Physiological responses and toxin production of Microcystis aeruginosa in short-term exposure to solar UV radiation
Received
14th July 2017
, Accepted 14th November 2017
First published on 15th November 2017
Abstract
The aim of this study was to evaluate the effects of short-term (hours) exposure to solar UV radiation (UVR, 280–400 nm) on the physiology of Microcystis aeruginosa. Three solar radiation treatments were implemented: (i) PAR (PAR, 400–700 nm), (ii) TUVA (PAR + UVAR, 315–700 nm) and (iii) TUVR (PAR + UVAR + UVBR, 280–700 nm). Differential responses of antioxidant enzymes and the reactive oxygen species (ROS) production to UVR were observed. Antioxidant enzymes were more active at high UVR doses. However, different responses were observed depending on the exposure to UVAR or UVBR and the dose level. No effects were observed on the biomass, ROS production or increased activity of superoxide dismutase (SOD) and catalase (CAT) compared to the control when UVR + PAR doses were lower than 9875 kJ m−2. For intermediate doses, UVR + PAR doses between 9875 and 10
275 kJ m−2, oxidative stress increased while resistance was imparted through SOD and CAT in the cells exposed to UVAR. Despite the increased antioxidant activity, biomass decrease and photosynthesis inhibition were observed, but no effects were observed with added exposure to UVBR. At the highest doses (UVR + PAR higher than 10
275 kJ m−2), the solar UVR caused decreased photosynthesis and biomass with only activation of CAT by UVBR and SOD and CAT by UVAR. In addition, for such doses, a significant decrease of microcystins (MCs, measured as MC-LR equivalents) was observed as a consequence of UVAR. This study facilitates our understanding of the SOD and CAT protection according to UVAR and UVBR doses and cellular damage and reinforces the importance of UVR as an environmental stressor. In addition, our results support the hypothesized antioxidant function of MCs.
Introduction
Cyanobacteria are a group of phototrophic organisms that have great ecological and economical importance. They existed on Earth for around 2500–3500 million years, when the weather conditions were extreme, mainly due to high levels of ultraviolet radiation (UVR, 280–400 nm).1 During the past few decades, springtime stratospheric ozone depletion over the Antarctic and the Southern Ocean has caused enhanced levels of ultraviolet B radiation (UVBR, 280–315 nm) to reach the Earth's surface.2 Although seasonal ozone depletion continues to occur over the Antarctic, the severity of the depletion is lessening and the expectation is that the seasonal depletion will cease by 2050.3
Some species of Microcystis can regulate their position in the water column, due to gas vesicles, while searching favorable depths for their development.4,5 In shallower depths, cyanobacteria may be exposed to increased solar UVR doses due to less light attenuation in the water column as a consequence of low turbidity.6 UVR can induce significant damage to a variety of cell targets, including DNA,7 proteins1 and photosystem II (PS II).6 UVAR (315–400 nm), like UVBR, has the potential for cell damage, which is caused by both direct effects and indirect effects via the production of reactive oxygen species (ROS).8 UVAR mainly has indirect effects via energy transfer from UVAR stimulated chromophores to the DNA target or via the photosensitized production of ROS.9,10 In addition, UVAR induces direct damage to PS II via the same mechanism as UVBR does.11 Growth and biomass accumulation will result from the complex interactions between the direct and indirect harmful effects of UVR, and a series of counteracting repair mechanisms.12 UVBR is more effective per energy unit,13 however, UVAR is responsible for most of the UVR damage just because its natural levels are much higher.14,15 In addition, the generalization of UVR effects on cyanobacteria is complex, considering that the responses are species-specific.16
The responses of cyanobacteria to UVR effects could include the generation of ROS. In all aerobically living organisms, respiration is thought to be a source of ROS produced inside the cells. In addition to ROS produced by the respiratory machinery, photosynthetic organisms are challenged by ROS generated by the photosynthetic electron transport chain. Light is essential for photosynthesis, but, at the same time, it can also be a source of major stress. The fact that cyanobacteria constantly produce oxygen under illumination makes it crucial for them to prevent electron escape from normal electron transfer pathways to oxygen, in order to avoid oxidative stress as much as possible. The chemistry of oxygen species is well documented.17 ROS, including singlet oxygen (1O2), superoxide anion (O2−), hydrogen peroxide (H2O2) and hydroxyl radical (OH−), are powerful oxidizing agents. Singlet oxygen (1O2) is produced by energy input to oxygen; it is highly reactive, it has a short half-life in cells18 and it reacts with target molecules (proteins, pigments, and lipids) in the immediate surroundings. The three oxygen reduction intermediates (O2−, H2O2, and OH−) have different intrinsic features, and therefore possess different reactivities, toxicity levels and targets. Both O2− and OH− have one unpaired electron each that makes them highly reactive towards biomolecules. Qian et al.19 showed, using the oxidation of 2′,7′-dichlorofluorescein diacetate (DCFH-DA, a general index of oxidative stress), that higher ROS levels destroy the pigment synthesis and the membrane integrity, causing the death of Microcystis aeruginosa.
On the other hand, cyanobacteria are the oldest autotrophic inhabitants of the planet, and, at some point, they may have been exposed to high UVR levels.1,20 Therefore, they must have developed effective mechanisms to counteract the detrimental effects of these highly energetic wavelengths. While some of these defenses are enzymatic [catalase (CAT), superoxide dismutase (SOD) and peroxidases], others are non-enzymatic (glutathione, α-tocopherol, β-carotene).12,17 When the balance between oxidant levels and antioxidant production is lost, the organisms have to face oxidative stress that generates a variety of damage.21
Cyanobacteria blooms are recognized as major health risks considering that some cyanobacteria strains produce a wide range of toxins, including neurotoxins and hepatotoxins, such as microcystins (MCs). Cellular MC production has been indirectly linked to environmental factors influencing cyanobacterial growth rates,22 which can account for a 3–4 fold variation in total MC concentrations.23 In addition, the action mechanisms and the ecophysiological toxin role remain unclear.24,25 For such reasons, the understanding of the environmental factors associated with MC production is a priority to predict toxic events in nature.26 Little information is found in the literature regarding the physiological effects and MC production by exposure of M. aeruginosa to increased solar UVR doses. The recent experimental data indicate that variations in MC concentrations were modified under stress conditions.27,28
The objective of the present study was to determine the activation of different in vivo enzymatic antioxidants (CAT and SOD) as a function of solar UVR intensity and quality as well as the consequent ROS increment with higher UVR doses in short-term (hours) exposure. We hypothesize that on increasing UVR doses the enzymatic antioxidant protection will not be the same for UVA or UVB irradiance according with the differences in the prevalence of different ROS generated. In addition, we related this differential protection to toxin synthesis, evaluating their effects on the growth rate and photosynthesis.
Materials and methods
Experimental set-up
The experiments were performed using M. aeruginosa (strain CAAT 2005-3), a wild-type strain, isolated from a water body located in the Province of Buenos Aires, Argentina.29
Unialgal cultures were grown in liquid BG-1130 at 26 °C. For experiments, we used cells from the cultures in the exponential growth phase. In order to avoid cell damage as a consequence of changes in irradiance from inside the incubator to solar exposure, the M. aeruginosa cultures were pre-adapted to PAR irradiance in an outdoor water bath with running water for temperature control (26 °C ± 1) in containers covered with UV cut-off filters rather than expose the cells to only PAR irradiance (see treatment “3”), 1 day previous to the experimental day. After this period, the cells were exposed simultaneously to three irradiance treatments:
(1) cultures that received full radiation (UVBR, UVAR and PAR)—uncovered quartz tubes (TUVR treatment);
(2) cultures that received UVAR and PAR—tubes covered with UV cut-off filter foil (Montagefolie No. 10155099, Folex, Germany: 50% transmission at 320 nm) (TUVA treatment); and
(3) cultures that received only PAR—containers covered with an Ultraphan film (UV Opak, Digefra, Munich, Germany—50% transmission at 395 nm) (PAR treatment).
The spectra of the materials used in our experiments are published in Hernando and Ferreyra.31
In order to determine the UVBR effect it was calculated as the difference between TUVR and TUVA values for each parameter (Biomass, ROS, CAT and SOD).
The M. aeruginosa culture was exposed to natural sunlight at Buenos Aires (34°35′S; 58°22′W) during spring and summer in 2014/2015, in an outdoor water bath with running water for temperature control. In order to expose the cells to maximum solar radiation doses, two of the experiments were performed on sunny days, between March 16 and 19, 2015, at the Universidad Nacional de Chilecito (29°9′S; 67°28′W, La Rioja, Argentina). Three replicate samples were used for each of the treatments and controls.
The intensities and UVR doses to which the unicellular M. aeruginosa cells were exposed are common in temperate latitudes. The UVBR doses ranged from 41 to 75 kJ m−2. The overall UVR + PAR doses during the incubation experiments ranged from 9700 to 11
200 kJ m−2. Incident solar radiation was monitored continuously during the experiment using a radiometer (model BIC 250, Biospherical Instruments, Inc.), which records irradiances at three wavelengths in the ultraviolet region (305, 320 and 380 nm, approx. 10 nm bandwidth). This radiometer was calibrated against the reference instrument (RGUV)32 and inter-compared with the GUV 511 sited at INGEBI (Buenos Aires during the experiments). Data were recorded every minute at a site located close to the experimental setup. The equation from Orce and Helbling33 is used for calculating UVBR doses expressed in kJ m−2. UVAR and PAR irradiances were monitored continuously using a spectroradiometer (model ILT 950, International Light Technologies, Inc., USA). Using it, data (provided in μW cm−2 s−1) were recorded every minute at a site next to the BIC 250 radiometer. The calibration was done by International Light Technologies some weeks before the start of the experiments and intercalibration was done with GUV 511 from INGEBI during the experiments.
Sampling and sample analyses
The experiments started at 9 h. The aliquots of culture samples at time 0 and after the incubation time (8–9 h) were taken and the following determinations were made: Chlorophyll a (Chla) analyses, cell counts, ROS detection, CAT activity, MC and photosynthesis measurements.
At the initial time and after the incubation period of solar radiation exposure, aliquot samples (3 ml) for cell counts were taken, kept in dark bottles and fixed with formalin previously neutralized with sodium borate (final concentration 0.4% w/v). In addition, aliquot samples (15 ml) for 2′,7′-dichlorodihydrofluorescein diacetate (DCF-DA) oxidation rate analysis, used for in vivo ROS detection, as well as Chla (15 ml), MCs (40 ml), SOD activity (15 ml) and CAT activity (15 ml) analyses, were filtered using a GF/F fiber glass filter. Those meant for in vivo measurements (DCF-DA) were evaluated immediately and those meant for the measurement of MCs (40 ml), Chla, CAT activity and SOD activity (see above) were kept at −20 °C until analysis.
Chla analyses and cell counts.
Pre-filtered Chla samples were extracted using 4 ml absolute methanol. Absorbance readings of the extracts (24 h later) were used to calculate the Chla concentration, after correction of phaeopigments34 and calibration with standard Chla with a PG spectrophotometer (model P11).35 For enumeration of cyanobacteria, cells were analysed with a phase contrast Olympus inverted microscope, according to the procedures described by Villafañe and Reid36 using a Sedgwick-Rafter counting chamber. In order to separate the colonies into single cells, the samples were previously sonicated (approximately 10 W; 30 s) using an ultrasonic homogenizer (US50; Nissei Co., Tokyo, Japan).
DCFH-DA oxidation rate
Membrane-permeable non-fluorescent DCFH-DA oxidation has been used for detecting several ROS in biological media.37 DCFH-DA was initially thought to be useful as a specific indicator of H2O2. However, it has already been demonstrated that H2DCF is oxidized by other ROS, including superoxide anion radical, hydroxyl radical, peroxyl, alkoxyl, hydroperoxyl and peroxynitrite, which are products of normal metabolism.38
DCFH-DA is a fluorogenic probe which passes through cell walls and membranes and is cleaved by cellular esterases. During incubation, DCFH-DA is hydrolysed, by means of intracellular hydrolytic deacetylation, to H2DCF, which is trapped inside the cell due to its polarity. This substance is then rapidly oxidized to the highly fluorescent compound DCF that allows the evaluation of cellular toxicity.
M. aeruginosa cells obtained from filtered samples (14 ml filtered on GF/F filters) were incubated in vivo in the dark for 30 min in 2 ml of 40 mM Tris-HCl buffer (pH 7.0), in the presence of 5 μM DCFH-DA at 27 °C.37 The fluorescence of the supernatant (without cells) was monitored in a microplate reader (Beckman counter DTX 880, Multimode Detectors) with excitation (λex) at 498 nm and emission (λem) at 525 nm. In all cases, parallel blank controls were included.
CAT and SOD activity
For the CAT activity, cells harvested in GF/F filters were suspended in 5 ml of ice-cold 0.1 M potassium phosphate buffer pH 7.0, sonicated in an ice-water bath and clarified by centrifugation at 10
000g for 10 min at 4 °C. The CAT activity was evaluated as the decomposition rate of hydrogen peroxide (H2O2) at 240 nm at 25 °C.39 One unit of CAT is defined as the amount of enzyme catalyzing the elimination of 1 mM H2O2 per minute.
For the SOD activity, cell extracts were obtained using the same process as that used for the CAT assay. The SOD activity was measured using a SOD assay kit (Cayman Chemicals). One unit is defined as the amount of enzyme needed to exhibit 50% of dismutation of the superoxide radical.
Photosynthesis measurements
Subsamples of the cultured cyanobacteria (100 ml) were placed in independent (not used for ROS, Chla, MC, SOD or CAT analysis) experimental quartz tubes (with Teflon-lined screw caps), and 0.04 ml of 50 μCi 14C-bicarbonate were added to each tube following Steeman Nielsen.40 The tubes were then placed horizontally on black anodized aluminum frames and exposed to solar radiation in a water bath (25–26 °C, described in the Experimental set-up).
After the incubation period, the samples were filtered through Whatman GF/F glass fiber filters (25 mm); the filters were placed in scintillation vials, exposed to HCl fumes for 3–4 h, and dried overnight in a vented hood. Readings of 14C incorporated by cyanobacteria were carried out by liquid scintillation counting using a Packard Liquid Scintillation Analyzer Model 1600 TR (Canberra, Australia). The scintillation cocktail used was OptiPhase “Hisafe” 3, LKB Wallac. The rates of carbon fixation were expressed as Assimilation Numbers (mg C per mg Chl-a per h).
MC HPLC analysis
Cell samples (15 ml) were broken by 3 frozen–unfrozen cycles followed by 30 min ultrasonication (Omni ruptor 400), and then were centrifuged for 15 min at 5000 rpm to eliminate cell debris. The supernatant was passed through conditioned (10 ml 100% methanol, 50 mL 100% distilled water) Sep-Pak C18 cartridges (Waters). The MCs were eluted with 80% methanol. Quantitative chromatographic analysis of MCs was performed by HPLC with a photodiode array detector (LC-20A, SPD-M20A, Shimadzu Scientific Instruments, Columbia, MD, USA) and C18 column (Thermo ODS-Hypersil, 150 × 4.60 mm, 5 μm). The column was equilibrated with a mixture composed of 65% of A solution [water with 0.05% (v/v) trifluoroacetic acid] and 35% of B solution [acetonitrile with 0.05% (v/v) trifluoroacetic acid]. The mobile phase consisted of a discontinuous gradient of A and B solutions. The flow rate was 1.0 ml min−1. MCs were identified on the basis of their UV spectra and retention time. The standard of MC-LR was purchased from Sigma (St Louis, MO, USA).
Effect of UVR on different physiological parameters of M. aeruginosa
The relative photosynthesis variation (RPV) and the relative variation in biomass (RBiomassV) due to UVR were calculated as follows:
RPV, RBiomassVUVAR (%) = (PAR-TUVA) × 100/PAR |
RPV, RBiomassVUVBR (%) = (TUVA-TUVR) × 100/PAR |
The variation in ROS concentration (RROSV) and the variation in SOD and CAT activities (RSODV and RCATV, respectively) were calculated as follows:
RROSV, RSODV, RCATVUVAR (%) = (TUVA-PAR) × 100/PAR |
RROSV, RSODV, RCATVUVBR (%) = (TUVR-TUVA) × 100/PAR |
where PAR, TUVA and TUVB denote the measurements of the respective parameter under each of the irradiance treatment.
Statistical analyses
One-way ANOVA analyses and then a Tukey test were performed (Statistica, version 9) to determine the significance of the differences observed between the treatments for each parameter value during the experiments at different solar irradiance. Normality was verified using a Kolmogorov–Smirnov test.41
Results
M. aeruginosa biomass and abundance
The initial number of cells was, on average, 8 × 105 cells per ml. The effect of solar radiation on the biomass of M. aeruginosa was evaluated at the end of the experiment.
The effect of UVBR on RPV and RBiomassV was estimated from the difference between UVAR + PAR and UVR + PAR. For experimental UVBR doses lower than 65 kJ m−2 (a UVR + PAR dose of 9776 kJ m−2) there were no differences in biomass (cells per ml) between the treatments (p > 0.05). For UVBR doses between 65.7 and 67.9 kJ m−2 (UVR + PAR doses between 9875 and 10
275 kJ m−2), there were significant differences between the treatments (p < 0.05) (Fig. 1A).
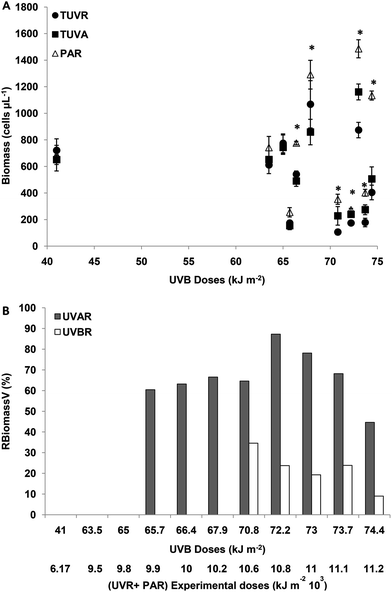 |
| Fig. 1 (A) M. aeruginosa biomass (cells per μl) after the incubation period as a function of solar doses when cells were exposed to: TUVR (UVBR + UVAR + PAR); TUVA (UVAR + PAR) and PAR. Each point represents the mean ± sd. Significant (Tukey test) differences between the treatments are marked with * for p < 0.05. (B) Relative biomass variation (RBiomassV) in percentage (%) calculated according to experimental biomass (cell per μL) as a function of incubation solar doses. RBiomassV indicates the increase in biomass calculated as a percentage of control PAR treatment considered as 100%. The bars show the statistically significant increase in biomass induced by UVAR and/or UVBR denoted in (A). | |
The effect of UVBR on the cell count was evaluated using an RBiomassV index and by determining the difference between TUVR and TUVA as was defined in Materials and methods. There were no differences in the cell number between TUVR and TUVA for UVB doses lower than 65 kJ m−2 (Fig. 1B). For UVB doses between 65 and 67.9 kJ m−2, there was no UVBR inhibition (Fig. 1B) because no significant differences were found (p > 0.05) between the TUVA and TUVR treatment (Fig. 1A). The UVAR inhibition for such a UVB dose range was on average 65%. Such results clearly show a decrease in biomass by exposure to UVAR and no effects for UVBR. For UVBR doses higher than 70.8 kJ m−2 (a UVR + PAR dose of 10
674 kJ m−2) significant differences were observed between the three radiation treatments (p < 0.01) (Fig. 1A). The percentage of relative UVA inhibition was similar in average to those calculated for intermediate UVR doses, however, the UVBR inhibition was 20% on average (Fig. 1B). Such results show that the UVBR as well as UVAR diminish the M. aeruginosa biomass and that it is not possible to determine a dose-dependent effect within this range of irradiances. However, a significant decrease was observed in biomass inhibition for both UVBR and UVAR on exposure to the highest doses (Fig. 1B).
ROS
For experimental UVBR doses lower than 65 kJ m−2 (a UVR + PAR dose of 9776 kJ m−2), there were no differences in cellular ROS concentration between the treatments (p > 0.05). For UVBR doses between 65.7 and 67.9 kJ m−2 (UVR + PAR doses between 9875 and 10
275 kJ m−2), there were significant differences between the treatments (p < 0.01), ROS concentrations being significantly higher in UVA treatments compared with PAR (Fig. 2A). Such increments of ROS concentrations as a consequence of exposure to UVAR reach a maximum of 390% on average (RROSV) at a UVR + PAR dose of 9975 kJ m−2. When the UVBR effect was null or negative, the ROS concentration was higher in the TUVA treatment compared to the TUVR treatment (Fig. 2B). For UVBR doses higher than 70.8 kJ m−2 (UVR + PAR doses of 10
674 kJ m−2), significant differences were observed between the three radiation treatments (p < 0.01) (Fig. 2A). However, the UVAR produced a higher RROSV (290%) compared with UVBR (200%). A lower ROS increment in both UVAR and UVBR treatments was observed at maximum exposure doses, compared with lower doses. No differences were found between both the treatments (around 110%) (Fig. 2B).
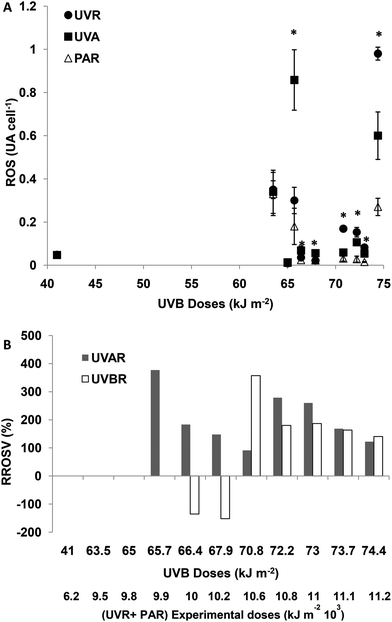 |
| Fig. 2 (A) ROS (UA per cell) after the incubation period as a function of solar doses when cells were exposed to: TUVR (UVBR + UVAR + PAR); TUVA (UVAR + PAR) and PAR. Each point represents the mean ± sd. Significant (Tukey test) differences between the treatments are marked with * for p < 0.05. (B) Relative ROS (RROSV) variation in percentage (%), calculated according to the experimental ROS concentration as a function of incubation solar doses. RROSV indicates the increase in ROS calculated as a percentage of control PAR treatment considered as 100%. The bars show the statistically significant increase in ROS induced by UVAR and/or UVBR denoted in (A). | |
SOD activity
For experimental UVBR doses lower than 65 kJ m−2 (a UVR + PAR dose of 9776 kJ m−2), there were no differences in SOD activity between the treatments (p > 0.05). For UVBR doses between 65.7 and 67.9 kJ m−2 (UVR + PAR doses between 9875 and 10
275 kJ m−2), there were significant differences between the treatments (p < 0.05) (Fig. 3A). The SOD activity was significantly higher in cells exposed to UVAR compared with PAR (p < 0.01) reaching a maximum increment (RSODV) of 620% on average at a UVR + PAR dose of 10
075 kJ m−2. In cells exposed to UVBR there was a negative increment, which means that the SOD activity was lower under TUVR (probably due to a consumption in the presence of UVBR) compared with the TUVA treatment (Fig. 3B). For UVBR doses higher than 70.8 kJ m−2 (a UVR + PAR dose of 10
674 kJ m−2), significant differences were observed only for the TUVA treatment compared to TUVR or PAR (p < 0.01) (Fig. 3A). The SOD activity was higher in cells exposed to UVAR reaching a maximum of 300% (RSODV) for a UVR + PAR dose of 10
674 kJ m−2 (Fig. 3B).
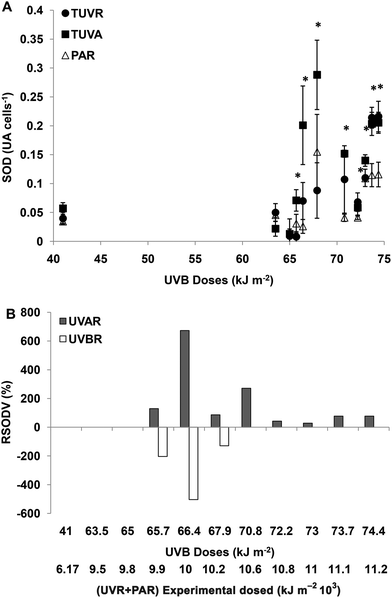 |
| Fig. 3 (A) SOD activity (UA per cells) after the incubation period as a function of solar doses when cells were exposed to: TUVR (UVBR + UVAR + PAR); TUVA (UVAR + PAR) and PAR. Each point represents the mean ± sd. Significant (Tukey test) differences between the treatments are marked with * for p < 0.05. (B) Relative SOD variation (RSODV) in percentage (%) calculated according to the experimental SOD activity as a function of incubation solar doses. RSODV indicates the increase in SOD calculated as a percentage of control PAR treatment considered as 100%. The bars show the statistically significant increase in SOD induced by UVAR and/or UVBR denoted in (A). | |
CAT activity
For experimental UVBR doses lower than 65 kJ m−2 (a UVR + PAR dose of 9776 kJ m−2), there were no differences in CAT activity between the treatments (p > 0.05). For UVBR doses between 65.7 and 67.9 kJ m−2 (UVR + PAR doses between 9875 and 10
275 kJ m−2), there were significant differences between the treatments (p < 0.05) (Fig. 4A). The CAT activity was only increased in cells exposed to UVAR reaching a maximum of 70% on average (RCATV) at a UVR + PAR dose of 10
275 kJ m−2 (Fig. 4B). Such results clearly show an increased CAT activity by exposure to UVAR and no effects of UVBR. For UVBR doses higher than 70.8 kJ m−2 (a UVR + PAR doses 10
674 kJ m−2), significant differences were observed between the three radiation treatments (Fig. 4A). Cells exposed to UVBR showed the maximum CAT activity reaching on average an increment of 110% (RCATV) for UVBR doses of 70.8 and 72.2 kJ m−2 and decreasing for higher experimental doses. In cells exposed to UVAR, however, the trend was towards increased activity with higher irradiance, with a maximum at a UVR + PAR dose of 11
173 kJ m−2, an average of 80% of increment in CAT activity (Fig. 4B).
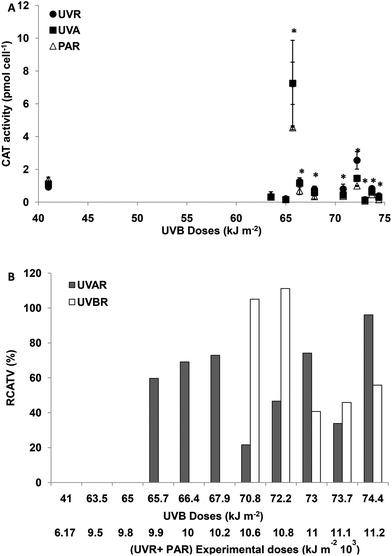 |
| Fig. 4 (A) CAT activity (UA per cell) after the incubation period as a function of solar doses when cells were exposed to: TUVR (UVBR + UVAR + PAR); TUVA (UVAR + PAR) and PAR. Each point represents the mean ± sd. Significant (Tukey test) differences between the treatments are marked with * for p < 0.05. (B) Relative CAT variation (RCATV) in percentage (%) calculated according to the experimental CAT activity as a function of incubation solar doses. RCATV indicates the increase in CAT calculated as a percentage of control PAR treatment considered as 100%. The bars show the statistically significant increase in CAT induced by UVAR and/or UVBR denoted in (A). | |
Photosynthesis
At 65.7 and 67.9 kJ m−2 UVBR doses, no significant differences between TUVR and TUVA treatments were found for the assimilation number; however, in both treatments, they were significantly lower compared to PAR (Fig. 5A). Consequently, UVBR photosynthesis inhibition was determined (Fig. 5B). At higher UVR doses, the assimilation number was significantly lower in both UVR treatments compared to PAR, but it is lower in UVB than in UVA treatments (Fig. 5A). The RPV was increased, being higher at 72.2 and 74.4 kJ m−2 UVBR doses with 25% inhibition of the photosynthetic rate on average (Fig. 5B). The photosynthesis inhibition produced by UVAR was higher compared to that produced by UVBR corresponding to 50% on average from 66.4 to 74.4 kJ m−2 of UVBR doses (Fig. 5B).
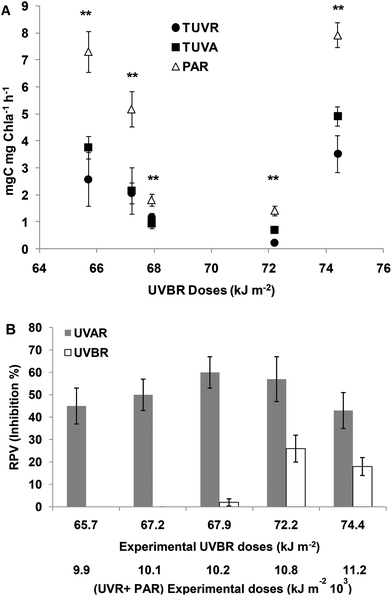 |
| Fig. 5 (A) Assimilation numbers (mg C per mg Chla per h) after the incubation period as a function of solar doses when cells were exposed to: TUVR (UVBR + UVAR + PAR); TUVA (UVAR + PAR) and PAR. Each point represents the mean ± sd. Significant (Tukey test) differences between the treatments are marked with * for p < 0.05 and ** for p < 0.01. (B) The bars show the statistically significant relative photosynthesis inhibition (%) induced by UVAR and/or UVBR as a function of incubation doses denoted in (A). | |
MC concentration
The most abundant MC was [Leu1] MC-LR. It was expressed as the amount of toxin per cell (quota Q[Leu1] MC-LR) with levels between 0.1 and 80 fg per cell (expressed as MC-LR equivalents) after the incubation period in different experiments analyzed. With UVBR doses between 41 and 73 kJ m−2, significant differences between the treatments were not found. However, a decreased quota trend for cells exposed to TUVA treatments was observed. Q[Leu1] MC-LR decreased significantly (p < 0.05) in cells exposed to TUVR and TUVA treatments compared to the control (Fig. 6) at a UVBR dose of 74.4 kJ m−2.
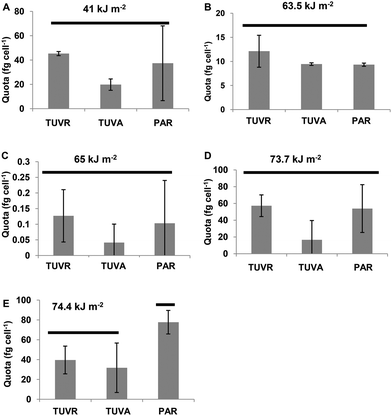 |
| Fig. 6 Effect of solar experimental doses after the incubation period on Quota [Leu1]MC-LR (fg per cell) in each experimental treatment. Each bar represents the mean ± sd. The same level of horizontal bars for each UVBR dose shows no significant differences at the p < 0.05 level by the Tukey test. | |
Discussion
The response of organisms including cyanobacteria to stress is the production of ROS,8,42 the photosynthetic process being an important source of ROS for photosynthetic organisms.43 We determine a UVBR threshold dose of 67.9 kJ m−2 (a UVR + PAR dose of 10
275 kJ m−2), below which no significant increment in ROS and no significant biomass decrease were observed in M. aeruginosa. The absence of cell damage in the TUVB treatment for low and moderate UVBR doses may be attributable to the highly efficient repair of DNA lesions in cyanobacteria in the presence of UVAR and PAR.44 A UVBR dose of 108 kJ m−2 induced a significant increase of ROS compared with lower doses with a maximum concentration at 647 kJ m−2 in Anabaena sp.8 An important clarification to be considered is that the relatively low cell concentration used in our experiments avoids a considerable self-shading of the cells exposed to UVR.
For UVBR doses higher than 67.9 kJ m−2 (a UVR + PAR dose of 10
275 kJ m−2), the ROS content increased significantly in the cells exposed to UVBR and UVAR, producing a significant biomass decrease. The main reason for the quick dissolution of cells exposed to high UVR doses is most likely a result of acute physiological stress and chronic depression of key physiological processes that resulted in rapid cellular necrosis.45 A similar observation was reported by Singh et al.,42 who applied high UVBR irradiance and found significantly decreased biomass accumulation in Phormidium foveolarum and Nostoc muscorum by inhibiting the process of photosynthesis caused by the UV-induced formation of ROS.9,18 ROS may act as a signal and/or secondary messenger enabling cyanobacteria to regulate the expression of a number of genes, resulting in protection from environmental stresses, especially UV irradiance.9,46 A decrease in the cell number of M. aeruginosa was reported after the exposure to 11 kJ m−2 of UVBR,45 however in such experiments, the cells were not exposed to UVAR nor PAR during the incubation with UVBR avoiding the possible repairing processes by UVAR and PAR.44–46 In addition, growth inhibition was shown as a consequence of UVBR exposure in M. aeruginosa, using long incubation periods (10 days) and cumulative doses of 146 and 210 kJ m−2, and no effects were observed when using a cumulative dose of 75 kJ m−2.47
Living organisms have developed several defense mechanisms to protect themselves against ROS damage.18 While some of these defense mechanisms are enzymatic (CAT, SOD and peroxidases),50 others are non-enzymatic (glutathione, vitamins A, C, E, carotenoids, etc.), and furthermore, they may repair DNA damage.51 When the balance between oxidant levels and antioxidant production is lost, the organisms have to face oxidative stress that generates a variety of damage.
H2O2, O2˙− and lipid hydroperoxides are considered to be suitable signaling species for initiating functional modulation avoiding disruption of the cellular integrity, which allows organisms to adapt to the stress conditions.52 H2O2 is the most stable ROS and, to avoid damage caused by it in cyanobacteria, these have evolved various enzymes that are able to detoxify this compound. CAT is one of the most-studied enzymes that exclusively dismutate H2O2.53 In our experiments, the CAT activity increased for cells exposed to UVAR, with UVR + PAR doses between 9776 and 10
275 kJ m−2, as well as for exposure to UVBR and UVAR with UVBR doses higher than 67.9 kJ m−2 (a UVR + PAR dose of 10
275 kJ m−2). In fact, the maximum increment in CAT activity for the cells exposed to UVAR was coincident with a low inhibition of biomass and a significant decrease in ROS concentration for UVAR and UVBR at the maximum experimental UVR doses. One of the possible reasons for the increased CAT activity in the cells exposed to UVAR for intermediate UVR doses (UVR + PAR doses between 9875 and 10
275 kJ m−2) is the activity of SOD. Disproportionation of O2− to H2O2 and oxygen is catalyzed by SOD, and the dismutation of H2O2 to oxygen and water is catalyzed by CAT. SOD activity was elevated only for the exposure to UVAR when cells were exposed to UVR + PAR doses higher than 9776 kJ m−2 (UVBR doses higher than 65 kJ m−2). Low SOD activity or consumption of it was observed for the cells exposed to intermediate UVBR doses, but no significant differences were observed at high UVBR doses. Consequently, this could mean that there was no O2˙− production for the exposure to UVBR at high UVBR doses. Another possible explanation for the lack of increased SOD activity during UVBR exposure could be the O2˙− reduction to OH−via the Fenton reaction which could cause highly damaging effects.12 Such a reaction would be produced considering the high Fe concentration in the BG11 culture medium. Nomura et al.54 showed that CAT and peroxidase in Synechococcus sp. PCC 7002 mutants and the wild-type were relatively unaffected compared to the increase in SOD activity. The significantly higher CAT activity in the cells exposed to UVBR for doses higher than 67.9 kJ m−2 shows a high H2O2 concentration which could be produced not only by the activity of SOD, but also by an indirect UVBR effect with increased ROS, particularly the H2O2 content. For intermediate UVBR doses the SOD consumption, coincident with a decrease of ROS, which may prevent cell damage, was observed. For higher UVR doses, UVAR exhibits similar effects of decreased biomass, as UVBR did, as indicated by the induction of ROS. Exposure of cyanobacteria to UVAR has been found to cause oxidative stress by producing ROS via photosensitized reactions,9 damaging the photosynthetic apparatus, decreasing survival and inhibiting growth.9,55 In our work, only PAR did not induce oxidative stress or photoinhibition compared to the initial exposure time (data not shown). This is because the PAR dose used in our study was not at high irradiance.
The differential type of response of plants to UVBR was demonstrated, which is dependent on its irradiance.56,57 High UVBR irradiance produces ROS and may cause damage to DNA, proteins and lipids, while low irradiance may produce a protective response against other stresses.56 However, it was reported that low UVBR irradiance can serve as a signal to regulate plant growth and development,58 without damaging effects. The lack of UVBR effects for low and moderate doses (between 65.7 and 67.9 kJ m−2) could be explained considering the high enzyme activity determined or an increased de novo synthesis of molecular antioxidants, such as ascorbic acid and carotenoids (not measured in our study).
There was no significant decrease of biomass or significant increase of ROS cellular content in the cells exposed to UVAR at experimental doses lower than 9776 kJ m−2 (UVR + PAR). For UVR doses between 9776 and 10
275 kJ m−2 (UVR + PAR), the UVAR produced a significant decrease in biomass and a higher ROS content compared to the PAR treatment. This can be due to the fact that there is a larger amount of UVAR reaching the Earth because it is not influenced by the depletion of stratospheric ozone.59
UVBR is a highly variable environmental signal, and fluctuations in irradiance will probably modulate the level of ROS and photoprotective signaling molecules, as well as the induction of UV-stress proteins.9 In order to accurately perceive the light environment and prevent damage caused by toxic light exposure, cyanobacteria possess photoreceptors, such as phytochromes, UVAR/blue photosensors and still undefined photoreceptive systems for mediation of responses to UVBR.60 The differential antioxidant responses and ROS production on exposure to UVR observed in our study could be the result of photoreceptive systems and other enzymatic or non-enzymatic antioxidants not measured by us.
Another consequence of the loss of balance between oxidant levels and antioxidant production is the photosynthesis inhibition. The photosynthesis inhibitory effect was absent for UVBR exposure to low and moderate doses (between 65.7 and 67.2 kJ m−2), despite the fact that, at higher doses, damage had been observed. It appears that the adaptive response of the photosynthetic function occurred and the repair system for photosynthetic damage is efficient when using UVBR doses lower than 65.7 kJ m−2. PS II is very sensitive to changes in the environment and may decline under unfavorable environmental conditions.61 The extent of the repair of PS II is determined by the rate of synthesis of the D1 protein de novo.62 Suppression of the de novo synthesis of the D1 protein by 1O2 and H2O2 was demonstrated in Anabaena sp.63 and in Synechocystis.64 While UVBR acts directly on DNA and proteins and thus damages them, UVAR is thought to cause long-term photosensitized oxidation.65 The light-saturated photosynthetic rates of M. aeruginosa 854, exposed to 15 kJ m−2 of UVBR doses, were significantly lower than the unexposed controls. This higher sensitivity, compared with that observed in our experiments, may be due to the fact that M. aeruginosa 854 has been exposed only to UVB compared with the exposure to the complete solar spectrum in our study. UVB-induced damage can be repaired by photoreactivation with UVA42,47 as well as by the low and middle regions of PAR.48 Photosynthesis inhibition and increased oxidative stress by exposure of M. aeruginosa to UVBR for a long incubation period (10 days) and at an accumulative dose of 210 kJ m−2 were recently reported.49 UVAR inhibition of primary production can be observed in the experiments for all UVBR doses, reaching an average of around 50%. It was significantly higher than the inhibition produced by UVBR starting at 67.9 kJ m−2 dose (UVR + PAR doses of 10
275 kJ m−2), of an average of 15%. Even though UVBR is more effective per energy unit,13 and, hence, potentially more damaging than those at longer wavelengths, many studies conducted in different locations have shown that UVAR is responsible for most of the photosynthetic inhibition just because its natural levels are much higher.14,15
Another adaptive way to avoid the damage due to an increased ROS concentration is the presence of MCs. There is scientific evidence that under oxidative stress, MC producers, compared with no producers, have a comparative advantage as MC acts as a protein-modulating metabolite and protectant, increasing the fitness of their host.66 Under our experimental conditions, the short-term exposure of M. aeruginosa to UVAR produced a significant [Leu1] MC-LR decrease (p < 0.05) when the UVR + PAR doses reached 11
173 kJ m−2 (a UVBR dose of 74.4 kJ m−2). In addition, for such doses there was observed a lower ROS concentration as well as a decreased biomass inhibition. For lower doses, a tendency of decreased MC quota was observed when the cells were exposed to UVAR. In other studies, it was demonstrated that several environmental factors have influenced the biosynthesis of cyanotoxins for several defined isolates. Kaebernick et al.67 proposed that the MC synthetase gene cluster is regulated by the light quality, either directly or via another regulatory factor, and that transcription requires different thresholds of light intensity for initiation and upregulation. Dziallas and Grossart27 and Hernando et al.21 showed that MCs weaken the detrimental effect of H2O2 on M. aeruginosa and proposed a function of cyanobacterial toxins as radical scavengers relevant for cyanobacterial growth. Yang and Kong49 showed a decreased mycD transcription after the exposure of M. aeruginosa to high UVBR doses (210 kJ m−2) and a long period of incubation, whereas lower irradiance stimulated the expression (75 kJ m−2 accumulative doses after 10 days of exposure). As more pieces of evidence supporting the role of MC related to oxidative stress, several studies showed an increased sensitivity of MC-deficient mutants under high light and oxidative stress conditions.67–69 In addition, Briand et al.70 observed a decrease in the MC cell quota in the late exponential growth phase, probably due to an increase in MC binding to proteins in senescent cultures that accumulate ROS.
Finally, an interesting property of several cyanobacteria is their capacity to overcome UVR damage using UV-absorbing/screening compounds as a third line of defense (not measured in our study). The results obtained by Sommaruga et al.71 suggest that the bloom-forming Microcystis utilizes direct UV sunscreen compounds such as MAAs. However, Jiang and Qiu72 were not able to detect the presence of UV-absorbing compounds in M. aeruginosa strain 384.
Conclusion
The cosmopolitan distribution of cyanobacteria shows that they can cope with a wide spectrum of environmental stresses such as heat, cold, desiccation, salinity, nitrogen starvation, photo-oxidation and osmotic stress. During the present investigation we have successfully attempted our proposed hypothesis. We found that antioxidant enzymes were more active at high UVR doses in short-term exposure. However, different responses were activated depending on the exposure to UVAR or UVBR and the dose levels. No effects were observed on the biomass, ROS production or increased activity of SOD and CAT compared to the control when UVR + PAR doses were lower than 9875 kJ m−2. For intermediate doses, oxidative stress and resistance were imparted through SOD and CAT in the cells exposed to UVAR. Despite such responses biomass decrease and photosynthesis inhibition were observed, but no effects were observed with the exposure to UVBR. At the highest doses, the solar UVR caused decreased photosynthesis and biomass with the only activation of CAT for UVBR and SOD and CAT for UVAR. In addition, significant decreases of [Leu1] MC-LR were observed as a consequence of UVAR. This was in agreement with the hypothesis of MC as an ROS scavenger. The metabolic characteristics of M. aeruginosa in solar UVR exposure and the differential enzymatic antioxidant and MC responses have proven to be useful in assessing the underlying biochemical mechanisms of UVR damage and acclimation. It is evidence of some internal cellular mechanisms in UVR-mediated signaling pathways in the context of the UVR perception mechanism. UVR signaling is an important but poorly understood aspect of light responsiveness in cyanobacteria, at the molecular and biological levels. Therefore, further study is necessary to improve our understanding of cellular signaling processes associated with UV-induced cell death and survival strategies in cyanobacteria.
Our results provide new perspectives on the influence of UV on aquatic ecosystems, and on its impact on population dynamics and photosynthesis.
Conflicts of interest
There are no conflicts to declare.
Acknowledgements
This study was supported by grants from the University of Buenos Aires, ANPCyT and CONICET. We are thankful to Dr S. Díaz who shared the Biospherical Inc. radiometer and Dr. Opezzo-Costa and her group for laboratory support. We would especially like to thank the personnel from Universidad de Chilecito for fieldwork and laboratory support. We thank two anonymous reviewers who, with their comments and suggestions, helped us to improve this manuscript.
References
- G. Pérez, S. Doldán, O. Borsani and P. Irisarri, Differential Response to Moderate UV-B Irradiation of Two Heterocystous Cyanobacteria Isolated from a Temperate Ricefield, Adv. Microbiol., 2012, 2(01), 37 CrossRef.
- J. B. Kerr and C. T. McElroy, Evidence for large upward trends of ultraviolet-B radiation linked to ozone depletion, Science, 1993, 262(5136), 1032 CAS.
- S. Solomon, D. J. Ivy, D. Kinnison, M. J. Mills, R. R. Neely and A. Schmidt, Emergence of healing in the Antarctic ozone layer, Science, 2016, 353, 269–274 CrossRef CAS PubMed.
-
I. Chorus and J. Bartram, Toxic cyanobacteria in water: a guide to their public health consequences, monitoring and management, World Health Organization/E&FN Spon/Routledge, London, 1999 Search PubMed.
-
R. L. Oliver and G. G. Ganf, Freshwater blooms, in The ecology of cyanobacteria, Springer, Netherlands, 2000, pp. 149–194 Search PubMed.
- F. Garcia-Pichel, Solar ultraviolet and the evolutionary history of cyanobacteria, Orig. Life Evol. Biosph., 1998, 28(3), 321 CrossRef CAS PubMed.
- R. P. Rastogi, S. P. Singh, A. Incharoensakdi, D. P. Häder and R. P. Sinha, Ultraviolet radiation-induced generation of reactive oxygen species, DNA damage and induction of UV-absorbing compounds in the cyanobacterium Rivularia sp. HKAR-4, S. Afr. J. Bot., 2014, 90, 163 CrossRef CAS.
- Y. Y. He and D. P. Häder, UV-B-induced formation of reactive oxygen species and oxidative damage of the cyanobacterium Anabaena sp.: protective effects of ascorbic acid and N-acetyl-L-cysteine, J. Photochem. Photobiol., B, 2002, 66(2), 115 CrossRef CAS.
- Y. Y. He and D. P. Häder, Involvement of reactive oxygen species in the UV-B damage to the cyanobacterium Anabaena sp, J. Photochem. Photobiol., B, 2002, 66(1), 73 CrossRef CAS.
- A. Hargreaves, F. A. Taiwo, O. Duggan, S. H. Kirk and S. I. Ahmad, Near-ultraviolet photolysis of β-phenylpyruvic acid generates free radicals and results in DNA damage, J. Photochem. Photobiol., B, 2007, 89(2), 110 CrossRef CAS PubMed.
- I. Vass, E. Turcsányi, E. Touloupakis, D. Ghanotakis and V. Petrouleas, The mechanism of UV-A radiation-induced inhibition of photosystem II electron transport studied by EPR and chlorophyll fluorescence, Biochemistry, 2002, 41(32), 10200 CrossRef CAS PubMed.
- A. Latifi, M. Ruiz and C. C. Zhang, Oxidative stress in cyanobacteria, FEMS Microbiol. Rev., 2009, 33(2), 258 CrossRef CAS PubMed.
-
M. Blumthaler and A. R. Webb, UVR climatology, in UV effects in aquatic organisms and ecosystems. Comprehensive Series in Photochemical and Photobiological Sciences, ed. E. W. Helbling and H. E. Zagarese, The Royal Society of Chemistry, Cambridge, 2003, pp. 21–58 Search PubMed.
- M. P. Hernando, G. F. Malanga and G. A. Ferreyra, Oxidative stress and antioxidant defences generated by solar UV in a Subantarctic marine phytoflagellate, Sci. Mar., 2005, 69(S2), 287 CrossRef CAS.
- M. Hernando, I. Schloss, S. Roy and G. Ferreyra, Photoacclimation to Long-Term Ultraviolet Radiation Exposure of Natural Sub-Antarctic Phytoplankton Communities: Fixed Surface Incubations Versus Mixed Mesocosms, Photochem. Photobiol., 2006, 82(4), 923 CrossRef CAS PubMed.
- M. Ehling Schulz and S. Scherer, UV protection in cyanobacteria, Eur. J. Phycol., 1999, 34(4), 329 CrossRef.
- J. A. Imlay, Pathways of oxidative damage, Annu. Rev. Microbiol., 2003, 57(1), 395 CrossRef CAS PubMed.
- A. A. Gorman and M. A. Rodgers, Current perspectives of singlet oxygen detection in biological environments, J. Photochem. Photobiol., B, 1992, 14(3), 159 CrossRef CAS.
- H. Qian, S. Yu, Z. Sun, X. Xie, W. Liu and Z. Fu, Effects of copper sulfate, hydrogen peroxide and N-phenyl-2-naphthylamine on oxidative stress and the expression of genes involved photosynthesis and microcystin disposition in Microcystis aeruginosa, Aquat. Toxicol., 2010, 99(3), 405 CrossRef CAS PubMed.
- G. C. Dismukes, V. V. Klimov, S. V. Baranov, Y. N. Kozlov, J. DasGupta and A. Tyryshkin, The origin of atmospheric oxygen on Earth: the innovation of oxygenic photosynthesis, Proc. Natl. Acad. Sci. U. S. A., 2001, 98(5), 2170 CrossRef CAS PubMed.
- M. Hernando, C. Houghton, L. Giannuzzi, B. Krock, D. Andrinolo and G. Malanga, Oxidative stress in Microcystis aeruginosa as a consequence of global climate change, Biocell, 2016, 40(1), 23 Search PubMed.
- P. T. Orr and G. J. Jones, Relationship between microcystin production and cell division rates in nitrogen-limited Microcystis aeruginosa cultures, Limnol. Oceanogr., 1998, 43(7), 1604 CrossRef CAS.
- R. Kurmayer, E. Dittmann, J. Fastner and I. Chorus, Diversity of microcystin genes within a population of the toxic cyanobacterium Microcystis spp. in lake Wannsee (Berlin, Germany), Microb. Ecol., 2002, 43(1), 107 CrossRef CAS PubMed.
- B. Sedmak and T. Eleršek, Microcystins induce morphological and physiological changes in selected representative phytoplanktons, Microb. Ecol., 2005, 50(2), 298 CrossRef CAS PubMed.
- M. Yoshida, T. Yoshida, Y. Takashima, N. Hosoda and S. Hiroishi, Dynamics of microcystin-producing and non-microcystin-producing Microcystis populations is correlated with nitrate concentration in a Japanese lake, FEMS Microbiol. Lett., 2007, 266(1), 49 CrossRef CAS PubMed.
- A. M. Hotto, M. F. Satchwell, D. L. Berry, C. J. Gobler and G. L. Boyer, Spatial and temporal diversity of microcystins and microcystin-producing genotypes in Oneida Lake, NY, Harmful Algae, 2008, 7(5), 671 CrossRef CAS.
- C. Dziallas and H. P. Grossart, Increasing oxygen radicals and water temperature select for toxic Microcystis sp, PLoS One, 2011, 6(9), 25569 Search PubMed.
- L. Giannuzzi, B. Krock, M. C. CrettazMinaglia, L. Rosso, C. Houghton, D. Sedan, G. Malanga, M. Espinosa, D. Andrinolo and M. Hernando, Growth, toxin production, active oxygen species and catalase activity of Microcystis aeruginosa (Cyanophyceae) exposed to temperature stress, Comp. Biochem. Physiol., Part C: Toxicol. Pharmacol., 2016, 189, 22 CrossRef CAS PubMed.
- L. Rosso, D. Sedan, M. Kolman, J. Caixach, C. Flores, J. M. Oteiza, G. Salerno, R. Echenique, L. Giannuzzi and D. Andrinolo, Microcystisaeruginos strain [D-Leu1] Mcyst-LR producer, from Buenos Aires province, Argentina, J. Coastal Life Med., 2014, 2(4), 287 CAS.
- R. Rippka, J. Deruelles, J. B. Waterbury, M. Herdman and R. Y. Stanier, Generic assignments, strain histories and properties of pure cultures of cyanobacteria, Microbiology, 1979, 111(1), 1 Search PubMed.
- M. P. Hernando and G. A. Ferreyra, The effects of UV radiation on photosynthesis in an Antarctic diatom (Thalassiosira sp.): Does vertical mixing matter?, J. Exp. Mar. Biol. Ecol., 2005, 325(1), 35 CrossRef CAS.
- S. Diaz, C. Camilion, G. Deferrari, H. Fuenzalida, R. Armstrong, C. Booth, A. Paladini, S. Cabrera, C. Casiccia, C. Lovengreen, J. Pedroni, A. Rosales, H. Zagarese and M. Vernet, Ozone and UV radiation over southern South America: climatology and anomalies, Photochem. Photobiol., 2006, 82, 834 CrossRef CAS PubMed.
- L. V. Orce and E. W. Helbling, Latitudinal UVR/PAR measurements in Argentina: extent of the “ozone hole”, Glob. Planet. Change, 1997, 15, 113 CrossRef.
- O. Holm Hansen, C. J. Lorenzen, R. W. Holmes and J. D. Strickland, Fluorometric determination of chlorophyll, ICES J. Mar. Sci., 1965, 30(1), 3–15 CrossRef CAS.
- S. T. Jeffrey and G. F. Humphrey, New spectrophotometric equations for determining chlorophylls a, b, c1 and c2 in higher plants, algae and natural phytoplankton, Biochem. Physiol. Pflanz., 1975, 167(2), 191 CrossRef CAS.
-
V. E. Villafañe and F. M. H. Reid, Métodos de microscopía para la cuantificación del fitoplancton, in Manual de métodos ficológicos, Universidad de Concepción, Concepción, 1995, p. 169 Search PubMed.
- R. E. McDowell, C. D. Amsler, D. A. Dickinson, J. B. McClintock and B. J. Baker, Reactive oxygen species and the Antarctic macroalgal wound response, J. Phycol., 2014, 50(1), 71 CrossRef CAS PubMed.
-
B. Halliwell and J. M. Gutteridge, Free Radicals in Biology and Medicine, Oxford University Press, New York, 2007 Search PubMed.
-
E. Beutler, Catalase, in Red Cell Metabolism a Manual Of Biochemical Methods, ed. E. Beutler, Grune and Stratton, Inc, 1982, p. 105 Search PubMed.
- N. Steeman, The use or radiocarbon (14C) for measuring organic production in the sea, J. Cons. Int. Explor. Mer., 1952, 18, 117–140 CrossRef.
-
S. M. Scheiner, Multiple response variables and multi-species interactions, in Design and analysis of ecological experiments, ed. S. M. Scheiner and J. Gurevitch, Chapman & Hall, New York, 2nd edn, 2001, p. 99 Search PubMed.
- S. P. Singh, D. P. Häder and R. P. Sinha, Cyanobacteria and ultraviolet radiation (UVR) stress: mitigation strategies, Ageing Res. Rev., 2010, 9(2), 79 CrossRef CAS PubMed.
- V. P. Singh, P. K. Srivastava and S. M. Prasad, Differential effects of UV-B radiation fluence rates on growth, photosynthesis, and phosphate metabolism in two cyanobacteria under copper toxicity, Toxicol. Environ. Chem., 2012, 94(8), 1511 CrossRef CAS.
- D. P. Häder, H. D. Kumar, R. C. Smith and R. C. Worrest, Effects of solar UV radiation on aquatic ecosystems and interactions with climate change, Photochem. Photobiol. Sci., 2007, 6, 267 Search PubMed.
- Y. Ding, L. Song and B. Sedmak, UVB radiation as a potential selective factor favoring microcystin producing bloom forming cyanobacteria, PLoS One, 2013, 8(9), 73919 Search PubMed.
- N. Blot, D. Mella Flores, C. Six, G. Le Corguillé, C. Boutte, A. Peyrat and L. Garczarek, Light history influences the response of the marine cyanobacterium Synechococcus sp. WH7803 to oxidative stress, Plant Physiol., 2011, 156(4), 1934 CrossRef CAS PubMed.
- A. L. Dany, T. Douki, C. Triantaphylides and J. Cadet, Repairof the main UV-induced thymine dimeric lesions within Arabidopsis thaliana DNA: evidence for the major involvement of photoreactivation pathways, J. Photochem. Photobiol., B, 2001, 65, 127 CrossRef CAS.
- H. W. Paerl, J. Tucker and P. T. Bland, Carotenoid enhancementand its role in maintaining blue-green algae (Microcystis aeruginosa) surface blooms, Limnol. Oceanogr., 1983, 28, 847 CrossRef CAS.
- Z. Yang and F. Kong, UV-B Exposure affects the biosynthesis of microcystin in toxic Microcystis aeruginosa cells and its degradation in the extracellular space, Toxins, 2015, 7, 4238 CrossRef CAS PubMed.
- A. Canini, D. Leonardi and M. G. Caiola, Superoxide dismutase activity in the cyanobacterium Microcystis aeruginosa after surface bloom formation, New Phytol., 2001, 152(1), 107 CrossRef CAS.
- R. P. Sinha and D. P. Häder, UV-induced DNA damage and repair: a review, Photochem. Photobiol. Sci., 2002, 1(4), 225 CAS.
- N. Kaul and H. J. Forman, Reactive oxygen species in physiology and toxicology: from lipid peroxidation to transcriptional activation, Toxicol. Hum. Environ.: The Critical Role of Free Radicals, 2000, 311 CAS.
- P. Chelikani, I. Fita and P. C. Loewen, Diversity of structures and properties among catalases, Cell. Mol. Life Sci., 2004, 61(2), 192 CrossRef CAS PubMed.
- C. T. Nomura, T. Sakamoto and D. A. Bryant, Roles for heme–copper oxidases in extreme high-light and oxidative stress response in the cyanobacterium Synechococcus sp. PCC 7002, Arch. Microbiol., 2006, 185(6), 471 CrossRef CAS PubMed.
-
R. W. Castenholz and F. Garcia Pichel, Cyanobacterial responses to UV-radiation, in The ecology of cyanobacteria, Springer, Netherlands, 2000, p. 591 Search PubMed.
- H. Frohnmeyer and D. Staiger, Ultraviolet-B radiation-mediated responses in plants. Balancing damage and protection, Plant Physiol., 2003, 133(4), 1420 CrossRef CAS PubMed.
- B. A. Brown and G. I. Jenkins, UV-B signaling pathways with different fluence-rate response profiles are distinguished in mature Arabidopsis leaf tissue by requirement for UVR8, HY5, and HYH, Plant
Physiol., 2008, 146(2), 576 CrossRef CAS PubMed.
- Y. N. Feng, Z. C. Zhang, J. L. Feng and B. S. Qiu, Effects of UV-B radiation and periodic desiccation on the morphogenesis of the edible terrestrial cyanobacterium Nostoc flagelliforme, Appl. Environ. Microbiol., 2012, 78(19), 7075 CrossRef CAS PubMed.
- J. E. Frederick, H. F. Snell and E. K. Haywood, Solar ultraviolet radiation at the earth's surface, Photochem. Photobiol., 1989, 50, 443 CrossRef CAS.
- B. L. Montgomery, Sensing the light: photoreceptive systems and signal transduction in cyanobacteria, Mol. Microbiol., 2007, 64(1), 16 CrossRef CAS PubMed.
- B. Demmig-Adams and W. W. Adams Iii, Photoprotection and other responses of plants to high light stress, Annu. Rev. Plant Biol., 1992, 43(1), 599 CrossRef CAS.
- E. M. Aro, I. Virgin and B. Andersson, Photoinhibition of photosystem II. Inactivation, protein damage and turnover, Biochim. Biophys. Acta, Bioenerg., 1993, 1143(2), 113–134 CrossRef CAS.
- C. I. Sicora, S. E. Appleton, C. M. Brown, J. Chung, J. Chandler, A. M. Cockshutt, I. Vass and D. A. Campbell, Cyanobacterial psbA families in Anabaena and Synechocystis encode trace, constitutive and UVB-induced D1 isoforms, Biochim. Biophys. Acta, Bioenerg., 2006, 1757(1), 47 CrossRef CAS PubMed.
- Y. Nishiyama, S. I. Allakhverdiev, H. Yamamoto, H. Hayashi and N. Murata, Singlet oxygen inhibits the repair of photosystem II by suppressing the translation elongation of the D1 protein in Synechocystis sp. PCC 6803, Biochemistry, 2004, 43(35), 11321 CrossRef CAS PubMed.
- M. M. Caldwell, J. F. Bornman, C. L. Ballaré, S. D. Flint and G. Kulandaivelu, Terrestrial ecosystems, increased solar ultraviolet radiation, and interactions with other climate change factors, Photochem. Photobiol. Sci., 2007, 6(3), 252 CAS.
- Y. Zilliges, J. C. Kehr, S. Meissner, K. Ishida, S. Mikkat, M. Hagemann, A. Kaplan, T. Börner and E. Dittmann, The cyanobacterial hepatotoxinmicrocystin binds to proteins and increases the fitness of Microcystis under oxidative stress conditions, PLoS One, 2011, 6(3), 17615 Search PubMed.
- M. Kaebernick, B. Neilan, T. Börner and E. Dittmann, Light and the Transcriptional Response of the Microcystin Biosynthesis Gene Cluster, Appl. Environ. Microbiol., 2000, 66(8), 3387 CrossRef CAS PubMed.
- S. Meissner, D. Steinhauser and E. Dittmann, Metabolomic analysis indicates a pivotal role of the hepatotoxin microcystin in high light adaptation of Microcystis, Environ. Microbiol., 2015, 17, 1497 CrossRef CAS PubMed.
- A. K. Makower, J. M. Schuurmans, D. Groth, Y. Zilliges, H. C. P. Matthijs and E. Dittmann, Transcriptomics-Aided Dissection of the Intracellular and Extracellular Roles of Microcystin in Microcystis aeruginosa PCC 7806, Appl. Environ. Microbiol., 2015, 81, 544 CrossRef PubMed.
- E. Briand, C. Yéprémian, J. F. Humbert and C. Quiblier, Competition between microcystin- and non-microcystin-producing Planktothrix agardhii (cyanobacteria) strains under different environmental conditions, Environ. Microbiol., 2008, 10, 3337 CrossRef CAS PubMed.
- R. Sommaruga, Y. Chen and Z. Liu, Multiple Strategies of Bloom-Forming Microcystis to Minimize Damage by Solar Ultraviolet Radiation in Surface Waters, Microb. Ecol., 2009, 57, 667 CrossRef PubMed.
- H. Jiang and B. Qiu, Photosynthetic adaptation of a bloom forming cyanobacterium Microcystis aeruginosa to prolonged UV-B exposure, J. Phycol., 2005, 41, 983 CrossRef.
|
This journal is © The Royal Society of Chemistry and Owner Societies 2018 |