DOI:
10.1039/C8QM00301G
(Research Article)
Mater. Chem. Front., 2018,
2, 2081-2090
Biomimetic dendrimers for mineralization: rare fibrous amorphous calcium carbonate (ACC) and branch-and-bud ACC–vaterite polymorphs†
Received
18th June 2018
, Accepted 10th September 2018
First published on 10th September 2018
Abstract
Inorganic mineralization plays a vital role in numerous biological, chemical, and pharmaceutical processes, ranging from hard-organ development through the so-called biomineralization to the synthesis of functional hybrid materials. Inspired by living organisms, a great effort has been devoted to directing the mineralization pathways toward obtaining rare polymorphs. We have synthesized a family of dendrimers based on tetraethylene glycol (TEG) backbone, symmetrically decorated with phosphonate groups on their surfaces, to engineer the interactions among precipitating ions and stabilize early-stage polymorphs under ambient conditions. In a model system, the mineralization of calcium carbonate was templated with a ppm level of TEG-based dendrimers, resulting in the first experimental observation of unique branch-and-bud CaCO3 structures, wherein amorphous calcium carbonate (ACC), the branch, was decorated with vaterite (buds). Favorable directional growth of ACC, stabilization of vaterite, and secondary nucleation were identified as key mineralization phenomena. Dendrimer generation, regulating molecular flexibility and functional group density, strongly affected the biomimetic mineralization time scale. The outcome of this research may have direct applications in designing hybrid inorganic–organic fibers, ACC and vaterite production, and scale inhibition in water-based industries.
Introduction
Inspired by nature, the recent decade has witnessed a great interest in taking control over the interactions between precipitating ions to design superior inorganic crystals and hybrid organic–inorganic composites.1–3 The precipitation of inorganic salts is typically affected by biological macromolecules through the so-called biomineralization process, which, opposite to the conventional crystallization pathways, involves intracellular local templating of ions to stabilize disordered, otherwise unstable polymorphs.4 Ions are taken up from body fluids or sea water, transported into the cell through endocytosis and/or ion channels, where early-stage amorphous polymorphs of an inorganic salt are formed via complex, yet to be understood, pathways.4 The disordered polymorphs are then transported to a crystallization front to form a desired polymorph inside the cell or at the cell surface. Some examples of biomineralization encompass the secondary nucleation of amorphous calcium carbonate in sea urchin larval spicule,5 including ∼0.05% organic macromolecules (amino acids),4,6 and iridescence protective layers of fish, beetles, mollusks, and spiders involving ordered arrays of guanine crystals.7–9
Common biominerals are (i) carbonates in invertebrates, (ii) calcium, carbonates, and phosphates in vertebrates, and (iii) silicates in algae10 among which calcium phosphate and calcium carbonate have been identified as two of the most abundant minerals. Calcium phosphate plays a key role in the structure of mammalian hard tissues, such as teeth and bones, typically as apatitic compounds.11 There have been several efforts in mimicking the in vivo mineralization of calcium phosphate using macromolecules for bone and dental tissue engineering applications. Carboxylate-modified poly(amidoamine) (PAMAM) dendrimers, benefitting from a controlled steric structure and monodispersed molecular weight, have been able to mimic the role of noncollagenous proteins, enabling the hierarchical intrafibrillar biomineralization of calcium phosphate within collagen fibrils.12 Phosphorylated dendronized poly(amidoamine) with a worm-like morphology has been used to mimic the mineralization of hydroxylapatite (HA), substituting noncollagenous proteins.13 Other examples of macromolecular templates for the biomimetic mineralization of HA may be found in the literature.14
Mechanistic studies on the crystallization pathways of calcium carbonate suggest that stable crystalline polymorphs (aragonite and calcite) are typically formed from an amorphous, highly unstable precursor called amorphous calcium carbonate (ACC), which is an intriguing, difficult-to-stabilize intermediate.15 Emerging applications of ACC encompass on-demand soluble ion carriers to treat ion (e.g., Ca2+) deficiency, hard tissue engineering,16–18 macromolecule (protein and peptide) and drug delivery platforms,19–23 isotropic structural fillers,24,25 and precursors26 for engineered crystalline calcium carbonate.27 The second most unstable polymorph of calcium carbonate under ambient conditions is vaterite;28 a well-known, unstable crystalline form of CaCO3 with a broad range of applications, such as personal care and biomedicine.29–32
Several strategies have been adopted to mimic the biomineralization of highly-unstable polymorphs of calcium carbonate.33–36 Inspired by biological pathways for regulating CaCO3 crystallization,37 macromolecules with negatively-charged functional groups (carboxylic acid, sulfate, phosphate),38–47 phospholipids,48–50 and hydrogels51,52 have been adopted for biomimetic mineralization. Among the designer macromolecules, phosphorous-based species, e.g., phosphates, mimicking the functionality of intracellular ion carrier phospholipid vesicles, benefit from stronger Ca2+ binding than other anionic functional groups, such as carboxylates and sulfates (e.g., Ksp,Ca3(PO4)2 ∼ O(10−23) ≪ Ksp,CaCO3 ∼ O(10−9) ≪ Ksp,CaSO4 ∼ O(10−4)).53–56 Phosphorus-containing functional groups, such as phosphates and phosphonates, have been used to obtain a variety of rare calcium carbonate polymorphs and morphologies, including spiral calcite,56 pancake-like self-stacked calcite,57 mushroom-like CaCO3,58 spherical56 and flower-like vaterite,59,60 and amorphous calcium carbonate nanospheres.61 Rapid transition of ACC to stable polymorphs, i.e., aragonite and calcite, has limited the mechanistic studies of CaCO3 morphogenesis and prevented the formation of large-scale ACC and vaterite. To this end, biomimetic additives have been designed for the efficient stabilization of rare inorganic polymorphs.
We endeavored to design dendrimers built from a tetraethylene glycol (TEG) core and decorated with terminal phosphonated groups to take precise control over the functional group density, molecular structure, and flexibility. Biomimetic mineralization of calcium carbonate was conducted using various generations of the dendrimers (G0–G2), and the tradeoff between the functional group density and molecular flexibility in mineralization efficiency was investigated. For the first time, time-dependent morphogenesis of shapeless ACC to fibrous ACC and vaterite was observed.
Materials and methods
Materials
All chemicals and solvents were used as purchased without any further purification except for Ts-Cl which was recrystallized from CHCl3 prior to its use. NMR spectral acquisitions were carried out on Mercury instruments (Varian, Palo Alto, CA, USA) using a 5 mm Smart Probe, operated via VNMRJ (Chempack 5) software. An aqueous solution of phosphoric acid (85 wt%) was used as a reference for 31P-NMR. Full details of dendrimer synthesis are provided in the ESI.† The chemical shifts (ppm) in NMR spectroscopy are reported, which are related to tetramethylsilane (TMS) as an internal standard and solvents as references (1H, 13C); CDCl3 (7.26, 77.16), (CD3)2SO (2.50, 39.52), D2O (4.79, —), CD3OD (3.31, 49.00).
Methods
Mineralization experiments
15 mL of a calcium chloride solution (20 mM) was mixed with 15 mL of a sodium bicarbonate (20 mM) solution including a desired amount of the dendrimer, followed by mixing vigorously for 1 min and incubation at rest (room temperature with no agitation) on an acid-cleaned cover glass placed inside a sealed glass vial. To stop the mineralization reactions, the supernatant was decanted, and the precipitate was thoroughly washed with milli-Q water three times and dried at 50 °C in an oven for at least 24 h.
Scanning electron microscopy (SEM)
SEM imaging was performed on dried specimens, which were directly transferred from the mineralization vials onto an adhesive carbon pad mounted on a sample holder, followed by coating with a 4 nm-thick layer of Pt using a vacuum sputtering instrument (Leica Microsystems EM ACE600 High Resolution Coater). SEM (FEI Inspect F-50 FE-SEM with energy dispersive spectroscopic equipment EDAX Octane Super 60 mm2 SDD and TEAM EDS Analysis System) was used for imaging and elemental analysis.
Focused ion beam (FIB)
A transmission electron microscopy (TEM) lamella was prepared using the FEI Helios NanoLab 660 DualBeam system. Final thinning was performed at 30 kV and 2.5 nA to acquire a thin sample (<100 nm). We investigated the local morphology, crystal orientation and packing, and chemical composition of various polymorphs by transmission electron microscopy (TEM) imaging, selected area electron diffraction (SAED), and energy dispersion spectroscopy (EDS), respectively.
TEM, SAED, and EDS
The FIB-prepared thin lamella was mounted on a copper grid and examined with TEM (FEI Tecnai G2 F20 200 kV Cryo-STEM with Gatan Ultrascan 4000 4k × 4k CCD Camera System Model 895 and EDAX Octane T Ultra W/Apollo XLT2 SDD and TEAM EDS Analysis System). To acquire the electron diffraction pattern, SAED (with a 10 μm selective aperture) was conducted on various regions of the lamella (∼270 nm-diameter circles).
ζ-Potential and hydrodynamic size
Electrophoretic mobility (based on the electrophoretic light scattering experiments in the universal dip cell kit using Zetasizer NanoZS equipped with a 4 mW–633 nm He–Ne solid-state laser, Malvern Instruments, UK) yielded the ζ-potential of dendrimers, which was used to study their complex formation with Ca2+. Dynamic light scattering experiments were conducted in disposable cuvettes using the same instrument to measure the hydrodynamic size.
Electrochemical deposition of CaCO3 through chronoamperometry
Calcium carbonate was deposited on a hydrochloric acid-cleaned gold electrode (fixed-disk rotating ring disk electrode, diameter ∼ 5 mm, with polyether ether ketone shroud OD ∼ 15 mm, Pine Research Instrumentation, U.S.A.). The electrode was rotated at a constant speed (700 rpm) in a saturated solution containing calcium chloride (∼6 mM) and sodium bicarbonate (∼10.7 mM) while applying a constant voltage (−1 V per standard calomel electrode SCE) using a WaveDriver 10 potentiostat, controlled by AfterMath Data Organizer Software (both purchased from Pine Research Instrumentation, U.S.A.). Complete CaCO3 deposition was obtained when the Cottrell current I ∼ 0.
Results and discussion
Dendrimers are monodisperse hyperbranched macromolecules that find applications in a wide variety of areas.62 We were interested in such architectures that could be synthesized using a simple and versatile methodology, and will incorporate easily accessible starting materials and robust click chemistry in their build-up and functionalization.63 TEG was chosen as the core and branching entity due to its water solubility and low cost. Using a branching AB3 system that facilitated rapid increase of the number of surface groups at the periphery, we synthesized generations 0, 1, and 2 of TEG-based dendrimers containing 6, 18, and 54 phosphonate groups at their periphery, respectively, which had an open and flexible architecture. Structures of the dendrimers employed in this study are shown in Schemes 1–3 (also see ESI†), and their properties are summarized in Table 1. By increasing the dendrimer generation from G0 to G2, the molecular weight increases by a factor of ∼3.7 and 3.2, respectively, and the functional group molar content increases by a factor of 3, resulting in an almost constant ratio of [PO32−]/dendrimer mass ∼2.5 ± 0.3 mmol g−1. Fixing this ratio is essential in performing mineralization experiments because typically the performance of an additive is evaluated based on its mass. This family of dendrimers enabled us to fine tune this ratio. Accordingly, in any added amount of the dendrimers, the ratio between the anionic functional groups to the calcium ion concentrations remains almost constant [PO32−]/[Ca2+] ∼ 0.1 ± 0.01 mmol mol−1.
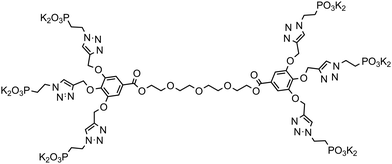 |
| Scheme 1 TEG-G0 dendrimer with 6 phosphonate groups. | |
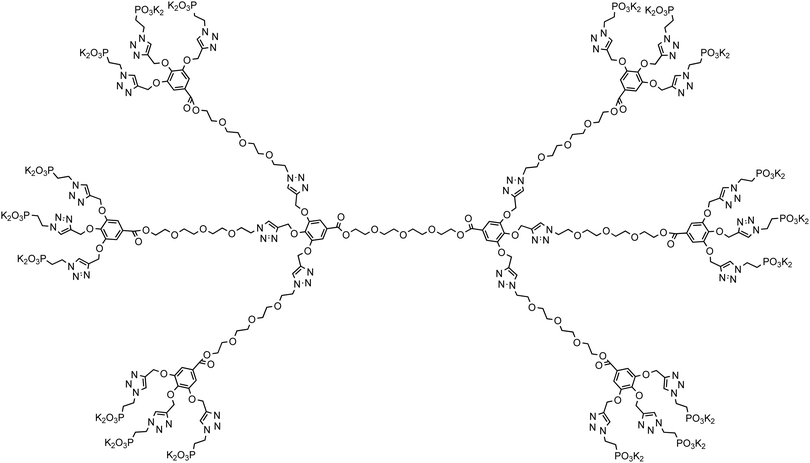 |
| Scheme 2 TEG-G1 dendrimer with 18 phosphonate groups. | |
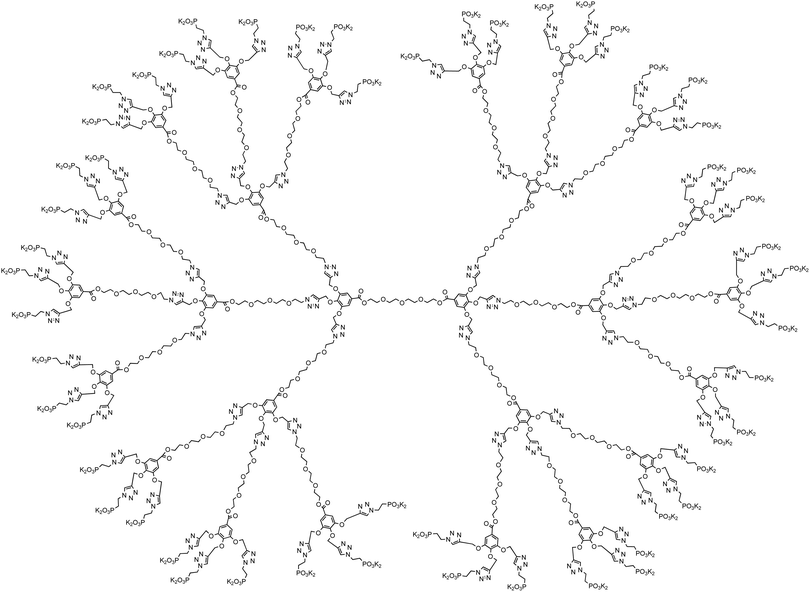 |
| Scheme 3 TEG-G2 dendrimer with 54 phosphonate groups. | |
Table 1 Molecular and colloidal characteristics of phosphonated dendrimers
Dendrimer |
TEG-G0 |
TEG-G1 |
TEG-G2 |
M
w (Da) |
2087.89 |
7721.99 |
24624.57 |
[PO32−] (mol/mol macromolecule) |
6 |
18 |
54 |
[PO32−]/[Ca2+] (mol/mol) |
0.115 × 10−3 |
0.094 × 10−3 |
0.092 × 10−3 |
Hydrodynamic size (MilliQ water) (nm) |
164 ± 56 |
249 ± 14 |
252 ± 8 |
Hydrodynamic size (10 mM CaCl2) (nm) |
206 ± 38 |
278 ± 58 |
N/A |
ζ-Potential in MilliQ water (mV) |
−52 ± 3 |
−38 ± 2 |
−36 ± 1 |
ζ-Potential in 10 mM CaCl2 (mV) |
−8.5 ± 0.4 |
−7 ± 0.5 |
−5.7 ± 0.2 |
The dendrimers readily disperse in water, forming stable colloid-like amorphous structures, presented in Fig. 1, with hydrodynamic sizes increasing by increasing the generation number. The transmission electron microscopy images suggest that the dendrimers form branched needle-like structures with lengths ∼100–200 nm. Owing to their anionic functional groups, the ζ-potentials of the dendrimers are negative in water, which approach zero by adding Ca2+, attesting to a strong binding of the cation to the dendrimers. Note that the dendrimers are highly charged and should repel each other. One possibility for the dendrimer aggregate formation is that the pKa of the carboxyl groups is affected by neighboring carboxyl groups, as e.g., in self-assembled monolayers (SAMs), where the pKa of the first carboxyl group is 4 and the neighboring one is 7–10,64 leading to an internal H-bond. A similar phenomenon has been observed in styrene maleic anhydride alternating copolymers.66 Accordingly, H-bonds may possibly form between neighboring dendrimers. Reflected in the hydrodynamic size of the dendrimers, no significant aggregation of the dendrimers is noticed upon the addition of calcium ions.
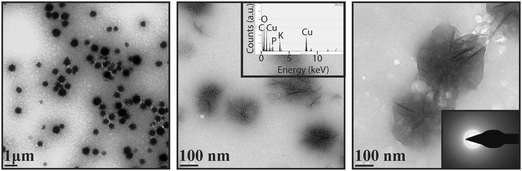 |
| Fig. 1 Transmission electron microscopy (TEM) images of the TEG-based dendrimers (TEG-G2) show clusters of branched needle-shaped amorphous aggregates, mainly made up of C, O, and P (from energy dispersion spectroscopy (EDS), inset of middle panel). Note that copper contribution to the elemental analysis is due to the TEM grid. | |
When these dendrimers are added to a carbonate solution followed by the addition of calcium ions, within a few minutes (e.g., 15 min), nanoscale amorphous calcium carbonate particles with sizes less than 100 nm are formed (Fig. 2A). Smeared SAED pattern in the inset of Fig. 2A does not show any evidence of a crystalline polymorph. The ACC particles undergo a time-dependent adhesion and growth (Fig. 2B) within the next 15 min to form shapeless amorphous clusters. In 1 hour, ACC is transformed to particles with mainly branched structures (Fig. 2C and D), which then partially transform to the least thermodynamically stable crystalline CaCO3, i.e., vaterite (Fig. 2F and G). The transition is shown in Fig. 2E, where the morphogenesis of ACC to vaterite is captured by stopping the crystallization reaction through removing the precipitating ions (see Materials and methods). The ACC particles further grow as branched structures (Fig. 2H, J, and K) in ∼16.5 h. Within this time scale, the vaterite particles grow as connected flakes (Fig. 2I). After a week of mineralization, branched ACC particles grow significantly and connect the vaterite features (Fig. 2L and M). These structures resemble branches of trees decorated with buds. Interestingly, the ACC fibers grow in a 3D space and do not remain in the plane of early-stage crystals, i.e., they partially detach from the substrate. Note that the unidirectional growth of calcium carbonate may be a result of heterogenous adsorption of the dendrimers to the growing crystal surfaces. Thermodynamically, dendrimer binding to the crystal faces is likely to be more favorable than to the edges. This is explained by the larger area of the faces, permitting a higher conformational freedom. Accordingly, the edges grow quicker than the faces, yielding the fibrous structures. Despite possible structural defects in high-generation dendrimers, the mineralization outcome is regulated by the collective performance of a large number of dendrimers, thus, it has been reproducible. Furthermore, the structural defects in low-generation dendrimers are typically less pronounced.
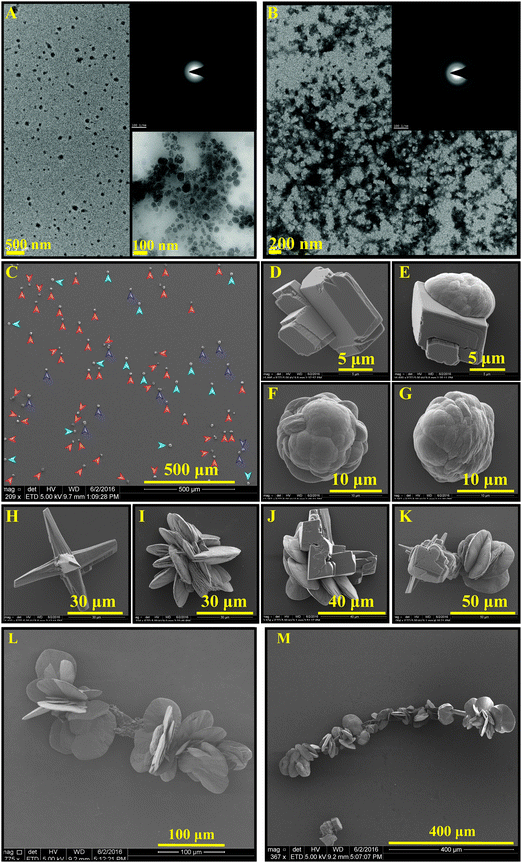 |
| Fig. 2 Early stages of the biomimetic mineralization of calcium carbonate in a solution consisting of CaCl2 (10 mM), NaHCO3 (10 mM), and TEG-G2 dendrimer (∼13.3 ppm), incubated for 15 min (A, TEM image with a SAED pattern inset) and 30 min (B, TEM image with a SAED pattern inset) result in the formation of ACC. After 1 h (C, SEM image), a mixture of stabilized individual ACC particles (red arrows in C, shown in D), ACC–vaterite polymorphs (purple arrows in C, shown in E), and vaterite particles (blue arrows in C, shown in F and G) are yielded. Crystallization for 16.5 h, increases both ACC (H) and vaterite particle (I) sizes while promoting a branched growth of star-like ACC particles (H, J, and K), which then connect the vaterite polymorphs at extended times (L and M, 185 h). Further crystallization on the fibrillar ACC may decorate it with vaterite and/or calcite features. | |
The mineralization experiment with a lower generation dendrimer (TEG-G1, Fig. 3) results in similar branched structures, decorated with a different shape of vaterite particles. Spiral vaterite particles (Fig. 3B–E) are the dominant morphology, decorating the long ACC fibers. Importantly, compared to TEG-G2, the mineralization of calcium carbonate is significantly delayed: no CaCO3 particles are observed within the first hours of experiments (Fig. 3A), and it takes more than 2 weeks to obtain branch-and-bud features. Such an improved inhibitory effect may be a result of enhanced molecular flexibility of G1 dendrimers. Similar results were obtained using the generation zero dendrimers with a time scale falling between G1 and G2 dendrimers. Accordingly, it can be concluded that the performance of the TEG-based dendrimers in stabilizing the early stages of calcium carbonate mineralization is regulated based on a tradeoff between the anionic functional group density and molecular flexibility. An example of a similar trend is the inability of rigid carboxylated biopolymers (e.g., carboxymethylated cellulose) in stabilizing ACC and vaterite compared to more flexible analogues, such as dicarboxylated cellulose.65 Increased molecular flexibility of G1 dendrimers, compared to G2, may also promote the formation of spiral vaterite clusters, an observation that warrants further in situ characterizations, such as liquid-phase TEM for mechanistic studies.
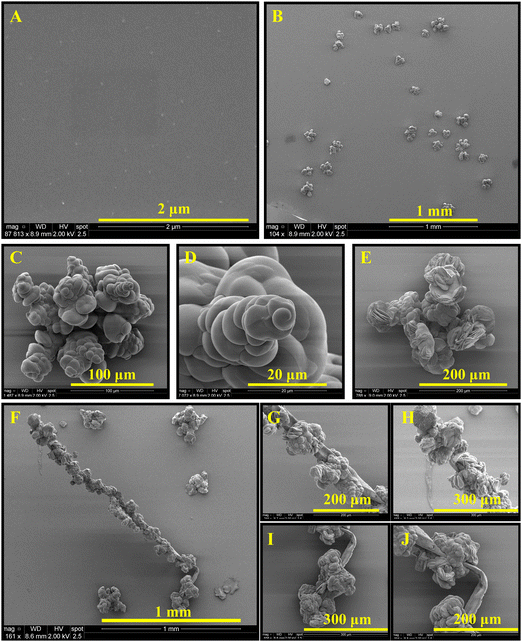 |
| Fig. 3 Biomimetic mineralization of calcium carbonate in a solution containing CaCl2 (10 mM), NaHCO3 (10 mM), and TEG-G1 dendrimer (∼13.3 ppm), incubated for 1 h (A), 24 h (B–D), 7 d (E), and 14 d (F–J). No crystal is observed at short times, confirming a much significant inhibitory effect of this dendrimer compared to its second generation. It takes a relatively longer time to develop vaterite structures (B–D), and the particles seem to have spiral orientation, which then transform to vaterite sheets (E). Unidirectional growth of crystals results in elongated features (F, branches), accommodating vaterite particles (G–J, buds). Note that the ACC fibers may detach from the substrate. | |
Independent of the dendrimer generation, at longer times (e.g., 30 d, Fig. 4), the fibrous ACC is covered with various polymorphs of CaCO3 as a result of extended nucleation and growth. Features resembling radially-stacked corn dog (pogo)-like calcium carbonate are formed through mineralization around the fibers. Secondary nucleation promotes the occurrence of well-known hexagonal vaterite particles on the fibers (Fig. 4C, E, and F). To shed light on the crystalline structure of dendrimer-templated CaCO3 polymorphs, a cluster of precipitated minerals was selected (Fig. 5A) and processed through focused ion beam milling (Fig. 5B and C) to obtain a thin lamella (Fig. 5D) on which TEM-based analyses, namely SAED and EDS were conducted. Long branched fibers, shown with numbers 1 and 2 in Fig. 5 have an amorphous structure, which is reflected in the smeared SAED patterns. The flake-like structures are confirmed to be vaterite, and the corn dog (pogo)-like feature is made up of vaterite, which is decorated with calcite spikes, as a result of morphogenesis at the extended mineralization time.
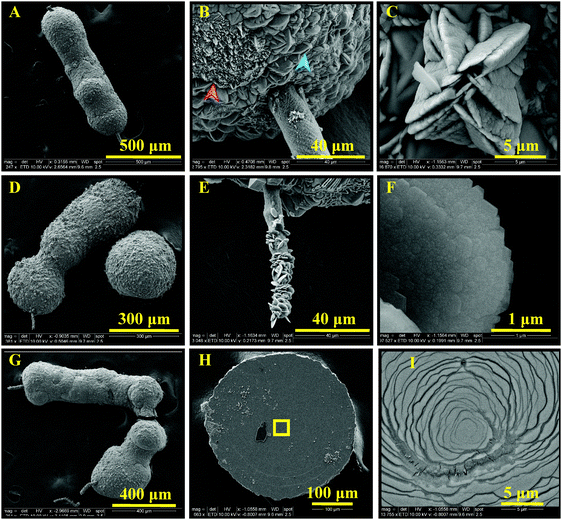 |
| Fig. 4 Long-time (30 d) biomimetic mineralization of calcium carbonate with 10 mM CaCl2, 10 mM NaHCO3, and TEG-G1. Elongated ACC fibers (A, D, and G) are almost fully covered with vaterite (B, blue arrow, transformed to calcite, red arrow) in some regions, forming corn dog (pogo)-like structures. The tail (B and E) is also covered with well-known hexagonal vaterite structures (C and F), possibly as a result of secondary nucleation. Layered formation of CaCO3 is evident (H, magnified in I). Dendritic CaCO3 features are also observed on the surface, which may extend as fibrous structures (ESI,† Fig. S1). | |
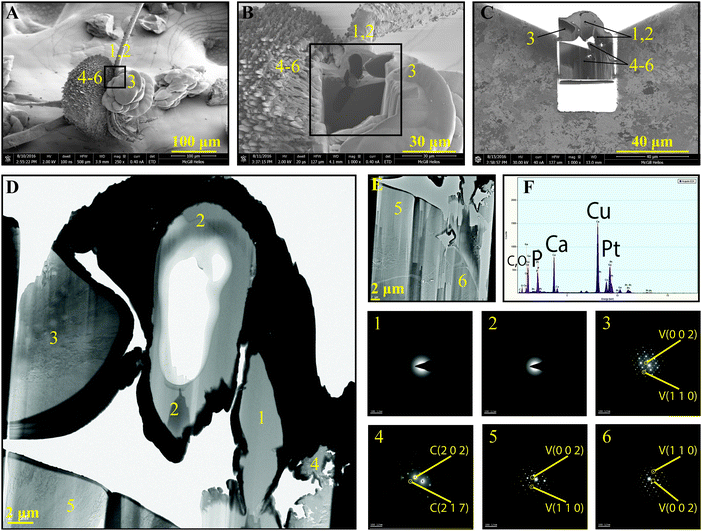 |
| Fig. 5 A well-grown CaCO3 cluster, including all possible polymorphs of dendrimer-assisted biomimetic mineralization was selected (A, SEM image) and coated with a thin layer of Pt (B), followed by focused ion beam (FIB) milling to obtain a cross-sectional view of features marked with numbers 1–6 (C). The lamella was transferred to a TEM grid (C), and the structure and composition of various regions were evaluated (D–F). Regions 1 and 2 present the structure of fibers, 3 shows the flake-like calcium carbonate, 4 presents the spikes on the outer surface of the corn dog (pogo)-like features, and 5 and 6 show the inner structure of the corn dog (pogo)-like features. The SAED patterns attest to an amorphous structure for the fibers, vaterite for the flakes, and calcite for the spikes formed on the corn dog (pogo)-like features. The elemental analysis through EDS (F) shows Ca, C, O, and P as the main components of the polymorphs. | |
The mineralization time scales are compared in an accelerated CaCO3 precipitation experiment using chronoamperometry. Fig. 6 shows the Cottrell current versus time, indicating a complete precipitation of calcium carbonate in 30 min. This time is doubled by the addition of ∼9.7 ppm G2 dendrimer and more than tripled by the G0 dendrimer. G1 dendrimer results in an incomplete deposition of calcium carbonate on the electrode within ∼100 min, proving that the G1 dendrimer regulates the crystallization of calcium carbonate more efficiently, possibly due to a higher molecular flexibility than the G2 dendrimer. Note that, while the flexibility of G0 > G1 > G2, at a fixed mass of added dendrimers, the number of molecules per unit mass decreases by increasing the generation, while the anionic functional group density remains almost constant. In other words, by increasing the generation, the same number of phosphonates per gram of dendrimer is distributed among a lower number of dendrimers, which interact with the pre-nucleation clusters and nuclei. Understanding the molecular mechanisms of lattice formation under the effect of self-assembled dendrimers is not trivial and may require further theoretical investigations.
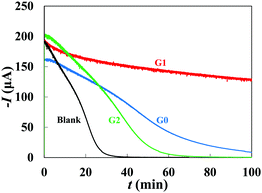 |
| Fig. 6 Cottrell current I versus electrochemically-induced precipitation time t of calcium carbonate on a rotating disk gold electrode in a solution containing 270 mg L−1 NaHCO3, 260 mg L−1 CaCl2·2H2O, and ∼9.7 ppm of dendrimers at T = 50 °C, obtained by chronoamperometry. In a dendrimer-free system, the electrode is completely coated in ∼30 min, shown by I ∼ 0. The precipitation time increases to ∼60 min for the G2 dendrimer, and the G1 dendrimer does not permit a full precipitation within ∼100 min. Superior flexibility of G1 over G2 increases the effect of dendrimers on the pre-nucleation clusters and nuclei, possibly as a result of better adsorption; whereas, G0 dendrimer, despite a higher flexibility than G1, bears one third of the phosphonate groups per each dendrimer molecule, decreasing its threshold effect. | |
The proposed mechanism of dendrimer-based biomimetic mineralization of calcium carbonate is presented in Fig. 7. The incubation of phosphonated dendrimers with precipitating calcium and carbonate ions results in the formation of shapeless amorphous calcium carbonate in the first few minutes. This unstable phase transforms into branched ACC with the possibility of further morphogenesis to vaterite particles. The branched ACC grows as amorphous fibers up to mm scale and becomes decorated by vaterite or, eventually, by calcite.
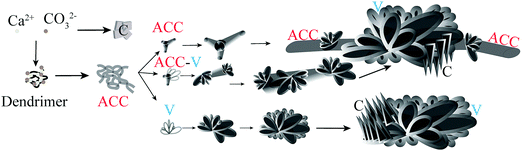 |
| Fig. 7 Proposed mechanism of dendrimer-assisted biomimetic mineralization of calcium carbonate: the self-assembly of mineralization precursors (dendrimer–Ca2+–CO32−) results in the formation of shapeless amorphous calcium carbonate followed by transformation to three combinations of polymorphs: fibrous amorphous calcium carbonate (ACC), ACC–vaterite, and vaterite (V). The ACC–vaterite structures are possibly obtained by the incomplete transformation of ACC to vaterite. The features grow by time, and individual vaterite and elongated ACC with vaterite decoration are obtained. Calcite (indicated by “C”) are formed at long enough times as a result of the partial morphogenesis of vaterite on the ACC fibers. The unique structures of branch-and-bud calcium carbonate are a result of such process. | |
Conclusions
Dendrimers with a tetraethylene glycol-based backbone and surface-decorated phosphonate groups present an intriguing platform to regulate mineralization of calcium carbonate, a model system for biomineralization. Three generations of dendrimers (G0–G2) were employed in this study, which provided an escalating number of peripheral anionic functional groups. These dendrimers permitted a constant functional group content [PO32−] per cation content [Ca2+] for all generations, enabling us to systemically study the effect of functional group density and molecular flexibility on the mineralization. Strong interaction between the calcium ions and the phosphonate groups resulted in the stabilization of the least thermodynamically stable polymorph of calcium carbonate, i.e., amorphous calcium carbonate (ACC) in a rare fibrous morphology. The time scale of ACC formation depended on the dendrimer generation; G1 delayed the mineralization the most and G2 the least. All dendrimers at ppm level yielded fibrous ACC decorated with vaterite, forming branch-and-bud features within weeks. TEG-based dendrimers may pave the way for the next-generation highly-efficient macromolecular systems for advanced nature-inspired hybrid inorganic–organic composites and water treatment applications.
Conflicts of interest
There are no conflicts to declare.
Acknowledgements
Financial support from Natural Sciences and Engineering Research Council (Canada), Fonds de Recherche du Québec Nature et technologies (FRQNT, Quebec, Canada), Centre for Self-Assembled Chemical Structures (CSACS), and Kemira is gratefully acknowledged. We would like to thank Prof. N. Tufenkji, McGill University for providing the Zetasizer instrument. The assistance of the Facility for Electron Microscopy Research (FEMR), McGill University, especially, Prof. H. Vali, Drs D. Liu and K. Sears, and L. Mongeon is much appreciated.
References
- U. G. K. Wegst, H. Bai, E. Saiz, A. P. Tomsia and R. O. Ritchie, Nat. Mater., 2015, 14, 23–36 CrossRef CAS PubMed.
- M. Darder, P. Aranda and E. Ruiz-Hitzky, Adv. Mater., 2007, 19, 1309–1319 CrossRef CAS.
- H.-B. Yao, H.-Y. Fang, X.-H. Wang and S.-H. Yu, Chem. Soc. Rev., 2011, 40, 3764–3785 RSC.
- S. Weiner and L. Addadi, Annu. Rev. Mater. Res., 2011, 41, 21–40 CrossRef CAS.
- Y. Politi, T. Arad, E. Klein, S. Weiner and L. Addadi, Science, 2004, 306, 1161–1164 CrossRef CAS PubMed.
- S. Raz, P. C. Hamilton, F. H. Wilt, S. Weiner and L. Addadi, Adv. Funct. Mater., 2003, 13, 480–486 CrossRef CAS.
- A. Levy-Lior, E. Shimoni, O. Schwartz, E. Gavish-Regev, D. Oron, G. Oxford, S. Weiner and L. Addadi, Adv. Funct. Mater., 2010, 20, 320–329 CrossRef CAS.
- D. Gur, B. A. Palmer, S. Weiner and L. Addadi, Adv. Funct. Mater., 2017, 27, 1603514 CrossRef.
- D. Gur, B. A. Palmer, B. Leshem, D. Oron, P. Fratzl, S. Weiner and L. Addadi, Angew. Chem., Int. Ed., 2015, 54, 12426–12430 CrossRef CAS PubMed.
- N. K. Dhami, M. S. Reddy and M. S. Mukherjee, Front. Microbiol., 2013, 4, 1–13 Search PubMed.
- S. V. Dorozhkin and M. Epple, Angew. Chem., Int. Ed., 2002, 41, 3130–3146 CrossRef CAS PubMed.
- J. Li, J. Yang, J. Li, L. Chen, K. Liang, W. Wu, X. Chen and J. Li, Biomaterials, 2013, 34, 6738–6747 CrossRef CAS PubMed.
- J. Xin, T. Chen, Z. Lin, P. Dong, H. Tan and J. Li, Chem. Commun., 2014, 50, 6491–6493 RSC.
- L. C. Palmer, C. J. Newcomb, S. R. Kaltz, E. D. Spoerke and S. I. Stupp, Chem. Rev., 2008, 108, 4754–4783 CrossRef CAS PubMed.
- L. Addadi, S. Raz and S. Weiner, Adv. Mater., 2003, 15, 959–970 CrossRef CAS.
- E. Tolba, W. E. G. Müller, B. M. Abd El-Hady, M. Neufurth, F. Wurm, S. Wang, H. C. Schroder and X. Wang, J. Mater. Chem. B, 2016, 4, 376–386 RSC.
- X. Wang, M. Ackermann, S. Wang, E. Tolba, M. Neufurth, Q. Feng, H. C. Schröder and W. E. G. Müller, Biomed. Mater., 2016, 11, 35005 CrossRef PubMed.
- K. Onuma and M. Iijima, Sci. Rep., 2017, 7, 2711 CrossRef PubMed.
- F. Tewes, O. L. Gobbo, C. Ehrhardt and A. M. Healy, ACS Appl. Mater. Interfaces, 2016, 8, 1164–1175 CrossRef CAS PubMed.
- V. Lauth, M. Maas and K. Rezwan, Mater. Sci. Eng., C, 2017, 78, 305–314 CrossRef CAS PubMed.
- C. Wang, S. Chen, Q. Yu, F. Hu and H. Yuan, J. Mater. Chem. B, 2017, 5, 2068–2073 RSC.
- Y. Zhao, Z. Luo, M. Li, Q. Qu, X. Ma, S.-H. Yu and Y. Zhao, Angew. Chem., Int. Ed., 2015, 54, 919–922 CrossRef CAS PubMed.
- S. Maleki Dizaj, M. Barzegar-Jalali, M. H. Zarrintan, K. Adibkia and F. Lotfipour, Expert Opin. Drug Delivery, 2015, 12, 1649–1660 CrossRef PubMed.
- Y. Oaki, S. Kajiyama, T. Nishimura, H. Imai and T. Kato, Adv. Mater., 2008, 20, 3633–3637 CrossRef CAS.
- T. Saito, Y. Oaki, T. Nishimura, A. Isogai and T. Kato, Mater. Horiz., 2014, 1, 321–325 RSC.
- T. Y.-J. Han and J. Aizenberg, Chem. Mater., 2008, 20, 1064–1068 CrossRef CAS.
- B. Cantaert, D. Kuo, S. Matsumura, T. Nishimura, T. Sakamoto and T. Kato, ChemPlusChem, 2017, 82, 107–120 CrossRef CAS.
- A. G. Christy, Cryst. Growth Des., 2017, 17, 3567–3578 CrossRef CAS.
- D. B. Trushina, T. V. Bukreeva, M. V. Kovalchuk and M. N. Antipina, Mater. Sci. Eng., C, 2015, 45, 644–658 CrossRef PubMed.
- B. V. Parakhonskiy, A. Haase and R. Antolini, Angew. Chem., Int. Ed., 2012, 51, 1195–1197 CrossRef CAS PubMed.
- M. S. Savelyeva, A. A. Abalymov, G. P. Lyubun, I. V. Vidyasheva, A. M. Yashchenok, T. E. L. Douglas, D. A. Gorin and B. V. Parakhonskiy, J. Biomed. Mater. Res., Part A, 2017, 105, 94–103 CrossRef CAS PubMed.
- J. R. Baylis, J. H. Yeon, M. H. Thomson, A. Kazerooni, X. Wang, A. E. S. John, E. B. Lim, D. Chien, A. Lee, J. Q. Zhang, J. M. Piret, L. S. Machan, T. F. Burke, N. J. White and C. J. Kastrup, Sci. Adv., 2015, 1–9 Search PubMed , asap.
- J. L. Arias and M. S. Fernández, Chem. Rev., 2008, 108, 4475–4482 CrossRef CAS PubMed.
- N. A. J. M. Sommerdijk and G. de With, Chem. Rev., 2008, 108, 4499–4550 CrossRef CAS PubMed.
- A. Dey, G. de With and N. A. J. M. Sommerdijk, Chem. Soc. Rev., 2010, 39, 397–409 RSC.
- C.-L. Chen, J. Qi, R. N. Zuckermann and J. J. DeYoreo, J. Am. Chem. Soc., 2011, 133, 5214–5217 CrossRef CAS PubMed.
- C. E. Killian and F. H. Wilt, Chem. Rev., 2008, 108, 4463–4474 CrossRef CAS PubMed.
- J. A. Lopez-Berganza and R. M. Espinosa-Marzal, Cryst. Growth Des., 2016, 16, 6186–6198 CrossRef CAS.
- K. Murai, T. Kinoshita, K. Nagata and M. Higuchi, Langmuir, 2016, 32, 9351–9359 CrossRef CAS PubMed.
- P. J. M. Smeets, K. R. Cho, R. G. E. Kempen, N. a. J. M. Sommerdijk and J. J. De Yoreo, Nat. Mater., 2015, 14, 394–399 CrossRef CAS PubMed.
- M. Cusack and A. Freer, Chem. Rev., 2008, 108, 4433–4454 CrossRef CAS PubMed.
- M. B. Dickerson, K. H. Sandhage and R. R. Naik, Chem. Rev., 2008, 108, 4935–4978 CrossRef CAS PubMed.
- S.-H. Yu and H. Colfen, J. Mater. Chem., 2004, 14, 2124 RSC.
- K. Naka and Y. Chujo, Chem. Mater., 2001, 13, 3245–3259 CrossRef CAS.
- L. A. Estroff and A. D. Hamilton, Chem. Mater., 2001, 13, 3227–3235 CrossRef CAS.
- I. Arias, C. Escobar, M. Bodero, M. David and S. Ferna, J. Mater. Chem., 2004, 2154–2160 RSC.
- A. Sheikhi, N. Li, T. G. M. van de Ven and A. Kakkar, Environ. Sci.: Water Res. Technol., 2016, 2, 71–84 RSC.
- J. H. Collier and P. B. Messersmith, Annu. Rev. Mater. Res., 2001, 31, 237–263 CrossRef CAS.
- C. Damle, A. Kumar, S. R. Sainkar, M. Bhagawat and M. Sastry, Langmuir, 2002, 18, 6075–6080 CrossRef CAS.
- D. N. Cacace, A. T. Rowland, J. J. Stapleton, D. C. Dewey and C. D. Keating, Langmuir, 2015, 31, 11329–11338 CrossRef CAS PubMed.
- E. Asenath-Smith, H. Li, E. C. Keene, Z. W. Seh and L. A. Estroff, Adv. Funct. Mater., 2012, 22, 2891–2914 CrossRef CAS.
- N. Rauner, M. Meuris, S. Dech, J. Godde and J. C. Tiller, Acta Biomater., 2014, 10, 3942–3951 CrossRef CAS PubMed.
-
D. R. Lide, CRC Handbook of Chemistry and Physics, CRC Press Inc., 73rd edn, 1992 Search PubMed.
- A. T. Kan, J. E. Oddo and M. B. Tomson, Langmuir, 1994, 10, 1450–1455 CrossRef CAS.
-
O. Sohnel and J. Garside, Precipitation, Butterworth-Heinemann, 1992 Search PubMed.
- T. Wang, G. Rother and H. Cölfen, Macromol. Chem. Phys., 2005, 206, 1619–1629 CrossRef CAS.
- S. F. Chen, S. H. Yu, T. X. Wang, J. Jiang, H. Cölfen, B. Hu and B. Yu, Adv. Mater., 2005, 17, 1461–1465 CrossRef CAS.
- S. H. Yu, H. Cölfen, J. Hartmann and M. Antonietti, Adv. Funct. Mater., 2002, 12, 541–545 CrossRef CAS.
- J. Rudloff, M. Antonietti, H. Cölfen, J. Pretula, K. Kaluzynski and S. Penczek, Macromol. Chem. Phys., 2002, 203, 627–635 CrossRef CAS.
- J. Rudloff and H. Cölfen, Langmuir, 2004, 20, 991–996 CrossRef CAS PubMed.
- S. Bentov, S. Weil, L. Glazer, A. Sagi and A. Berman, J. Struct. Biol., 2010, 171, 207–215 CrossRef CAS PubMed.
-
Dendrimers, Dendrons and Dendritic Polymers, ed. D. A. Tomalia, J. B. Christensen and U. Boas, Cambridge Univ. Press, 2012 Search PubMed.
- G. Franc and A. K. Kakkar, Chem. Soc. Rev., 2010, 39, 1536–1544 RSC.
- K. P. Fears, S. E. Creager and R. A. Latour, Langmuir, 2008, 24, 837–843 CrossRef CAS PubMed.
- A. Sheikhi, A. Kakkar and T. G. M. van de Ven, Cryst. Growth Des., 2016, 16, 4627–4634 CrossRef CAS.
- C. Malardier-Jugroot, T. G. M. van de Ven and M. A. Whitehead, Mol. Simul., 2005, 31, 173–178 CrossRef CAS.
Footnotes |
† Electronic supplementary information (ESI) available: Details of dendrimer synthesis and long-time biomimetic mineralization of calcium carbonate. See DOI: 10.1039/c8qm00301g |
‡ Current address: California NanoSystems Institute (CNSI), Center for Minimally Invasive Therapeutics (C-MIT), Department of Bioengineering, University of California-Los Angeles, 570 Westwood Plaza, CNSI 4523, Los Angeles, CA 90095, USA. E-mail: sheikhi@ucla.edu |
|
This journal is © the Partner Organisations 2018 |