. Water Works Assoc., 2012, 104(6), 348–358 CrossRef
. - M. Chebeir and H. Liu, Kinetics and Mechanisms of Cr(VI) Formation via the Oxidation of Cr(III) Solid Phases by Chlorine in Drinking Water, Environ. Sci. Technol., 2016, 50(2), 701–710 CrossRef CAS PubMed
. - F. Fu and Q. Wang, Removal of heavy metal ions from wastewaters: a review, J. Environ. Manage., 2011, 92(3), 407–418 CrossRef CAS PubMed
. - M. J. Waring and D. Parsons, Physico-chemical characterisation of carboxymethylated spun cellulose fibres, Biomaterials, 2001, 22(9), 903–912 CrossRef CAS PubMed
. - M. Boruah and P. Gogoi, et al., Biocompatible carboxymethylcellulose-g-poly(acrylic acid)/OMMT nanocomposite hydrogel for in vitro release of vitamin B12, RSC Adv., 2014, 4(83), 43865–43873 RSC
. - C. Xing, F. Musharavati and H. Li, et al., Synthesis, characterization, and properties of nickel–cobalt layered double hydroxide nanostructures, RSC Adv., 2017, 7(62), 38945–38950 RSC
. - J. He, M. Wei and B. Li, et al., Preparation of Layered Double Hydroxides, Springer, Berlin, Heidelberg, 2006, pp. 345–373 Search PubMed
. - G. Mishra, B. Dash and S. Pandey, Layered double hydroxides: A brief review from fundamentals to application as evolving biomaterials, Appl. Clay Sci., 2018, 153, 172–186 CrossRef CAS
. - Y. H. Chuang, M. T. Yu and M. K. Wang, et al., Removal of 2-Chlorophenol from Aqueous Solution by Mg/Al Layered Double Hydroxide (LDH) and Modified LDH, Ind. Eng. Chem. Res., 2008, 47(11), 3813–3819 CrossRef CAS
. - T. Zhang, Q. Li and H. Xiao, et al., Synthesis of Li–Al Layered Double Hydroxides (LDHs) for Efficient Fluoride Removal, Ind. Eng. Chem. Res., 2012, 51(35), 11490–11498 CrossRef CAS
. - M. Yadollahi, H. Namazi and M. Aghazadeh, Antibacterial carboxymethyl cellulose/Ag nanocomposite hydrogels cross-linked with layered double hydroxides, Int. J. Biol. Macromol., 2015, 79, 269–277 CrossRef CAS PubMed
. - S. Barkhordari and M. Yadollahi, Carboxymethyl cellulose capsulated layered double hydroxides/drug nanohybrids for Cephalexin oral delivery, Appl. Clay Sci., 2016, 121–122, 77–85 CrossRef CAS
. - T. Toraishi, S. Nagasaki and S. Tanaka, Adsorption behavior of IO3− by CO32−- and NO3−-hydrotalcite, Appl. Clay Sci., 2002, 22(1), 17–23 CrossRef CAS
. - G. S. Thomas, A. V. Radha and P. Vishnu Kamath, et al., Thermally Induced Polytype Transformations among the Layered Double Hydroxides (LDHs) of Mg and Zn with Al, J. Phys. Chem. B, 2006, 110(25), 12365–12371 CrossRef CAS PubMed
. - A. R. Ricardo, G. Carvalho and S. Velizarov, et al., Kinetics of nitrate and perchlorate removal and biofilm stratification in an ion exchange membrane bioreactor, Water Res., 2012, 46(14), 4556–4568 CrossRef CAS PubMed
. - M. Hamayun, T. Mahmood and A. Naeem, et al., Equilibrium and kinetics studies of arsenate adsorption by FePO4, Chemosphere, 2014, 99(3), 207–215 CrossRef CAS PubMed
. - S. Chen, Q. Yue and B. Gao, et al., Removal of Cr(VI) from aqueous solution using modified corn stalks: Characteristic, equilibrium, kinetic and thermodynamic study, Chem. Eng. J., 2011, 168(2), 909–917 CrossRef CAS
. - P. Baskaralingam, M. Pulikesi and D. Elango, et al., Adsorption of acid dye onto organobentonite, J. Hazard. Mater., 2006, 128(2–3), 138–144 CrossRef CAS PubMed
. - M. Dalaran, S. Emik and G. Güçlü, et al., Removal of acidic dye from aqueous solutions using poly(DMAEMA–AMPS–HEMA) terpolymer/MMT nanocomposite hydrogels, Polym. Bull., 2009, 63(2), 159–171 CrossRef CAS
. - I. Langmuir, The adsorption of gases on plane surfaces of glass, mica and platinum, J. Chem. Phys., 1918, 40(12), 1361–1403 CAS
. - H. Freundlich, Of the adsorption of gases. Section II. Kinetics and energetics of gas adsorption. Introductory paper to Section II, Trans. Faraday Soc., 1932, 28(7), 195–201 RSC
.
|
This journal is © The Royal Society of Chemistry 2018 |