DOI:
10.1039/C7RA12753G
(Paper)
RSC Adv., 2018,
8, 4006-4013
UV and blue-light anti-reflective structurally colored contact lenses based on a copolymer hydrogel with amorphous array nanostructures†
Received
24th November 2017
, Accepted 8th January 2018
First published on 22nd January 2018
Abstract
This study presents a facile and inexpensive method for fabricating novel contact lenses with anti-reflective (AR) properties for UV and blue light based on a copolymer hydrogel with amorphous array (AA) nanostructures. The AR structurally colored contact lenses exhibited high optical transparency, homogeneous bluish structural color, and physical rigidity. In addition, the proposed contact lenses blocked short-wavelength blue transmittance by approximately 20% and had high visible-light transmittance of over 90%. Consequently, the risk of harm posed by commercial contact lenses with chemical dyes was avoided. Adjusting the volume fraction of the silica nanospheres of the AAs in the hydrogel contact lenses was quite effective for controlling the structural colors, transmittance, equilibrium water content (EWC), and oxygen permeability (Dk). The EWC and Dk of the structurally colored contact lenses were in the range of 40.14–45.65% and 11.6–13.7 barrers, respectively. These values indicate that the characteristics of the AR structurally colored contact lenses satisfied the requirements of daily disposable contact lenses. Therefore, the proposed AR structurally colored contact lenses are very promising as substitutes for commercial colored contact lenses with chemical dyes.
1. Introduction
White light-emitting diodes (WLEDs) in solid-state devices have been widely applied for the backlight modules of digital screens, such as TVs, LCDs, tablets, and mobile phones.1,2 Commercially available WLEDs generally use blue LEDs to excite yellow phosphor.3 Therefore, WLEDs contain high-energy blue light, which is responsible for the blue-light hazard (BLH) problem. A report by the US Department of Energy on BLH discussed how exposure to excessive blue light at approximately 400–480 nm damages the retina. More and more people are being exposed to high-energy blue light from WLEDs, which has been demonstrated to affect human physiology and psychology.4–6 In addition, UV light and strong blue-light radiation damage the human eye and cause diseases, such as cataracts and age-related macular degeneration, by cumulative injury.7,8 Soft colored contact lenses are commonly used worldwide because these lenses enable the correction of vision, as well as for fashion and beauty purposes. Therefore, developing colored contact lenses with UV and blue-light anti-reflective (AR) properties is extremely important. In this study, we propose novel structurally colored contact lenses with UV and blue-light AR properties using amorphous array (AA) nanostructures.
The structural color of photonic structures is generally divided into periodic structures consisting of long-range ordered structures with iridescence (angle-dependent)9,10 and short-range ordered amorphous (or quasi-amorphous) structures with non-iridescence (angle-independent).11–13 Structural color is color generated by scattering, refraction, interference, or diffraction of visible light by microstructures or nanostructures. The mechanism of generating structural color differs from those of chemical dyes and pigments. Non-iridescent structural colors with unique properties endow AAs with mild, bright and angle-independent characteristics, which have been proposed for application in optical devices.14
Generation of a colloidal nanosphere suspension is a facile approach to prepare structurally colored materials with periodic or AA nanostructures.9–13 Structural color can be used as an optimal method for fabricating colored contact lenses compared with commercial colored contact lenses because of the bright color and absence of chemical dye in the former. Gu et al. reported two approaches for fabricating structurally colored contact lenses using periodic crystal nanostructures.15,16 The iridescent contact lenses exhibited brilliant colors under light illumination, but they did not block UV and blue light. Therefore, we propose novel structurally colored contact lenses that are AR for UV and blue light using AA nanostructures. In this study, we report a facile approach for fabricating the proposed colored contact lens by decorating the hydrogels with AAs by UV photo-polymerization in molds. The AR structurally colored contact lenses were fabricated by dispersing silica nanoparticles with AAs in the copolymer hydrogel precursor. Silica nanospheres were used because silica materials are generally recognized as safe by the United States Food and Drug Administration. The obtained AR contact lenses blocked UV and blue light, transmitted over 90% of visible light, and had a bluish structural color without using chemical dye, which prevented the potential harm from dye leakage posed by commercial colored contact lenses.
Poly(2-hydroxyethyl methacrylate) [poly(HEMA)] and poly(methylmethacrylate) [poly(MMA)] materials are commonly used in soft contact lenses.17 HEMA is the host material for soft contact lenses, whereas MMA can increase the hardness and adhesion of hydrogel contact lenses. In addition, N-vinylpyrrolidone (NVP) in poly(NVP-co-HEMA) hydrogels have also been widely studied and used as soft contact lenses because of their special molecular structure and ability to improve the water content of the hydrogel.18,19 Copolymerization of monomer MMA with N,N-dimethylacrylamide (DMAA) hydrogel has been reported to balance mechanical strength and water content.20 Moreover, increased DMAA enhanced the equilibrium water content (EWC) and oxygen permeability (Dk).21,22 In this study, the AAs of silica nanospheres were homogeneously dispersed in the hydrogel precursor containing HEMA, MMA, NVP, and DMAA without additional surfactant. The obtained AR structurally colored contact lenses exhibited blue structural color and blocked UV and blue light. The effect was varied by adjusting the volume fraction of silica nanospheres (ϕsilica). We also investigated the hydrogel material characteristics such as Fourier transform infrared (FT-IR) spectra, optical reflectance and transmittance, EWC, Dk, and contact angle (CA) to demonstrate the validity of this study for application in soft contact lenses. The AR property towards blue light of structurally colored contact lenses has substantial potential for commercial contact lenses, which use chemical dyes to absorb blue light (Fig. S1 and Table S1†).
2. Experimental section
2.1 Materials
Monomers HEMA, NVP, and DMAA were purchased from Sigma-Aldrich. MMA was purchased from Acros Organics. Ethylene glycol dimethylacrylate (EGDMA) as the crosslinking agent and 2-hydroxy-2-methylpropiophenone (HMPP) as the photoinitiator were purchased from Tokyo Chemical Industry Co., Ltd. The chemical reagents HEMA, MMA, EGDMA, and DMAA were purified by reduced pressure. Absolute ethanol (99.5%) was purchased from Echo Chem. Co., Ltd. Deionized (DI) water was used in this study. Monodisperse non-uniform silica nanospheres with a mean diameter (Dmean) of 151 nm and a polydispersity index of approximately 0.4 were synthesized to form AAs according to a previously reported method (Fig. S2†).13
2.2 Preparation of copolymer hydrogel with silica nanospheres of AAs
In this study, the copolymer hydrogel precursor mixtures containing HEMA, MMA, NVP, and DMAA monomers were mixed at a certain weight percentage (wt%) to produce contact lenses with high Dk properties. The mixtures were polymerized in the presence of 1 wt% EGDMA and 2 wt% HMPP to yield colorless transparent soft contact lenses (standard, Table S2†). Silica nanosphere powders with three different wt% were dispersed in 1.0 g of absolute ethanol using ultrasonication to complete dispersion. Then, suspensions with AAs of silica nanospheres at concentrations of 18.6 wt%, 33.8 wt%, and 46.8 wt% were mixed with the copolymer hydrogel by vortex mixing. Finally, the absolute ethanol in the mixing hydrogel was evaporated by heating the solutions at 50 °C in a convection oven. The final ϕsilica concentrations of AAs were 10% (sample A), 20% (sample B), and 30% (sample C). The synthesis parameters are summarized in Table S2.†
2.3 Preparation of the AR structurally colored contact lenses
A schematic illustration of the preparation of AR structurally colored contact lenses with AA nanostructures in molds is shown in Fig. 1. First, 50 μL of hydrogel with AAs was added to the polypropylene (PP) lens mold. Then, the top PP mold was applied carefully onto the bottom PP mold lid. During the pressing process, it was ensured that no air bubbles were produced. After photo-polymerization by UV light (48 mW cm−2) for 5 min, the top mold was carefully separated from the bottom mold and a solid-state structurally colored contact lens with bright color and good mechanical strength was obtained. Next, the structurally colored contact lens that adhered onto the top mold was hydrated by DI water and heated to 80 °C until the contact lens fell off from the top mold. Finally, the structurally colored contact lenses were washed and stored in DI water.
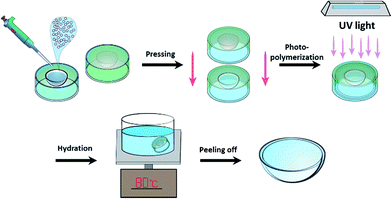 |
| Fig. 1 Schematic illustration of the fabrication of AR structurally colored contact lenses. | |
2.4 Characterization
The morphology of the silica nanospheres and the hydrogel with AAs were characterized using field emission scanning electron microscopy (FESEM) (S-4800; Hitachi) at 3 kV. The average diameter of the silica nanospheres was determined by dynamic light scattering using a laser particle size analyzer (LPSA) (N4 plus; Beckman Coulter). FT-IR spectra (Frontier; PerkinElmer Inc.) were measured using attenuated total reflection and recorded with a resolution of 1.0 cm−1. The reflectance and transmittance of the structurally colored contact lenses were measured using a UV-VIS-NIR spectrometer (HR2000; Ocean Optics) and a fiber-coupled UV-enhanced Xe lamp as the white-light source. The contact lenses were soaked in DI water and then mounted on the side surface of the cuvette. The cuvette was placed in a CUV-UV cuvette holder (Ocean Optics) and the measurements were performed at wavelengths ranging from 200 nm to 1100 nm. The Dk and EWC of the hydrogel contact lenses were measured according to ISO 18369-4:2006 (Ophthalmic Optics—Contact lenses—Part 4: Physicochemical properties of contact lens materials) using polarographic analysis and a gravimetric method, respectively.28,29 The Dk was analyzed by polarographic analysis using a Model 201T O2 premeometer with a polarographic electrode cell (8.5 mm radius; Createch/Rehder Co Dev), temperature and humidity chamber (Model PL-3J; ESPEC Corp.), and motorized thickness meter (Model VL-50; Mitutoyo Corp.). The CAs of the contact lenses with AAs were determined using a CA machine (FTA-1000B; first ten angstroms).
3. Results and discussion
3.1 FT-IR analysis of the copolymer hydrogel materials
Poly(HEMA) is generally used for manufacturing hydrogel contact lenses, but this compound has weak mechanical strength. Therefore, we copolymerized poly(HEMA) with a small amount of MMA to enhance the copolymer hydrogel. MMA can effectively enhance the strength of the gel network and has no effect on the transparency of the gel. NVP can improve the EWC of hydrogel contact lenses.18,19 Hydrophilic DMAA monomers have excellent biocompatibility and good Dk.21,22 The integrated poly(HEMA-co-MMA-co-NVP-co-DMAA) copolymer hydrogel was synthesized by free radical copolymerization using EGDMA as the crosslinker and HMPP as the photoinitiator. FT-IR spectra were used to characterize the synthesized copolymer hydrogels of all the produced contact lens samples (Fig. 2 and S3†).22,23
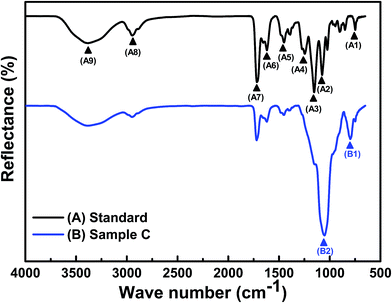 |
| Fig. 2 FT-IR spectra demonstrated the characteristics of the produced contact lens samples. | |
Both samples were characterized using FT-IR to establish the chemical functional groups of all the samples. Table 1 lists the major characteristic peak positions of the functional groups in the standard sample. The standard sample exhibited vibrational bands in the hydroxyl (O–H) (∼3381 cm−1, labelled as A9) and carbonyl (C
O) (∼1719 cm−1, labelled as A7) stretching regions, stretching of the C–N group (∼1246 cm−1, labelled as A4), and bands between 1451 cm−1 (labelled as A5) and 2933 cm−1 (labelled as A8) associated with the symmetric and asymmetric C–H stretching vibrations (CH2 and CH3 groups), respectively. These results show that the carbon functional groups on the membrane-polymer composites can be solely attributed to the presence of the HEMA polymer.23 In addition, the peak at 1151 cm−1 (labelled as A3) was caused by the symmetrical O–C stretching vibration, which demonstrated the existence of poly(HEMA-co-MMA-co-NVP-co-DMAA) copolymer segments in the hydrogel contact lenses.
Table 1 Spectral band assignment of standard contact lens sample
Functional group |
FT-IR peak position [cm−1] |
(A1) C–O–C bending |
748 |
(A2) C–O–C stretching |
1075 |
(A3) C–O–C stretching |
1151 |
(A4) C–N stretching |
1246 |
(A5) aliphatic carbon (–CH2) |
1451 |
(A6) C C stretching |
1620 |
(A7) C O stretching |
1719 |
(A8) aliphatic carbon (–CH3, –CH2) |
2933 |
(A9) O–H stretching |
3381 |
Further, the FT-IR spectrum of sample C was slightly different from that of the standard sample (Fig. 2). The FT-IR spectra of the calcined silica nanosphere powder is illustrated in Fig. S4.† The strong characteristic peaks at 797 cm−1 (labelled as B1) and 1055 cm−1 (labelled as B2) of sample C were attributed to the Si–O asymmetric and symmetric stretching of silica material structures, respectively.24 In addition, the samples with different ϕsilica values of AAs were measured by FT-IR spectra (Fig. S3†). Further, it is worth mentioning that the intensity of the peak corresponding to the Si–O linkage increases with increasing silica content (Fig. S3†). The results demonstrated that several bands (A1–A4) were covered by the signal of the silica material, demonstrating that these characteristics depend on the ϕsilica of the AAs.
3.2 Measurement of the optical characteristics of the AR structurally colored contact lenses
The preparation of the AR structurally colored contact lenses is illustrated in Fig. 1. The internal arrangement of silica nanospheres, which formed the AAs within the hydrogel contact lenses, was observed by FESEM. The FESEM images of sample C are shown in Fig. 3. The top surface and cross sections of the structurally colored contact lenses exhibited analogously amorphous crystal structures of silica nanoparticles in the copolymer hydrogel. Characterization of the copolymer hydrogel using FESEM should be performed during the drying process. Therefore, the lattice distances between the silica nanospheres in the FESEM images were shorter than the actual length in the contact lenses. In addition, the 2D fast Fourier transform (FFT) of the FESEM image [inset in Fig. 3(a) and (b)] shows circular and ellipse patterns, respectively. These patterns demonstrate that the AAs were distributed with long-range disordered and short-range ordered structures. Consequently, the angle-independent structural color of the contact lenses was attributed to the isotropic characteristic of the AAs.
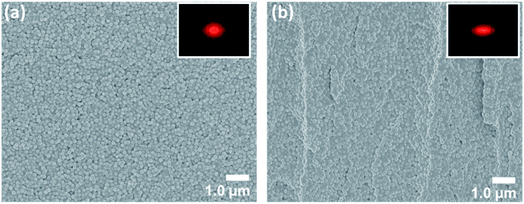 |
| Fig. 3 (a) Top surface and (b) cross-section of FESEM images of the AR structurally colored contact lenses with ϕsilica = 30% (sample C). Inset shows the 2D FFT images that exhibited long-range disordered and short-range ordered structures. | |
The fabricated structurally colored contact lenses demonstrated soft and flexible characteristics after hydration in DI water. Changes in the structural color (from colorless to blue) of the contact lenses are shown and compared in Fig. 4(a). Standard contact lenses are colorless, so are not easy to distinguish when being used. Therefore, commercial standard soft contact lenses add blue dyes into the contact lens hydrogel (Fig. S1†). In this study, bluish contact lenses were easily developed by increasing the ϕsilica of the AAs. When the ϕsilica was increased to 30% in the copolymer hydrogel, the contact lens of sample C generated bright structural colors [Fig. 4(a)]. The proposed structurally colored contact lenses were brilliant and semi-transparent. Fig. 4(b) and 5(b) exhibit the reflectance and transmittance spectra of the structurally colored contact lenses, respectively. The fabricated lenses were aspherical. Therefore, the reflection spectrum was measured on the top central point of the contact lens with a spot size of 5 mm diameter. The reflectance measurement was performed using the standard sample before and after hydration as reference for Fig. 4(b) and S5,† respectively. The reflectance spectra show the distinct weak reflection peak of the three ϕsilica of the structural color contact lenses that were approximately located at 427 nm (sample A, ϕsilica = 10%), 435 nm (sample B, ϕsilica = 20%), and 463 nm (sample C, ϕsilica = 30%) [Fig. 4(b)]. The reflection peaks could be attributed to the constructive interference of light from the AA nanostructures of the silica nanospheres. The reflection spectra [Fig. 4(b)] of the structurally colored contact lenses reveal a clear reflection peak only when ϕsilica = 30% of silica nanoparticles because the refractive indices of the silica nanospheres and hydrogel were very close. These results are also similar to those in previous reports.15,16
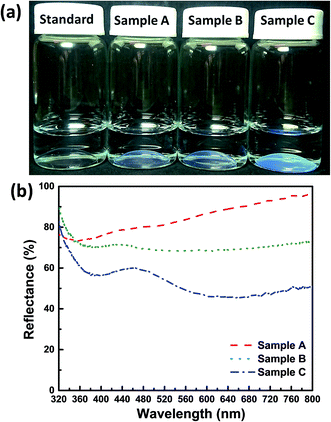 |
| Fig. 4 (a) Digital photographs of all the structurally colored contact lens samples. (b) Reflectance spectra of the three structurally colored contact lenses. | |
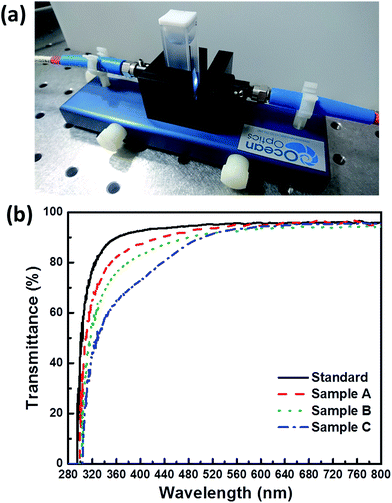 |
| Fig. 5 (a) Digital photograph of the lateral view of the transmittance measurement setup. (b) Transmittance spectra of all the contact lens samples. | |
In addition, the reflectance and transmittance spectra of sample C before hydration were measured, and the reflection peak was located at approximately 425 nm (Fig. S5†). The dominant reflection peak of the sample C after hydration exhibited a red shift to longer wavelengths, owing to the swelling of copolymer hydrogel in DI water caused by the increased lattice distance between two neighboring silica nanoparticles. Therefore, we calculated the coefficient of expansion of the interlayer space (dint) of sample C before and after the hydration reaction (ϕsilica = 30%). The effective refractive index (neff) can be obtained using eqn (1):
|
neff2 = nsilica2ϕsilica + nhydrogel2(1 − ϕsilica)
| (1) |
where
nsilica = 1.42 (
ref. 25) and
nhydrogel = 1.43.
22 In this case,
neff = 1.427 for sample C (
ϕsilica = 30%). Then, the reflection peak wavelength
λR of the vertical incident was determined according to the Bragg–Snell law [
eqn (2)] as follows:
13,14
Accordingly, the 425 nm and 463 nm reflection peak wavelengths (sample C) before and after hydration and neff can be calculated using eqn (2) to obtain dint_1 = 148.9 nm (before hydration) and dint_2 = 162.2 nm (after hydration). The dint_1 value was very consistent with the Dmean (approximately 151 nm) of the results from the LPSA (Fig. S2†). Therefore, the coefficient of expansion of the hydrogel for ϕsilica = 30% is 1.09 in the structurally colored contact lenses before and after hydration. This result is similar to that observed in the periodic crystal structure of contact lenses.15,16
In addition, the optical transmittance of all samples was measured using a cuvette (Fig. 5(a)). In this study, blue light is divided into the short-wavelength blue region (SWB, approximately 381–460 nm) and the long-wavelength blue region (LWB, approximately 461–500 nm). The transmittances of all contact lens samples are shown in Table 2. The American National Standards Institute of Z80.20 standard has regulated that contact lenses should satisfy Class II UV blocking, which blocks 70% of UV-A and 95% of UV-B radiation. Hydrogel contact lenses generally offer little blockage of UV and blue radiation. Therefore, UV absorbers should be added to commercial contact lenses (Fig. S1 and Table S1†) to satisfy the class II UV block. Transmittances for UV-B, UV-A, SWB, LWB, and visible light by the standard lens sample were 29.71%, 86.25%, 93.16%, 94.51%, and 95.05%, respectively. The visible-light transmittance of over 90% of all contact lenses satisfies the visual requirements, although these measurements also show that the standard hydrogel could not block UV-B and UV-A. By contrast, sample C with AAs (ϕsilica = 30%) exhibited optical transmittances of 7.42%, 58.24%, 76.88%, 87.30%, and 90.12% for UV-B, UV-A, SWB, LWB, and visible light, respectively. Therefore, sample C of the structurally colored contact lenses could block 92% of UV-B and 42% of UV-A [Fig. 5(b) and Table 2]. In addition, the graded drop in overall transmittance for blue light was estimated to reduce SWB transmittance by approximately 20% because of the structural color of AAs. This phenomenon is similar to the previously reported AA resin films.13,14 In general, the transmittance decreased as the ϕsilica of AAs increased, and this phenomenon was owing to the strong reflection of AAs. We also performed a transmittance stability test after all samples were stored in DI water for 155 days (Fig. S6†). No differences were found in the samples as shown by the digital photographs in Fig. S6(a).† However, all samples exhibited a smallest transmittance drop of 5% after storage for 155 days (Table 2). These results demonstrated the high stability of the structurally colored contact lenses.
Table 2 Optical transmittance of all the contact lens samples
T [%] |
UV-B (280–315 nm) |
UV-A (316–380 nm) |
SWB (381–460 nm) |
LWB (461–500 nm) |
Visible (381–780 nm) |
Standard |
29.71% |
86.25% |
93.16% |
94.51% |
95.05% |
Sample A |
18.22% |
77.05% |
88.88% |
92.50% |
93.69% |
Sample B |
11.98% |
70.01% |
85.07% |
90.01% |
91.50% |
Sample C |
7.42% |
58.24% |
76.88% |
87.30% |
90.12% |
![[thin space (1/6-em)]](https://www.rsc.org/images/entities/char_2009.gif) |
All the samples were stored in DI water for 155 days |
Standard |
26.69% |
85.35% |
92.83% |
93.70% |
94.08% |
Sample A |
16.53% |
72.05% |
83.77% |
88.95% |
91.76% |
Sample B |
11.34% |
67.54% |
81.29% |
86.96% |
89.65% |
Sample C |
7.37% |
57.32% |
73.00% |
82.65% |
88.20% |
3.3 Measurement of the characteristics of the AR structurally colored contact lenses
The contact lenses have two unique characteristics, namely EWC and Dk, which should be measured, because they demonstrate the properties of the hydrogel material. EWC was measured using thermogravimetry to evaluate the water content of the contact lenses.26 Thermogravimetry was also used to determine the ratio of free-to-bound water in the hydrogel material. The EWC of the standard sample was measured to be 54.70%. Sample A (ϕsilica = 10%) showed an average EWC of 45.65%, indicating that the AAs reduced the EWC. By contrast, the EWCs of samples B (ϕsilica = 20%) and C (ϕsilica = 30%) were 39.66% and 40.14%, respectively. These results demonstrated that AAs increased proportionally with the decrease in EWC. A comparison of the changes in the EWC of each sample is shown in Table 3. Specialists on contact lenses have recommended that the EWC of contact lenses should ideally range within 30 to 40%. EWC of more than 50% is high for contact lenses, and this characteristic facilitates wear and dry eyes, which lead to discomfort.27
Table 3 Dks and EWCs of all the contact lens samples
Sample |
Dka [barrers] |
R2 |
EWC [%] |
Conventional Dk units (×10−11 (cm2 s−1) [ml O2 (ml × mm Hg)−1]), also known as barrers. |
Standard |
16.9 |
0.9978 |
54.70% |
Sample A |
13.7 |
0.9986 |
45.65% |
Sample B |
11.5 |
0.9995 |
39.66% |
Sample C |
11.6 |
0.9914 |
40.14% |
In addition, the Dks of all samples were determined by measuring four different thicknesses of stacked contact lens samples to obtain multiple thicknesses (Fig. 6). Dk was derived by measuring the reading for each thickness. The process was repeated to provide the number of results shown in Fig. 6. Prior to the Dk measurements, all contact lens samples were required to be placed in a chamber to be stabilized at 35 ± 0.5 °C with an achievable humidity value of approximately 98%. The contact lens was carefully placed on the polarographic electrode cell and during the process it was ensured that no air bubbles were trapped between the contact lens and the electrode cell. The thicknesses of all samples were measured and the results were used to analyze and calculate the Dk. The measured Dks were applied to correct for the effects of the boundary-layer and edge.28,29 The measured Dk values of all contact lens samples are also summarized in Table 3. The measurement results exhibited that the Dk of the standard sample is 16.9 barrers, which is similar to that of a general hydrogel contact lens.27 In addition, the Dk increased proportionally with increasing EWC. The structurally colored contact lens sample C had an EWC of approximately 40.14% and a Dk of approximately 11.6 barrers. Slight differences were found between the EWC and Dk of samples B and C. To confirm this result, we measured the Dk of five random samplings from samples B and C. The findings were measured and confirmed by the Taiwan Food and Drug Administration. The measured results showed that the true percent relative error and the R2 of these samples were approximately 1% and over 0.99, respectively. Therefore, the Dk of the structurally colored contact lenses could not depend on the ϕsilica of the AAs. The viscosity of the silica-hydrogel suspensions depended on the ϕsilica concentration. Silica-hydrogel suspensions with ϕsilica of over 30% were not used to prepare the samples because of the higher viscosity. Hence, ϕsilica of 30% is the optimal parameter for the silica-hydrogel suspensions in this study. The hydrogel contact lenses approached the point where the oxygen needs of the cornea would be satisfied.30 These results indicate the good performance of the developed structurally colored contact lenses for daily disposable contact lenses.
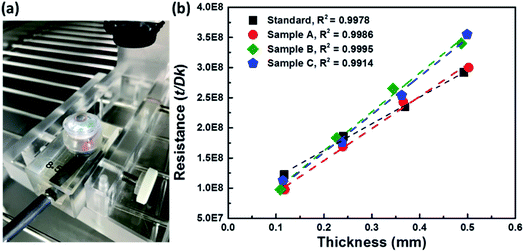 |
| Fig. 6 (a) Polarographic electrode cell for measuring the Dk of sample C. (b) Corrected resistance versus thickness plots for all contact lens samples. The plots are closely approximated by a line (R2 > 0.99). | |
3.4 Contact angle measurements of AR structurally colored contact lenses
The standard sample was sticky compared with the structurally colored contact lenses (samples A–C). Therefore, we inferred that the surface roughness of the contact lenses affected their hydrophilicity, and this supposition is based on previous reports.13,14 Fig. 7(a) and (b) show the variation in the water CA of the contact lenses with and without AA nanostructures. The CA of the standard contact lens was approximately 129.05° with a hydrophobic surface, which was reduced to nearly 54.42° with ϕsilica = 30% (sample C) with a hydrophilic surface. These results indicate that the AA-induced hydrophilic structurally colored contact lenses were successfully obtained in the interfaces between each silica nanosphere. Moreover, the CA on sample A was approximately 93.77° and reduced to approximately 54.42° when the ϕsilica was increased [Fig. 7(c)]. The CA showed decreased dependence on the ϕsilica of AAs, because the surface roughness of AAs was greater than that of smooth contact lenses. This result was attributed to the AA surface roughness and this finding is consistent with the previously reported resin films with AA nanostructures.13,14 Finally, we could not evaluate the practical usage of these contact lenses because these soft contact lenses are medical devices. Consequently, they must undergo human clinical trials and be approved by the United States Food and Drug Administration before marketing. Therefore, this study only proposed a novel method and demonstrated that contact lenses with AA nanostructures present improved hydrophilicity, are non-sticky, and block UV and blue light without further processing.
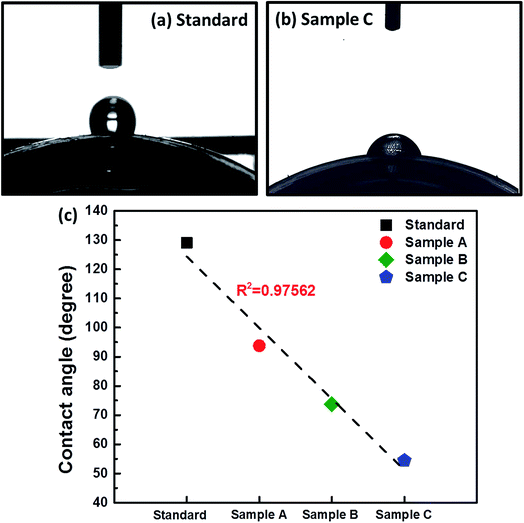 |
| Fig. 7 Optical photographs of (a) standard and (b) sample C for CA measurements. (c) CA versus samples plot closely approximated by a line (R2 > 0.97). | |
4. Conclusions
In summary, we presented novel AR structurally colored contact lenses with AA nanostructures. The proposed contact lenses not only blocked UV and blue light under sunlight but also avoided the risk of harm posed by commercial contact lenses colored with chemical dyes. The AR structurally colored contact lenses exhibited homogeneous bluish structural colors and high transmittance of over 90%. Moreover, the proposed contact lenses blocked SWB transmittance by approximately 20%. Adjusting the ϕsilica in the hydrogel contact lenses effectively controlled the structural colors, transmittance, EWC, Dk, and CA. The EWC and Dk of the structurally colored contact lenses were in the range of 40.14–45.65% and 11.6–13.7 barrers, respectively, indicating that the structurally colored contact lenses satisfied the requirements for daily disposable contact lenses. Therefore, the AR structurally colored contact lenses are very promising as substitutes for commercial colored contact lenses using chemical dyes.
Conflicts of interest
There are no conflicts to declare.
Acknowledgements
This work is supported by the Ministry of Science and Technology (MOST) in Taiwan under contract numbers MOST105-2221-E-035-041 and MOST106-2221-E-035-076. The authors appreciate the Precision Instrument Support Center of Feng Chia University for providing the fabrication and measurement facilities.
References
- E. Jang, S. Jun, H. Jang, J. Lim, B. Kim and Y. Kim, White-Light-Emitting Diodes with Quantum Dot Color Converters for Display Backlights, Adv. Mater., 2010, 22, 3076 CrossRef CAS PubMed.
- R. J. Xie, N. Hirosaki and T. Takeda, Wide Color Gamut Backlight for Liquid Crystal Displays Using Three-Band Phosphor-Converted White Light-Emitting Diodes, Appl. Phys. Express, 2009, 2, 022401 CrossRef.
- S. Ye, F. Xiao, Y. X. Pan, Y. Y. Ma and Q. Y. Zhang, Phosphors in phosphor-converted white light-emitting diodes: recent advances in materials, techniques and properties, Mater. Sci. Eng. R Rep, 2010, 71, 1 CrossRef.
- G. C. Brainard, J. P. Hanifin, J. M. Greeson, B. Byrne, G. Glickman, E. Gerner and M. D. Rollag, Action Spectrum for Melatonin Regulation in Humans, J. Neurosci., 2001, 21, 6405 CAS.
- S. M. Pauley, Lighting for the human circadian clock: recent research indicates that lighting has become a public health issue, Med. Hypotheses, 2004, 63, 588 CrossRef PubMed.
- J. P. Hanifin, K. T. Stewart, P. Smith, R. Tanner, M. Rollag and G. C. Brainard, High-intensity red light suppresses melatonin, Chronobiol. Int., 2006, 23, 251 CrossRef CAS PubMed.
- E. Chamorro, C. B. Arias, M. J. P. Carrasco, J. M. d. Luna, D. Vazquez and C. S. Ramos, Effects of Light-emitting Diode Radiations on Human Retinal Pigment Epithelial Cells In Vitro, Photochem. Photobiol., 2013, 89, 468 CrossRef CAS PubMed.
- U. Wihlmarka, A. Wrigstada, K. Robergb, S. E. G. Nilssona and U. T. Brunkb, Lipofuscin Accumulation in Cultured Retinal Pigment Epithelial Cells Causes Enhanced Sensitivity to Blue Light Irradiation, Free Radical Biol. Med., 1997, 22, 1229 CrossRef.
-
(a) H. S. Lee, T. S. Shim, H. Hwang, S. M. Yang and S. H. Kim, Colloidal Photonic Crystals toward Structural Color Palettes for Security Materials, Chem. Mater., 2013, 25, 2684 CrossRef CAS;
(b) Y. Takeoka, M. Honda, T. Seki, M. Ishii and H. Nakamura, Structural Colored Liquid Membrane without Angle Dependence, ACS Appl. Mater. Interfaces, 2009, 1, 982 CrossRef CAS PubMed.
- F. Li, B. Tang, S. Wu and S. Zhang, Facile Synthesis of Monodispersed Polysulfide Spheres for Building Structural Colors with High Color Visibility and Broad Viewing Angle, Small, 2017, 13, 1602565 CrossRef PubMed.
- Y. Takeoka, S. Yoshioka, A. Takano, S. Arai, K. Nueangnoraj, H. Nishihara, M. Teshima, Y. Ohtsuka and T. Seki, Production of Colored Pigments with Amorphous Arrays of Black and White Colloidal Particles, Angew. Chem., Int. Ed., 2013, 52, 7261 CrossRef CAS PubMed.
-
(a) D. Ge, L. Yang, G. Wu and S. Yang, Angle-independent colours from spray coated quasi-amorphous arrays of nanoparticles: combination of constructive interference and Rayleigh scattering, J. Mater. Chem. C, 2014, 2, 4395 RSC;
(b) K. Katagiri, Y. Tanaka, K. Uemura, K. Inumaru, T. Seki and Y. Takeoka, Structural color coating films composed of an amorphous array of colloidal particles via electrophoretic deposition, NPG Asia Mater., 2017, 9, e355 CrossRef CAS.
- C. F. Lai, Y. C. Wang and H. C. Hsu, High transparency in the structural color resin films through quasi-amorphous arrays of colloidal silica nanospheres, J. Mater. Chem. C, 2016, 4, 398 RSC.
- C. F. Lai, J. S. Li and C. W. Shen, High-Efficiency Robust Free-Standing Composited Phosphor Films with 2D and 3D Nanostructures for High-Power Remote White LEDs, ACS Appl. Mater. Interfaces, 2017, 9, 4851 CAS.
- Z. Xie, L. Li, P. Liu, F. Zheng, L. Guo, Y. Zhao, L. Jin, T. Li and Z. Gu, Self-assembled coffee-ring colloidal crystals for structurally colored contact lenses, Small, 2015, 11, 926 CrossRef CAS PubMed.
- P. Liu, Z. Xie, F. Zheng, Y. Zhao and Z. Gu, Surfactant-free HEMA crystal colloidal paint for structural color contact lens, J. Mater. Chem. B, 2016, 4, 5222 RSC.
- P. C. Nicolson and J. Vogt, Soft contact lens polymers: an evolution, Biomaterials, 2001, 22, 3273 CrossRef CAS PubMed.
- Y. C. Lai, Effect of crosslinkers on photocopolymerization of N-vinylpyrrolidone and methacrylates to give hydrogels, J. Appl. Polym. Sci., 1997, 66, 1475 CrossRef CAS.
- Y. C. Lai, A novel crosslinker for UV copolymerization of N-vinyl pyrrolidone and methacrylates to give hydrogels, J. Polym. Sci., Part A: Polym. Chem., 1997, 35, 1039 CrossRef CAS.
- L. M. Muratore and T. P. Davis, Self-reinforcing hydrogels comprised of hydrophobic methyl methacrylate macromers copolymerized with N,N-dimethylacrylamide, J. Polym. Sci., Part A: Polym. Chem., 2000, 38, 810 CrossRef CAS.
- T. H. Kim and A. Y. Sung, Preparation and Physical Properties of Hydrogle Lens Containing N,N-Dimethylacrylamide, J. Korean Chem. Soc., 1994, 54, 761 CrossRef.
- A. Y. Sung and T. H. Kim, Optical application of poly(HEMA-co-MMA) containing silver nanoparticles and N,N-dimethylacrylamide, Korean J. Chem. Eng., 2012, 29, 686 CrossRef CAS.
- T. S. Perova, J. K. Vij and H. Xu, Fourier transform infrared study of poly(2-hydroxyethyl methacrylate) PHEMA, Colloid Polym. Sci., 1997, 275, 323 CAS.
- K. Zhang, L. M. Gan, C. H. Chew and L. H. Gan, Silica from hydrolysis and condensation of sodium metasilicate in bicontinuous microemulsions, Mater. Chem. Phys., 1997, 47, 164 CrossRef CAS.
- Y. L. Ko, H. P. Tsai, K. Y. Lin, Y. C. Chen and H. Yang, Reusable macroporous photonic crystal-based ethanol vapor detectors by doctor blade coating, J. Colloid Interface Sci., 2017, 487, 360 CrossRef CAS PubMed.
- N. Efron and N. A. Brennan, The clinical relevance of hydrogel lens water content, International Contact Lens Clinic, 1987, 10, 9 Search PubMed.
- P. C. Nicolson and J. Vogt, Soft contact lens polymers: an evolution, Biomaterials, 2001, 22, 3273 CrossRef CAS PubMed.
- W. J. Benjamin and Q. A. Cappelli, Oxygen Permeability (Dk) of Thirty-Seven Rigid Contact Lens Materials, Optom. Vis. Sci, 2002, 79, 103 CrossRef PubMed.
- M. D. Young and W. J. Benjamin, Oxygen Permeability of the Hypertransmissible Contact Lenses, Eye Contact Lens, 2003, 29, S17 Search PubMed.
- N. Efron, N. A. Brennan and B. A. Holden, The oxygen permeability of rigid gas permeable contact lens materials: reaching a concensus, Int. Contact Lens Clin., 1987, 10, 62 Search PubMed.
Footnotes |
† Electronic supplementary information (ESI) available. See DOI: 10.1039/c7ra12753g |
‡ All authors contributed equally to this work. |
|
This journal is © The Royal Society of Chemistry 2018 |
Click here to see how this site uses Cookies. View our privacy policy here.