Coexisting properties of thermostability and ultraviolet radiation resistance in the main S-layer complex of Deinococcus radiodurans†
Received
28th June 2017
, Accepted 24th November 2017
First published on 27th November 2017
Abstract
Deinococcus radiodurans is well known for its unusual resistance to different environmental stresses. Recently, we have described a novel complex composed of the surface (S)-layer protein DR_2577 and the carotenoid deinoxanthin. We also showed a role of this complex in the UV resistance under desiccation. Both these properties, UV and desiccation resistance, suggest a selective pressure generated by Sun irradiation. In order to confirm this hypothesis we checked whether this S-layer Deinoxanthin Binding Complex (SDBC) has features of thermo-resistance, a property also expected in proteins evolved under solar irradiative pressure. We performed the spectroscopic characterization of the SDBC by means of thermal shift assay, circular dichroism and related in silico analysis. Our findings identify a stability typical of thermo-adapted proteins and provide a new insight into the origin of specific S-layer types. The results are discussed in terms of co-evolutionary mechanisms related to Sun-induced desiccation and heat.
Introduction
Deinococcus radiodurans, as well as other eubacteria and archaea, shows an ordered paracrystalline layer of proteins called the Surface (S)-layer that surrounds the external surface of the outer membrane.1 Recently, we have shown that D. radiodurans retains the carotenoid deinoxanthin on its surface by building a complex with the main S-layer protein DR_2577,2 also known as SlpA. This S-layer Deinoxanthin Binding Complex (SDBC) is a homo-oligomer, most likely a homo-hexamer of about 770 kDa.3 Due to both the cofactor deinoxanthin and the particular amino acid composition of DR_2577, this complex exhibits spectral properties, which comply with the UV-radiation resistance and the related resistance to deep desiccation observed in D. radiodurans.2 In this bacterium, radio-resistance is assumed to be the indirect result of adaptation processes associated with dehydration, for which the related DNA damage was handled through the specialization of an efficient system of DNA repair.4 In fact, the retention of UV-resistance properties is not obvious since nowadays the terrestrial rate of UV radiation is too low to be considered a selective force.4 However, environments characterized by having high background radiation are shown to select radiation-resistant bacteria,5 and, related to this, experiments on microbes exposed to UV-radiation showed their ability to evolve resistance up to 3 times higher than their ancestors.6 These observations suggest that bacteria are capable of adaptation to environmental changes including the ones related to electromagnetic stress such as dose-related changes or exposure to γ-, UV- and IR-radiation. In this respect, rather than being of indirect origin, the SDBC properties may be considered as the vestigial and direct result of a selection that took place four billion years ago when the early Earth, lacking the ozone layer, was exposed to high-energy solar radiation. These environmental conditions might have been an evolutionary force strong enough to shape at the same time several mechanisms of resistance against heat and photons. This hypothesis may explain the thermo-tolerance exhibited by several mesophilic species including D. radiodurans.7–9
Sun irradiation is characterized by a high-energy UV component, which could cause photo-oxidation and ROS formation, and a lower energy IR component, which could cause heating and ultimately drying. These components, responsible for electromagnetic, thermal and thereby desiccation damage, are strictly connected and coexisting. To cope with such harmful factors, especially in the case of radioactive environments,10 bacteria evolved different and simultaneous mechanisms of radiation resistance including efficient DNA and protein repair systems,11 and protection mechanisms based on the antioxidant properties of carotenoids.2,12–17Accordingly, the combined UV and desiccation resistance associated with the SDBC might be the result of the evolution to survive in extreme environments that nowadays are confined to peculiar terrestrial niches, such as higher layers of the atmosphere and deserts located at high altitudes in which, interestingly, Deinococcus species were reported to be found.18–20 In this respect, considering a selective pressure driven by the Sun, a specific pattern of heat-induced properties related to thermo-resistance, as found in phylogenetically related thermophilic bacteria such as Thermus thermophilus, may also be expected for D. radiodurans and, in particular, for its SDBC. In order to explore this hypothesis and provide a landscape of the possible selective conditions that led to the extreme SDBC properties, in this work we performed the spectroscopic characterization of the SDBC by means of thermal shift assay, circular dichroism and related in silico and in vivo analyses. The obtained results show that the SDBC carries typical features of thermo-adapted proteins confirming not only that the SDBC derives from an ultraviolet-induced selective pressure, but also that, during evolution, this complex was subjected to selective pressures due to heat stress. Along the same lines, the results also provide an explanation for the persistency of ultraviolet radiation resistance even though nowadays the extent of its damaging effect is only limited to particular environments. In this respect, we discuss our findings in terms of co-evolutionary mechanisms to cope with Sun-induced desiccation and heat, which may explain the persistency of ultraviolet stress resistance. Moreover, the observed features provide an unedited suggestion on the origin of specific S-layer types, confirming their role as protective structures that enhance the cell stability in extreme environments.
Experimental
Bacterial strains, growth conditions and SDBC isolation
Cell cultures of D. radiodurans strain R1 (ATCC 13939) were grown in Tryptone/Glucose/Yeast extract media (TGY)21 for 24 hours at 25 °C till the stationary phase (OD600 of 1.7–1.8), under shaking at 250 rpm. The D. radiodurans cell walls were isolated according to ref. 22, while the subsequent SDBC isolation was performed according to ref. 2. Assays of thermo tolerance were performed on TGY plates solidified with 1.5% of agar at 25 and 35 °C for both the wild type and the DR_2577 deletion (ΔDR_2577) strains.2
Absorption spectroscopy and Sun emission spectra
The absorption spectroscopy measurements were performed with a Pharmacia Biotech Ultrospec 4000 spectrophotometer (optical path length of 1 cm; band-pass of 2 nm) using an Ultra Micro quartz cell with a 10 mm light path (Hellma Analytics). DR_2577 spectra were recorded at a protein concentration of 0.05 mg ml−1 at 4 °C in the spectral range of 200–750 nm. The AM 1.5 G and AM 0 G spectra used were the ASTM G173–03 reference spectra derived from the program SMARTS (Simple Model for the Atmospheric Radiative Transfer of Sunshine) v 2.9.2. (http://rredc.nrel.gov/solar/spectra/am1.5/astmg173/astmg173.html).
Fluorescence spectroscopy
Fluorescence spectroscopy experiments were performed on a Jasco FP-8200 spectrofluorometer at 4 °C, using an Ultra Micro quartz cell with a 3 mm light path (Hellma Analytics). The concentration of the DR_2577 samples was kept as reported in the Absorption spectroscopy section. The main absorption bands were used as excitation wavelengths to record the emission spectra (200–700 nm range). The excitation spectra (200–700 nm range) were recorded on the main emission band (325 nm).
Thermal shift assay
The calculated melting temperature (Tmc), based on the primary sequence, was determined in silico by using the software online platform Tm predictor (http://tm.life.nthu.edu.tw/). The thermal shift assay by means of absorption spectroscopy was performed on 300 μL of purified SDBC at intervals of 5 °C in the range between 25 and 95 °C. Measurements were performed after 5 minutes of thermal exposure. The absorption measurements were performed on an Ultrospec 3100 Pro spectrophotometer (Amersham) using a quartz cuvette with a 10 mm light path (Hellma Analytics) under a band pass of 1 nm. Considering that the tyrosine frequencies of the SDBC are above the standard average for proteins,2 the absorbance as a function of temperature was measured at a wavelength of 276 nm, a typical absorption band for tyrosine residues. Data analysis was performed as described in ref. 23. Briefly, the measured data were fitted using a logistic function and the inflection point, equivalent to the experimental melting temperature (Tme), was identified graphically as the midpoint between the asymptote at low absorbances, equivalent to 100% of folded species (and 0% of unfolded ones) in the sample, and the one at high absorbances, equivalent to 0% of folded species (and 100% of unfolded ones).
Circular dichroism spectroscopy
DR_2577 pure samples were analysed by means of circular dichroism (CD). The spectra were the average of three accumulations recorded at a sensitivity of 100 mdeg and a scan speed of 100 nm min−1 using a CD spectrometer Jasco J-810. Absorption and CD spectra were recorded at 4 °C temperature in the range of 190–250 nm, with a band-pass of 2 nm. The spectra were recorded on an absorption Ultra Micro quartz cell with a 10 mm light path (Hellma Analytics). In all cases, measurements were performed using a protein concentration of 4 μM. The data processing was performed with the program Bestsel (http://bestsel.elte.hu/index.php)24 using the measured ellipticity (expressed in mdeg) by providing the specific concentration (1 μM), number of residues (1167) and path length (1 cm).
Bioinformatic analysis
The sequences of DR_2577 and SLAP1_THET8 were obtained from the Universal Protein Knowledgebase (UniProt) server (http://www.uniprot.org/
25). The amino acid frequencies were analysed using the ExPasy – ProtParam server (http://web.expasy.org/protparam/
26).
Results and discussion
A high thermostability allows the SDBC to cope with the heat stress
In D. radiodurans, the properties of resistance to desiccation and UV radiation may be the result of adaptation to solar irradiation. If this assumption is correct, the SDBC should show the properties of thermoresistance. To test this hypothesis, we performed an in silico analysis based on the primary structure of DR_2577, which predicted a melting temperature (Tmc) value of 55 °C for the monomer. Similar analysis on the dimeric DR_2577, which is known to be exceptionally stable,3 led to a Tmc of 60 °C. Further analysis on the hexamers of DR_2577, constituting the SDBC,2 led to a further increase of Tmc to a value of 74 °C (Table 1). These experiments prompted us to carry out further in vitro tests on pure SDBC samples. A thermal shift assay by means of absorption spectroscopy was performed in order to assess the folding stability of the protein on the basis of its experimental melting temperature (Tme) in the range between 25 and 95 °C after 5 minutes of thermal exposure. Measurements were performed at a wavelength of 276 nm, the typical absorption peak of tyrosine, an amino acid present at a high level in the SDBC. The obtained dataset was approximated by a logistic function with a resulting Tme value of 81 °C (Table 1), which confirms the high stability of the SDBC hexamer (Fig. 1). In order to provide a reference of thermostability, the SDBC Tm values were compared with the Tmc value (67 °C) of the SLAP1_THET8 protein (monomer) from Thermus thermophilus, which is known to have 25% identity and 37% similarity at the amino acid level with DR_257727 and to be adapted to resist high temperatures (Table 1). These experiments suggested a comparable propensity of the SDBC to resist high doses of heat as in thermophiles, a useful property in order to cope with prolonged direct Sun exposure.
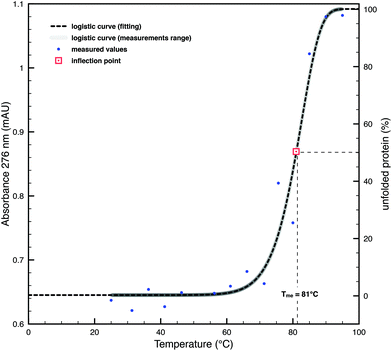 |
| Fig. 1 Thermal shift assay on the SDBC samples. Blue dots represent the measured absorbance at 276 nm at different temperatures. The fitted logistic curve (dashed-dark curve) and its interval in the range of measurements (solid-grey curve) show a typical melting curve. The inflection point, that graphically is the midpoint between the asymptotic side at lower absorbances and the one at higher absorbances, is equivalent to Tme (red dot) and has a temperature of 81 °C. | |
Table 1 Calculated (Tmc) and experimental (Tme) melting temperatures for different oligomeric states of the main S-layer protein of D. radiodurans (DR_2577) and for the monomer of T. thermophilus (SLPA1_THET8)
Protein |
Oligomeric state |
T
m (°C) |
T
mc
|
T
me
|
n.a. = not applicable.
|
D. radiodurans (protein DR_2577) |
Monomer |
55 |
n.a.a |
Dimer |
60 |
n.a.a |
Hexamer |
74 |
81 |
T. thermophilus (protein SLAP1_THET8) |
Monomer |
67 |
n.a.a |
The secondary structure inferred by in silico analysis and circular dichroism spectroscopy confirms the high SDBC stability
Further experiments were carried out to find the structural features that provide such thermostability. In particular, in silico analysis based on the primary structure (Fig. 2) predicted the prevalence of disordered regions, coils and turns (68.72%) followed by beta-barrels (23.3%) and a limited amount of alpha helices (7.96%). To confirm these findings, we performed CD experiments on pure SDBC samples (Fig. 2). The analysis was followed by a secondary structure prediction based on the CD data in the 190–250 nm spectral range. For this analysis the software Bestsel was used, confirming the dominance of disordered regions, coils and turns (62.7%), followed by beta-barrels (34.9%) and a very low content of alpha helices, (2.4%) in agreement with the in silico findings (Fig. 2).
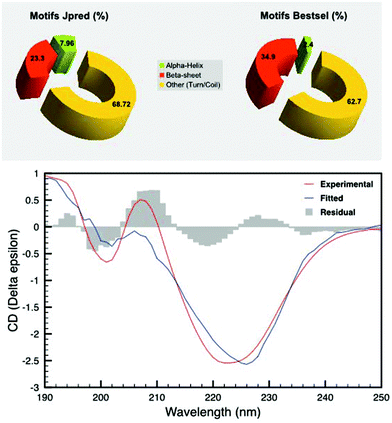 |
| Fig. 2 Secondary structure prediction by CD spectroscopy and in silico studies. Secondary structure prediction using the software Jpred shows a dominance of disordered regions mixed with random coils and turns (68.72%), beta-barrels (23.3%) and alpha helices (7.96%) (top left); on the bottom is shown the CD spectrum (red line) and the fitted spectrum calculated using the software Bestsel (blue line). The residual between the curves is shown as a histogram in grey. The secondary structure prediction according to Bestsel (top right) confirms a dominance of disordered regions mixed with random turns and coils (62.7%), beta-barrels (34.9%) and alpha helices (2.4%) confirming in silico findings. | |
The SDBC shows a pattern of amino acid frequencies typical of thermostable proteins
In order to confirm the results from the Tm and CD analyses, we verified whether in DR_2577 the property of thermostability was also reflected by amino acid frequencies and compositions. We analysed the amino acid frequencies of DR_2577 and compared them with those of the protein SLAP1_THET8 from T. thermophilus, and the averaged frequencies for mesostable and thermostable proteins28 (Fig. 3). The comparison shows that residues such as aspartate and arginine are present in DR_2577 at frequencies (8.0% and 6.1%, respectively) comparable to the ones reported for thermostable (6.37% and 5.02%) and mesostable proteins (6.4% and 3.97%, respectively), confirming the thermostable feature of this protein. Similarly, the frequencies of alanine and glycine, which are expected to increase in order to stabilize the aspartate and arginine residues,28 are higher in DR_2577 (11.7% and 12.0%, respectively) than the ones for mesostable (8.44% and 8.21%, respectively) and thermostable proteins (7.93% and 7.99%, respectively). Comparable high values are observed for the protein SLAP1_THET8 from the thermophile T. thermophilus in which frequencies of 13.9% for alanine and 9.7% for glycine are found. Moreover, the frequencies of cysteine, tryptophan and histidine decreased in DR_2577 (0.2%, 0.2%, and 0.4%, respectively) compared to the ones in thermostable proteins (1.62%, 1.69%, and 2.13%, respectively28). This is consistent with our previous studies in which cysteine, tryptophan and histidine, that are known to be UV sensitive, were found only in little amount, contributing to the characteristic UV resistance of DR_2577.2
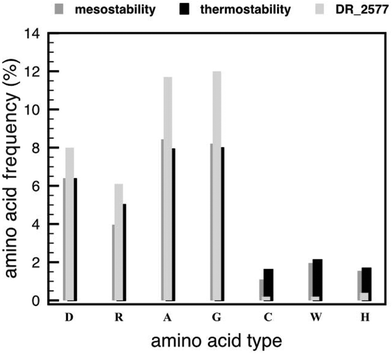 |
| Fig. 3 Comparison of DR_2577 with mesostable and thermostable proteins by following the frequencies of the most significant amino acids involved in providing thermostability. The histogram shows the frequencies of aspartate (D), arginine (R), alanine (A), glycine (G), cysteine (C), trypsin (W) and histidine (H) as the percentage of the total amino acid amount. The values for thermostable proteins (dark grey), mesostable proteins (light grey) and DR_2577 (dotted-square symbol) are compared. | |
The SDBC confers the property of thermotolerance in vivo
These results were further confirmed by assaying the thermo-protective role of DR_2577 and its SDBC in vivo. In these experiments, the growth of D. radiodurans at 35 °C and its DR_2577 deletion mutant strain (ΔDR_2577) was compared with their growth at the optimal temperature of 25 °C. These experiments showed a pronounced temperature-dependent sensitivity of the ΔDR_2577 with respect to the wild type, suggesting an involvement of the SDBC in providing the thermotolerance property to this bacterium (Fig. 4).
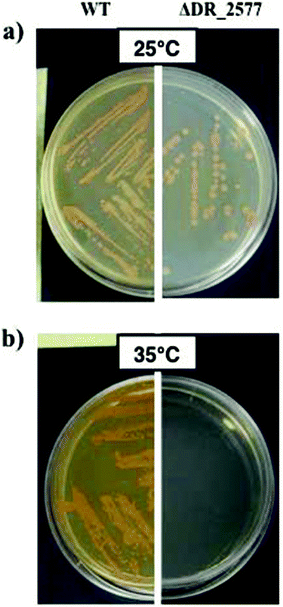 |
| Fig. 4 Comparative growth between D. radiodurans and its DR_2577 deletion mutant (ΔDR_2577) at 25 °C (a) and 35 °C (b). Temperatures higher (35 °C) than the optimal temperature for this bacterium (25 °C) allow the observation of a drastic effect on the ΔDR_2577 growth due to the SDBC depletion. | |
Overall interpretation
The S-layer is a proteinaceous coat constituting one of the most external layers of the bacterial cell wall. Although S-layers are considered structures with a function of protection, this property has rarely been proved by direct evidence. The SDBC was recently identified as the main component of the D. radiodurans S-layer.2 This complex is composed of the protein DR_2577 and its cofactor deinoxanthin,2 the main carotenoid of D. radiodurans.29 Deinoxanthin and DR_2577 are both reported to be essential in contributing to the UV and desiccation resistance features of this bacterium.2,27,30–32 Because of these features, the Sun radiation appears to be the main driving force in the evolution of the SDBC and its properties of resistance. Since electromagnetic, thermal and the ensuing desiccation damage is strictly connected, the coexistence of the properties of protection against UV radiation and desiccation together with the features of thermo-adaptation may be expected. We have analysed this possibility by finding a number of independent pieces of evidence indicating that the SDBC also possesses the property of thermo-adaptation.
Since the UV fraction of the Sun spectra is efficiently filtered by the atmosphere, the S-layer of D. radiodurans could be a vestigial structure originated in times when the sunlight was not subjected to the atmospheric filter. This observation is in agreement with the spectroscopic complementarity between the SDBC and the exoatmospheric Sun radiation (ESI Fig. 1 and 2†).
In particular, the spectral features of the SDBC2 extend beyond the emission range of the solar radiation on the Earth's surface (ESI Fig. 1 and 2†), while the exospheric solar spectrum, recorded just on the top of the atmosphere, includes also the wavelength range in which the SDBC is photoactive (ESI Fig. 1 and 2†). This suggests its origin back to the UV-radiation stress characteristic of the Archean era (3.9–2.5 Ga ago). During this time, the Earth, lacking the ozone layer, was exposed to a high flux of UVC (200–280 nm) and UVB (280–315 nm) radiation, as suggested by the conditions present on Mars’ surface nowadays.33 In the Archean era, the characteristic adaptive radiation of several groups of bacteria, including the Deinococcus–Thermus phylum is also well documented.33–35 Early life evolved in water36 and among other important properties, oceans behaved as a UV filter, protecting organisms from radiation, heat and desiccation.37 However, the adaptation to unstable terrestrial environments, such as the tidemark regions, may have played a significant role in selecting potential ancestors resistant to UV radiation, heat and desiccation. The representatives of the Deinococcus–Thermus phylum can be easily placed into this context. If the assumption that the SDBC and its S-layer are vestigial structures is correct, then an important question is why this structure is retained even now. Vestigial features are frequently observed in biology and their level of preservation is directly related to their co-evolution with other features that are important for the fitness of the organism in a given environment.38,39 Extreme environments are frequently characterized by a combination of coexisting extreme factors, which explains the poly-extremophilic profile of D. radiodurans and other extremophiles. A specialized S-layer fits well in this context. Being the main component of this S-layer and the forefront of the bacterial cell encountering radiation, the SDBC appears to be designed to resist not only UV light, but also other side effects of high Sun exposure, such as heat and desiccation. Therefore, the S-layer integrity was preserved to cope with such harmful conditions still present now despite the reduced level of UV radiation.
D. radiodurans, among other properties, has also the ability to survive for long periods under deep desiccation4,11,40–46 and the S-layer integrity was found to be essential for protecting the bacterium against desiccation under UV radiation.2 Here, we find that the S-layer protein DR_2577 is not only able to provide protection under desiccation in the presence of UV, but also its apoprotein is resistant and structurally adapted to heat stress. In fact, the secondary structure of DR_2577, its amino acid sequence and relative frequencies (Fig. 3) reveal a strong thermostability that is comparable to its counterpart from the thermophilic bacterium T. thermophilus27 (Table 1). The property of thermostability associated with DR_2577 is also confirmed by the characteristic thermo-sensitivity observed for the DR_2577 deletion mutant at temperatures above the optimal range (Fig. 4). This is an indication that in thermo-adapted proteins, the secondary and tertiary structures are related to stability, as already reported for the adaptation of thermophilic bacteria and archaea.47 The structural organization of DR_2577 is dominated by disordered regions and junction motifs including coils and turns (>60%, Fig. 2). Heating increases beta-strand structures at the expense of the alpha-helix with only minor changes in the disordered regions.48 Accordingly, as identified by the CD measurements (Fig. 2), the DR_2577 has only a small amount of alpha-helix, and thus its structural organization does not suffer from big changes at higher temperatures (as confirmed by its Tm values, Table 1), offering resistance towards heat stress. Moreover, the high amount of random motifs provides the flexibility for a reversible deformation of the cell, which is essential for dealing not only with heat stress, but also with physical stress in general. Our findings show that the hexameric oligomerization of the SDBC increases the thermostability (Tmc 55 °C of monomers vs. Tmc 74 °C for hexamers). These results, as summarized in Table 1, are in agreement with previous studies reporting that in protein complexes from thermo-resistant organisms oligomerization can play a major role in extreme thermostabilization.49–51 Finally, as opposed to hydrothermal extremophiles such as T. thermophilus where all the cellular components are adapted to high temperatures, in mesophilic bacteria the features of thermo-adaptation are associated with specific bacterial components and their related functions. This is in agreement with our in vivo assays (Fig. 4) on D. radiodurans where the high specialization of the SDBC may be seen as indirect evidence of adaptation to the effects of sun irradiation in mesothermic environments. These observations would agree with the properties of thermotolerance already described for this bacterium7–9 and other extremophiles such as the haloarchaea Haloferax volcanii which shows similar coexisting properties of UV and desiccation resistance.52–54
Conclusions
Overall, the described properties of DR_2577 and SDBC indicate a primordial role in protection against dangerous radiation and thermo-induced stress. Complex and ancient structures such as carotenoid-binding S-layers might have been among the earliest templates where the carotenoid selection has taken place. Therefore, these structures represent the evolutionary precursors of processes that require not only the presence of functionalized carotenoid-protein complexes, but also the presence of a surface with a regular and paracrystalline array of functional units. In this respect, carotenoid-binding S-layers might be instrumental in understanding the evolutionary origin of characteristic carotenoid functions which reached their highest expression in processes such as vision and photosynthesis.
Conflicts of interest
There are no conflicts to declare.
Acknowledgements
DP is grateful to the European Synchrotron Research Facility and the Partnership for Structural Biology (Grenoble, France) for preliminary studies. The ΔDR_2577 strain was kindly provided by Professor Mary E. Lidstrom (University of Washington, Seattle, USA). DF gratefully acknowledges the support from the L'Oréal-UNESCO Fellowship for Women in Science 2017, Italy (L'Oréal Italia Per le Donne e la Scienza). The authors acknowledge the reviewers for the helpful comments and suggestions.
References
- W. Baumeister, F. Karrenberg, R. Rachel, A. Engel, B. Heggeler and W. O. Saxton, The major cell envelope protein of Micrococcus radiodurans (R1). Structural and chemical characterization, Eur. J. Biochem., 1982, 125, 535–544 CrossRef CAS PubMed.
- D. Farci, C. Slavov, E. Tramontano and D. Piano, The S-layer protein DR_2577 binds the carotenoid deinoxanthin and under desiccation conditions protect against UV-radiation in Deinococcus radiodurans, Front. Microbiol., 2016, 7, 155 Search PubMed.
- D. Farci, M. W. Bowler, F. Esposito, S. McSweeney, E. Tramontano and D. Piano, Purification and characterization of DR_2577 (SlpA) a major S-layer protein from Deinococcus radiodurans, Front. Microbiol., 2015, 6, 414 Search PubMed.
- V. Mattimore and J. R. Battista, Radioresistance of Deinococcus radiodurans: functions necessary to survive ionizing radiation are also necessary to survive prolonged desiccation, J. Bacteriol., 1996, 178, 633–637 CrossRef CAS PubMed.
- M. X. Ruiz-González, G. Á. Czirják, P. Genevaux, A. P. Møller, T. A. Mousseau and P. Heeb, Resistance of Feather-Associated Bacteria to Intermediate Levels of Ionizing Radiation near Chernobyl, Sci. Rep., 2016, 6, 22969 CrossRef PubMed.
- M. Wassmann, R. Moeller, G. Reitz and P. Rettberg, Adaptation of Bacillus subtilis cells to Archean-like UV climate: relevant hints of microbial evolution to remarkably increased radiation resistance, Astrobiology, 2010, 10, 605–615 CrossRef CAS PubMed.
- K. Harada, M. Moriwaki and S. Oda, Arrhenius plot analysis of the mechanism of the thermotolerance induction in the radioresistant bacterium Deinococcus radiodurans, J. Gen. Appl. Microbiol., 1988, 34, 209–212 CrossRef.
- Y. Liu, J. Zhou, M. V. Omelchenko, A. S. Beliaev, A. Venkateswaran, J. Stair, L. Wu, D. K. Thompson, D. Xu, I. B. Rogozin, E. K. Gaidamakova, M. Zhai, K. S. Makarova, E. V. Koonin and M. J. Daly, Transcriptome dynamics of Deinococcus radiodurans recovering from ionizing radiation, Proc. Natl. Acad. Sci. U. S. A., 2003, 100, 4191–4196 CrossRef CAS PubMed.
- A. Airo, S. L. Chan, Z. Martinez, M. O. Platt and J. D. Trent, Heat shock and cold shock in Deinococcus radiodurans, Cell Biochem. Biophys., 2004, 40, 277–288 CrossRef CAS PubMed.
- D. Asker, T. Beppu and K. Ueda, Unique diversity of carotenoid-producing bacteria isolated from Misasa, a radioactive site in Japan, Appl. Microbiol. Biotechnol., 2007, 77, 383–392 CrossRef CAS PubMed.
- J. R. Battista, Against all odds: the survival strategies of Deinococcus radiodurans, Annu. Rev. Microbiol., 1997, 51, 203 CrossRef CAS PubMed.
- M. M. Mathews and N. I. Krisky, The relationship between carotenoid pigments and resistance to radiation in non-photosynthetic bacteria, Photochem. Photobiol., 1965, 4, 813–817 CrossRef CAS PubMed.
- W. Stahl and H. Sies, Carotenoids and protection against solar UV radiation, Skin Pharmacol. Appl. Skin Physiol., 2002, 15, 291–296 CrossRef CAS PubMed.
- H. Sies and W. Stahl, Carotenoids and UV protection, Photochem. Photobiol. Sci., 2004, 3, 749–752 CAS.
- W. Stahl and H. Sies, β-Carotene and other carotenoids in protection from sunlight, Am. J. Clin. Nutr., 2012, 96, 1179S–1184S CrossRef CAS PubMed.
- B. Tian and Y. Hua, Carotenoid biosynthesis in extremophilic Deinococcus-Thermus bacteria, Trends Microbiol., 2010, 18, 512–520 CrossRef CAS PubMed.
- A. Krisko and M. Radman, Biology of extreme radiation resistance: they way of Deinococcus radiodurans, Cold Spring Harbor Perspect. Biol., 2013, 5, a012765 Search PubMed.
- F. Peng, L. Zhang, X. Luo, J. Dai, H. An, Y. Tang and C. Fang,
Deinococcus xinjiangensis sp. nov., isolated from desert soil, Int. J. Syst. Evol. Microbiol., 2009, 59, 709–713 CrossRef CAS PubMed.
- Y. Yang, T. Itoh, S. Yokobori, S. Itahashi, H. Shimada, K. Satoh, H. Ohba, I. Narumi and A. Yamagishi,
Deinococcus aerius sp. nov., isolated from the high atmosphere, Int. J. Syst. Evol. Microbiol., 2009, 59, 1862–1866 CrossRef CAS PubMed.
- H. Bouraoui, M. B. Aissa, F. Abbassi, J. P. Touzel, M. O'donohue and M. Manai, Characterization of Deinococcus sahariens sp. nov., a radiation-resistant bacterium isolated from a Saharan hot spring, Arch. Microbiol., 2012, 194, 315–322 CrossRef CAS PubMed.
-
R. G. E. Murray, The family Deinococcaceae. The Prokaryotes, Springer, New York, 1992, pp. 3732–3744 Search PubMed.
- D. Farci, M. W. Bowler, J. Kirkpatrick, S. McSweeney, E. Tramontano and D. Piano, New features of the cell wall of the radio-resistant bacterium Deinococcus radiodurans, Biochim. Biophys. Acta, 2014, 1838, 1978–1984 CrossRef CAS PubMed.
- N. Poklar and G. Vesnaver, Thermal denaturation of proteins studied by UV spectroscopy, J. Chem. Educ., 2000, 77, 380 CrossRef.
- A. Micsonai, F. Wien, L. Kernya, Y. H. Lee, Y. Goto, M. Réfrégiers and J. Kardos, Accurate secondary structure prediction and fold recognition for circular dichroism spectroscopy, Proc. Natl. Acad. Sci. U. S. A., 2015, 112, E3095-103 CrossRef PubMed.
- R. Apweiler, A. Bairoch and C. H. Wu, Protein sequence databases, Curr. Opin. Chem. Biol., 2004, 8, 76–80 CrossRef CAS PubMed.
-
E. Gasteiger, C. Hoogland, A. Gattiker, S. Duvaud, M. R. Wilkins, R. D. Appel and A. Bairoch, Protein Identification and Analysis Tools on the ExPASy Server, in The Proteomics Protocols Handbook, ed. J. M. Walker, Humana Press, 2005, pp. 571–607 Search PubMed.
- H. Rothfuss, J. C. Lara, A. K. Schmid and M. E. Lidstrom, Involvement of the S-layer proteins HPI and SlpA in the maintenance of cell envelope integrity in Deinococcus radiodurans, R1, Microbiology, 2006, 152, 2779–2787 CrossRef CAS PubMed.
- B. Folch, Y. Dehouck and M. Rooman, Thermo- and mesostabilizing protein interactions identified by temperature-dependent statistical potentials, Biophys. J., 2010, 98, 667–677 CrossRef CAS PubMed.
- L. Lemee, E. Peuchant, M. Clerc, M. Brunner and H. Pfander, Deinoxanthin: A new carotenoid isolated from Deinococcus radiodurans, Tetrahedron, 1997, 53, 919–926 CrossRef CAS.
- N. E. Gentner and R. E. Mitchel, Ionizing radiation-induced release of a cell surface nuclease from Micrococcus radiodurans, Radiat. Res., 1975, 61, 204–215 CrossRef CAS PubMed.
- S. Karlin and J. Mrazek, Predicted highly expressed and putative alien genes of Deinococcus radiodurans and implications for resistance to ionizing radiation damage, Proc. Natl. Acad. Sci. U. S. A., 2001, 98, 5240–5245 CrossRef CAS PubMed.
- L. Zhang, Q. Yang, X. Luo, C. Fang, Q. Zhang and Y. Tang, Knockout of crtB or crtI gene blocks the carotenoid biosynthetic pathway in Deinococcus radiodurans R1 and influences its resistance to oxidative DNA-damaging agents due to change of free radicals scavenging ability, Arch. Microbiol., 2007, 188, 411–419 CrossRef CAS PubMed.
-
C. S. Cockell, The ultra-violate radiation environment of Earth and Mars: past and present, in Astrobiology: The Quest for the Conditions of Life, ed. G. Horneck and C. Baumstark-Khan, Springer-Verlag, Berlin, 2002, pp. 219–232 Search PubMed.
- C. E. Blank, Evolutionary timing of the origins of mesophilis sulphate reduction and oxygenic photosynthesis: a phylogenomic dating approach, Geobiology, 2004, 2, 1–20 CrossRef CAS.
- C. E. Blank, Not so old Archaea – the antiquity of biogeochemical processes in the archael domain of life, Geobiology, 2009, 7, 495–514 CrossRef CAS PubMed.
- E. G. Nisbet and N. H. Sleep, The habitat and nature of early life, Nature, 2001, 409, 1083–1091 CrossRef CAS PubMed.
- J. H. Cleaves and S. L. Miller, Oceanic protection of prebiotic organic compounds
from UV radiation, Proc. Natl. Acad. Sci. U. S. A., 1998, 95, 7260–7263 CrossRef.
- E. D. Brodie and B. J. Ridenhour, Reciprocal selection at the phenotypic interface of coevolution, Integr. Comp. Biol., 2003, 43, 408–418 CrossRef PubMed.
- T. Held, A. Nourmohammad and M. Lässig, Adaptive evolution of molecular phenotypes, J. Stat. Mech.: Theory Exp., 2014, P09029 CrossRef.
- A. Anderson, H. Nordon, R. Cain, G. Parrish and D. Duggan, Studies on a radio-resistant Micrococcus. I. (1956). Isolation, morphology, cultural characteristics, and resistance to gamma radiation, Food Technol., 1956, 10, 575 Search PubMed.
- D. E. Duggan, A. W. Anderson, P. R. Elliker and R. F. Cain, Ultraviolet exposure studies on a gamma radiation-resistant micrococcus isolated from food, Food Res., 1959, 24, 376–382 CrossRef.
- J. R. Battista, A. M. Earl and M. J. Park, Why is Deinococcus radiodurans so resistant to ionizing radiation?, Trends Microbiol., 1999, 7, 362–365 CrossRef CAS PubMed.
- M. M. Cox and J. R. Battista,
Deinococcus radiodurans - the consummate survivor, Nat. Rev. Microbiol., 2005, 3, 882–892 CrossRef CAS PubMed.
- J. K. Fredrickson, S. M. Li, E. K. Gaidamakova, V. Y. Matrosova, M. Zhai, H. M. Sulloway, J. C. Scholten, M. G. Brown, D. L. Balkwill and M. J. Daly, Protein oxidation: key to bacterial desiccation resistance?, ISME J., 2008, 2, 393–403 CrossRef CAS PubMed.
- D. Slade and M. Radman, Oxidative stress resistance in Deinococcus radiodurans, Microbiol. Mol. Biol. Rev., 2011, 75, 133–191 CrossRef CAS PubMed.
- A. D. Das and H. Misra, Hypothetical proteins present during recovery phase of radiation resistant bacterium Deinococcus radiodurans are under purifying selection, J. Mol. Evol., 2013, 77, 31–42 CrossRef CAS PubMed.
- A. Szilágyi and P. Závodszky, Structural differences between mesophilic, moderately thermophilic and extremely thermophilic protein subunits: results of a comprehensive survey, Structure, 2000, 8, 493–504 CrossRef.
- S. Ngarize, H. Herman, A. Adams and N. Howell, Comparison of changes in the secondary structure of unheated, heated, and high-pressure-treated beta-lactoglobulin and ovalbumin using fourier transform raman spectroscopy and self-deconvolution, J. Agric. Food Chem., 2004, 52, 6470–6477 CrossRef CAS PubMed.
- U. Opitz, R. Rudolph, R. Jaenicke, L. Ericsson and H. Neurath, Proteolytic Dimers of Porcine Muscle Lactate Dehydrogenase: Characterization, Folding, and Reconstitution of the Truncated and Nicked Polypeptide Chain, Biochem, 1987, 26, 1399–1406 CrossRef CAS.
- M. Kohlhoff, A. Dahm and R. Hensel, Tetrameric triosephosphate isomerase from hyperthermophilic Archaea, FEBS Lett., 1996, 383, 245–250 CrossRef CAS PubMed.
- V. Villeret, B. Clantin, C. Tricot, C. Legrain, M. Roovers, V. Stalon, N. Glansdorff and J. van Beeumen, The crystal structure of Pyrococcus furiosus ornithine carbamoyltransferase reveals a key role for oligomerization in enzyme stability at extremely high temperatures, Proc. Natl. Acad. Sci. U. S. A., 1998, 95, 2801–2806 CrossRef CAS.
- X. C. Abrevaya, I. G. Paulino-Lima, D. Galante, F. Rodrigues, P. J. Mauas, E. Cortón and A. Lage Cde, Comparative survival analysis of Deinococcus radiodurans and the haloarchaea Natrialba magadii and Haloferax volcanii exposed to vacuum ultraviolet irradiation, Astrobiology, 2011, 11, 1034–1040 CrossRef CAS PubMed.
- K. Zerulla and J. Soppa, Polyploidy in haloarchaea: advantages for growth and survival, Front. Microbiol., 2014, 5, 274 Search PubMed.
- J. Soppa, Polyploidy in archaea and bacteria: about desiccation resistance, giant cell size, long-term survival, enforcement by eukaryotic host and additional aspects, J. Mol. Microbiol. Biotechnol., 2014, 24, 409–419 CrossRef CAS PubMed.
Footnote |
† Electronic supplementary information (ESI) available. See DOI: 10.1039/c7pp00240h |
|
This journal is © The Royal Society of Chemistry and Owner Societies 2018 |