DOI:
10.1039/C8NJ04965C
(Paper)
New J. Chem., 2019,
43, 115-120
Investigating the influence of a CrO42−/Cr2O72− template in the formation of a series of silver–chalcogenide clusters†
Received
30th September 2018
, Accepted 8th November 2018
First published on 9th November 2018
Abstract
Three new silver–chalcogenide clusters protected by naphthyl ligands are described in this work, namely [Ag14(SCH2C10H7)6(CF3COO)8(DMAc)6] (1), [Ag30(SCH2C10H7)18(CrO4)2(DMAc)2 (CF3COO)8] (DMAc = dimethylacetamide) (2) and {Ag12(SCH2C10H7)6(CF3COO)4(CH3CN)6(Cr2O7)}n (3). Their crystal structures were studied by X-ray crystallography, and they show hollow-core–shell (1), template-core–shell (2) and 1D infinite (3) structures, respectively. The luminescence properties of cluster 1 were investigated, which showed interesting thermochromic variation from red to bright yellow. Moreover, the chromate ions were proven to act as templates in forming the silver clusters through a series of experiments to prepare compounds 2 and 3, which could be considered as derivatives of cluster 1.
Introduction
Coinage metal clusters have consistently attracted researchers’ attention since F. A. Cotton introduced the term ‘cluster’ in the 1960s. Especially after the concept of M⋯M metallophilic interactions was proposed in the 1980s,1–3 research in this field blossomed in the following decades,4,5 including studies of alkynyl6–8 and chalcogenide based gold and silver clusters.9–12 Metallophilic interactions are well-established for gold(I) and silver(I) complexes, and have been proven to be efficient for extra complexity, providing a high probability of forming high-nuclearity clusters.12–16 Besides, the introduction of R–CH2S− moieties into clusters may functionalize the resulting clusters, so that they not only possess appealing structures, but also display new optical, electrical and chemical properties.17–19 For example, the dodecanuclear cluster Ag12(SCH2C10H7)6(CF3CO2)6(CH3CN)620 not only exhibits fascinating luminescence emission, but also shows thermochromic variation from red to bright yellow.
Our group has been committed to studies of Ag–S clusters.20–25 We have found that the preparation of clusters with specific metal numbers is hardly controlled, because such processes always involve the intricate assembly of multiple components.26 However, recent research progress shows that if anion templates are introduced, such preparations could be comparatively predictable.27–29 Various anion templates, such as halogen (F−, Cl−, Br−, and I−), sulfide30,31 and polyatomic (NO3−, CrO42−, SO42−, CO32−, pyrovanadate, and polyoxometalate) anions, have been found in high-nuclear silver clusters.32–39 Their superiority in forming huge clusters has been summarized by Prof. Wang,26 who reported that an anion template could not only control the size and shape of a cluster, but could also stabilize it. Moreover, functionalized anions could also bring interesting physical properties to the system. For example, the [Mo6O22]8− ion conferred electronic communication behavior on the [Ag60(Mo6O22)2(C
CtBu)38](CF3SO3)632 and [Ag40(C
CtBu)20(CF3COO)12(Mo6O22)]·2CH3OH clusters,6 and the [W6O21]6− ion lent both luminescence and electrochemical properties to [Ag34(StBu)26(W6O21)(CF3COO)](CF3COO)·Et3N·20CH3OH at ambient temperature.19
In previous studies, we successfully introduced the polyoxomolybdate anion [Mo20O66]12− into Ag–S systems to obtain a giant peanut-like 62-core silver–thiolate nanocluster.21 In order to study the influence of the template on the structure and properties of a cluster, we are willing to try other anions. Chromate anions show flexible coordination modes, such as μ6-η2,η2,η2 and μ12-η3,η3,η3,η3, which makes them perfect anionic candidates, but their roles as templates for silver clusters are scarcely reported. To our knowledge, few silver clusters with chromate anion templates have been reported to date.28,35,40,41 Wang et al. reported two unprecedented chromate-templated Ag22 and Ag35 silver–alkynyl clusters;35 C. W. Liu et al. reported a Ag16 silver–dithiophosphate cluster.28
Herein, we report the syntheses and crystal structures of Ag14(SCH2C10H7)6(CF3COO)8(DMAc)6 (1) and its derivatives based on the chromate and dichromate ions, Ag30(SCH2C10H7)18(CrO4)2(DMAc)2(CF3COO)8 (DMAc = dimethylacetamide) (2) and {Ag12(SCH2C10H7)6(CF3COO)4(CH3CN)6(Cr2O7)}n (3), respectively. The cluster 1 has a core–shell structure with a nanoscale size. In the presence of K2Cr2O7, we obtained cluster 2 and polymer 3. Cluster 1 shows a peanut-shaped structure with two CrO42− ions at the core. Polymer 3 shows a 1D structure; the [Ag12S6] clusters extend through the bridging dichromate. Dichromate ions play a predominant template function in forming cluster 2 and polymer 3. Studies of the luminescence properties of cluster 1 reveal that cluster 1 shows interesting thermochromic variation from red to bright yellow.
Results and discussion
Crystal structure of [Ag14(SCH2C10H7)6(CF3COO)8(DMAc)6] (1)
Single-crystal X-ray diffraction analysis revealed that cluster 1 crystallizes in the orthorhombic space group Pbca, in which fourteen silver(I) ions are aggregated through a linkage of six −SCH2–naphthyl ligands and stabilized by eight CF3COO− and six DMAc auxiliary ligands (Fig. 1(a)). All the S atoms come from −SCH2–naphthyl ligands. As shown in Fig. 1(b), the S1 atoms adopt a μ5-η1:η1:η1η1:η1 coordination mode, linking Ag1, Ag2, Ag3, Ag4 and Ag7 to build a compressed Ag5S pentagonal pyramid, which is made up of a silver pentagonal base and five Ag5S triangle faces. The S2 and S3 atoms both adopt a μ4-η1:η1:η1η1 ligation mode, linking four silver(I) ions (Ag2, Ag4, Ag5 and Ag6–S2; Ag1, Ag3, Ag5 and Ag6–S3) to build compressed Ag4S square pyramids. Layers also can be used to describe the whole structure. As shown in Fig. 1(b), in the first layer, there are three silver ions (Ag2, Ag3 and Ag6) arranged in a triangle (with Ag⋯Ag distances of 2.915(2), 3.009(1) and 3.103(2) Å), and the S atoms are shared by each pair of silver ions on the side of the Ag-triangle like a traditional checkerboard. The Ag–S distances fall in the range of 2.475(2)–2.599(2) Å. Correspondingly, the other set of atoms (Ag2#, Ag3#, Ag6# and S) forms another checkerboard in the opposite direction with a distance of 3.745(2) Å to the first one. An eight-membered (Ag1–Ag7–Ag4–Ag5)2 ring is inserted between these two checkerboard-like layers, which are stabilized through Ag–S bonds (with Ag–S distances of 2.495(3)–2.756(2) Å) and Ag⋯Ag argentophilic interactions (2.946(2)–3.390(2) Å). The diameter of the eight-membered Ag8 ring is ca. 8.111(3) × 9.037(7) Å2 with respect to the Ag5⋯Ag5# and Ag7⋯Ag7# distances, respectively. Meanwhile, the [Ag14S6] core is further stabilized by the DMAc and CF3COO− ligands, with Ag–O distances in the range of 2.203(9)–2.582(9) Å. All the CF3COO− anionic ligands have μ2-O′:O′′ bridging mode; as for the six DMAc molecules, two have mono-O and the other four adopt a μ2-O mode. Taking into account the expansion of the ligands, the size of the tetradecanuclear Ag–S cluster 1 can reach the nanoscale, with dimensions of ca. 15 × 20 × 20 Å3, which has been proven by TEM experiments (Fig. S1, ESI†).
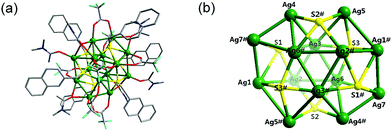 |
| Fig. 1 (a) General description of cluster 1 protected by different ligands. (b) The inner core framework, [Ag14S6], in which all the protecting ligands are eliminated for clarity. The hydrogen atoms have been omitted for clarity. Color legend: green, Ag; yellow, S; blue, N; red, O; turquoise, F; gray, C. | |
Crystal structure of [Ag30(SCH2C10H7)18(CrO4)2(DMAc)2 (CF3COO)8] (2)
The addition of K2Cr2O7 in the synthesis of cluster 2 produced a peanut-shaped cage, which was templated by two in situ generated CrO42− ions. The IR vibration at 906 cm−1 confirmed the presence of the CrO42− ions, and the band at 1656 cm−1 was assigned to the CF3COO− anion. The molecular structure of cluster 2 determined by single-crystal X-ray diffraction data is illustrated in Fig. 2(a). Selected bond lengths and angles are presented in Table S2 (ESI†). Cluster 2 crystallizes in the monoclinic space group P
, and the cage is templated by two CrO42− ions and protected by 18 peripheral −SCH2–naphthyl ligands, eight CF3COO− ligands, and two DMAc molecules (Fig. 2(b)). The −SCH2–naphthyl ligands adopt two coordination modes, μ3-η1:η1:η1 and μ4-η1:η1:η1η1. In the μ3-η1:η1:η1 mode, sulfur atoms (S1, S3, S4 and S6) link three Ag(I) ions, forming a compressed Ag3S trigonal pyramid with a Ag3 triangle base and three Ag2S triangle faces. Sulfur atoms (S2, S5, S7, S8 and S9) bind Ag(I) ions in the μ4-η1:η1:η1η1 mode, forming a compressed Ag4S square pyramid with four silver ions at the base. All these trigonal and square pyramids form the peanut-shape cage by sharing their Ag-corners. The Ag–S distances fall in the reasonable range of 2.413(2)–2.651(2) Å, which are comparable to the reported values.35,42 The −SCH2–naphthyl ligands stick out of the [Ag30S18] shell with angles of ca. 16° and 24° with respect to the Ag3 and Ag4 bases. Additionally, eight CF3COO− anions and two DMAc molecules finish the protection of the [(CrO42−)2@Ag30S18] core by coordinating to Ag(I) ions with Ag–O distances of 2.193(4)–2.555(5) Å. This shows that the presence of CF3COO− and DMAc in the system enhances the stability of the high-nuclear [(CrO42−)2@Ag30S18] cage.6
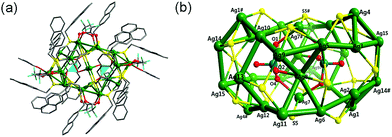 |
| Fig. 2 (a) The framework of cluster 2 templated by two CrO42− ions. (b) The inner core, [(CrO42−)2@Ag30S18], in which all the protecting ligands are eliminated for clarity. The hydrogen atoms have been omitted for clarity. Color legend: green, Ag; yellow, S; blue, N; red, O; turquoise, F; gray, C. | |
The silver ions can be divided into 10 types according to their coordination environment, taking into account the Ag⋯Ag contacts. Ag1, Ag2, Ag3, Ag4, Ag9, Ag10, Ag11 and Ag12 have a [Ag–S2OAg3] coordination environment; Ag13 has a [Ag–S2Ag3] environment; the other Ag ions have Ag5, Ag6–[Ag–S3OAg3]; Ag14, Ag15–[Ag–S2O2Ag3], Ag7–[Ag–S2O2]; and Ag8–[Ag–SOAg4] environments, respectively. These silver ions show diverse irregular coordination geometries. The Ag–Ag distances (from 2.869(7) to 3.343(7) Å) show typical argentophilic interactions, which are significantly shorter than twice the van der Waals radius (ca. 3.44 Å) of the silver atom.43,44 The whole cluster is completed by the linkage of CrO42− ions with Ag(I) inside the silver core. Two template CrO42− ions are encapsulated in the [Ag30S18] cage, and coordinate to Ag(I) ions in the μ3-η1:η1:η1 mode. The Ag8–O2, Ag7–O4 and Ag7#–O1 distances are 2.205(7), 2.484(1) and 2.457(2) Å, respectively, indicating strong contacts with the [Ag30S18] shell. The CrO42− ions have tetrahedral geometry, as they always appear to, but with a slight distortion with τ = 0.8 (for an ideal tetrahedron, τ4 = 1),45 and the O–Cr–O angles range from 96.542(7)° to 136.727(8)°. Two template chromate ions share two silver ions, forming an 8-membered ring with a chair conformation. The whole structure has pseudo-twofold symmetry, and the inversion center is situated at the center of the 8-membered ring. The [(CrO42−)2@Ag30S18] cage has an outer diameter of ca. 22 × 26 × 19 Å3, taking the −SCH2–naphthyl ligands into account. The inner core [(CrO42−)2@Ag30S18] has a diameter of ca. 13 × 8 × 10 Å3 with respect to the Ag14⋯Ag14#, S5⋯S5# and Ag9⋯Ag9# distances, respectively.
Such Ag–O distances can be compared to the clusters [Ag22(C
CtBu)18(CrO4)](BF4)2, [Ag35(C
CPh)28(CrO4)2(TMEDA)4](BF4)335 and [Ag16(CrO4) {S2P(OEt)2}12]2(PF6)4.28 Such a condensed skeleton also benefits the stability of this huge [(CrO42−)2@Ag30S18] cluster in air.
Crystal structure of {Ag12(SCH2C10H7)6(CF3COO)4(CH3CN)6(Cr2O7)}n (3)
The X-ray structural analysis of 3 reveals the existence of a [Ag12S6] cluster connected by dichromate ions forming a 1D polymer. The [Ag12(SCH2C10H7)6(CF3COO)4(CH3CN)6]2− cluster (Fig. 3(a)) in 3 greatly resembles Ag12(SCH2C10H7)6(CF3COO)6(CH3CN)6.† The Ag–Ag distances in 3, which range from 2.960(1) to 3.239(1) Å, indicate argentophilicity.
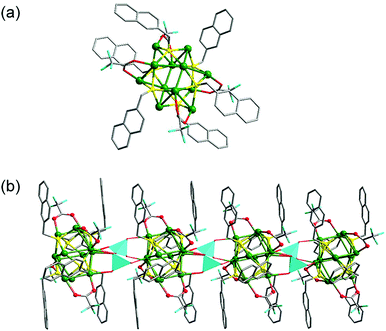 |
| Fig. 3 (a) The dodecanuclear cluster unit Ag12(SCH2C10H7)6(CF3COO)6(CH3CN)6 of polymer 3. (b) 1D chain formed by the extension of the dodecanuclear cluster unit through dichromate ions. The hydrogen atoms have been omitted for clarity. The turquoise tetrahedra represent the dichromate ions. Color legend: green, Ag; yellow, S; blue, N; red, O; turquoise, F; gray, C. | |
The disordered trifluoroacetate groups display μ2-bridging mode in association with two silver centers. Acetonitrile is a terminal ligand in monodentate coordination mode. The dichromate ions show μ6-mode. The terminal O atoms coordinate to two silver atoms in one Ag12S6 cluster; the other pair of O atoms in the dichromate ion bind silver atoms in the neighboring Ag12S6 cluster; and the bridging O8 atom links two Ag12S6 clusters simultaneously (Fig. 3(b)). The dichromate connectors join one Ag12S6 cluster to two others in this way, thus developing a 1D coordination polymer along the a axis. The S atoms in the −SCH2–naphthyl ligand all adopt μ4-mode. The Ag–S distances in the Ag12S6 cluster range from 2.421(2) to 2.706(2) Å, which are in the normal interval for Ag(μ4-SR).
Dichromate ion templating
In order to confirm the templating function and origin of the CrO42− ions, several comparative experiments were performed. Without the addition of K2Cr2O7, we obtained the 14-nuclearity core–shell cluster 1, which indicates that the anionic CrO42− template is very important for the formation of 2. DMAc also plays an important role in the self-assembly. It not only coordinates to Ag(I) as an auxiliary ligand to stabilize the final structure, but also acts as a weak base to favor the transformation of Cr2O72− to CrO42−. We also attentively added different sources of chromate (Na2CrO4, K2CrO4, (NH4)2CrO4) to the reaction mixture in the preparation of 2, but no crystals were obtained, which indicates that the slow introduction of CrO42− into the reaction system could avoid the formation of insoluble Ag2CrO4 and is very important for the formation of 2.
For cluster 3, Cr2O72− but not CrO42− ions exist as templates in the absence of DMAc, which indicates that the basic DMAc influences the form of the template. The template function of the dichromate ions also can be proven by a comparison of cluster 3 and the reported cluster Ag12(SCH2C10H7)6(CF3COO)6(CH3CN)6.20 Both clusters contain a dodecanuclear [Ag12S6] cluster inner core protected by C10H7CH2S−, CF3COO− and acetonitrile, but cluster 3 forms a 1D polymer in the presence of Cr2O72− ions. In cluster 3, the Cr2O72− ions adopt a μ6-η1:η1:η2:η1:η1 coordination mode, and connect [Ag12S6] cluster units in an infinite chain. The Cr–Ot distances fall into the range of 1.588–1.597 Å, which are comparable to those in free dichromate ions (1.62 Å),46 and the Cr–Ob distance of 1.777(8) Å is also a little shorter than that in free Cr2O72− ions (1.80 Å).
The O atoms of the encapsulated CrO42− in cluster 2 and of the intercluster Cr2O72− ions in polymer 3 show significant interactions with the Ag atoms because of the high affinity of silver for O donors. The average Ag–O distances of 2.35 Å (in 2) and 2.58 Å (in 3) show comparatively strong interactions.
On one hand, chromate ions introduce new clusters; on the other hand, the existence of chromate and dichromate ions greatly enhances the stability of the resultant clusters, due to their high capacities for extra electrons from the Ag-clusters.
Morphological studies
The morphologies of single crystals of clusters 1 and 2 in ethanol were investigated using TEM images. Fig. S1 (ESI†) shows that cluster 1 exists as discrete particles with an average size of about 2 nm, which is close to the size described above. The TEM image of cluster 2 reveals that discrete particles exist, with an identified size of about 3.0 nm (Fig. S2, ESI†), which is close to the above description of [(CrO42−)2@Ag30S18]. Some other bigger particles may be attributed to the aggregation of several clusters.
Optical properties
The phase purity of 1 was confirmed by the corresponding powder X-ray diffraction (PXRD) patterns (Fig. S3, ESI†). The UV-vis spectrum of 1 in the solid state at room temperature shows broad absorption from 200 nm to 450 nm (Fig. S4, ESI†), where two distinct absorption peaks can be found at about 350 nm and 400 nm. The high-energy (HE) absorption at ca. 350 nm can be assigned to the transition of the ligand involving the π orbitals of the naphthyl ring. The low-energy (LE) absorption at ca. 400 nm is assigned to the S 3p → Ag 5s transition, as a similar assignment was made for a hypothetical Ag2S monomer.47 The broad absorption suggests that more than one excited state may be populated.48
Powder X-ray diffraction (PXRD) patterns (Fig. S5, ESI†) showed the purity of cluster 2. The absorption spectrum of a red powder sample of 2 was measured in the solid state at room temperature. As shown in Fig. S6 (ESI†), the broad higher energy absorption band in the range of 200–400 nm could be ascribed to the intraligand π–π* transition of the naphthyl rings. The absorption peak from 400 to 600 nm is consistent with the dark-red color of the crystals. The intense low-energy band in the visible region can reasonably be assigned to the charge transfer from the S 3p to the Ag 5s orbitals. As expected, with the existence of the CrO42− species, the luminescence is quenched for cluster 2.
In order to clarify the luminescence characteristics of cluster 1, we extended the test conditions from 293 K to 93 K. The results show that no detectable luminescence emission was observed at room temperature, while a slight dual emission could be found at lower temperatures and the intensity increases with decreasing temperature. Upon cooling from 293 K to 93 K under 360 nm excitation, the HE emission bands of cluster 1 show a slight blue-shift (from 590 and 540 nm to 560 and 530 nm, respectively) with a continuous increase in intensity, which becomes dominant at 93 K, as shown in Fig. 4. The emission of 1 at low temperatures may be caused by the enhanced rigidity, which effectively reduces the loss of energy by non-radiative decay.42 The HE emissions at 530 nm and 560 nm can be ascribed to the excimer-like emission from a naphthyl-based excited state that is involved in aromatic stacking.20 On the other hand, the LE emission bands were constant at 660 nm with a comparatively slow increase in intensity, which is attributed to the S 3p → Ag 5s charge transfer in the cluster. Such luminescence is very similar to that of our previously reported cluster, Ag12(SCH2C10H7)6(CF3COO)6 (CH3CN)6,20 which also shows interesting dual emission.
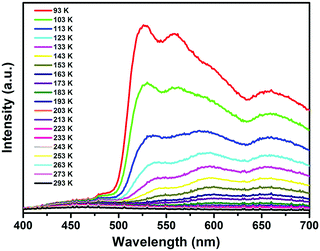 |
| Fig. 4 The temperature-dependent luminescence spectra of cluster 1 excited at 360 nm in the solid state, showing prominent dual emission characteristics. | |
Experimental
General procedures
All the starting materials were of analytical grade as obtained from commercial sources, and used without further purification. The ligand 2-(methylthio)naphthalene (HSCH2C10H7) was synthesized by a reported procedure.20
FT-IR spectra were recorded using KBr pellets in the range of 4000 to 400 cm−1 on a Bruker VECTOR 22 spectrometer. Elemental analyses for C, H, N and S were performed on a Perkin-Elmer 240 elemental analyzer. Transmission electron microscopy (TEM) measurements were performed on a JEM-2100 microscope. In the preparation of samples 1 and 2 for TEM observation, the crystals were first dispersed in ethanol using an ultrasonic bath and then dropped onto a copper grid, which was dried in air at room temperature and kept under vacuum for 20 min before TEM observation. The software NANO MEASURER 1.2 was applied to calculate the particle sizes of the nanoclusters. Powder X-ray diffraction (PXRD) data for 1 and 2 was collected on a Rigaku D/Max-2500PC diffractometer with Cu-Kα radiation (λ = 1.5418 Å) over the 2θ range of 3–50° at room temperature. UV-visible absorption spectra were recorded on a TU-1900 double-beam UV-vis spectrophotometer. Luminescence spectra for the solid sample of 1 were recorded on a Hitachi 850 fluorescence spectrophotometer.
Synthetic procedures
Preparation of [Ag14(SCH2C10H7)6(CF3COO)8(DMAc)6] (1).
A mixture of 2-(methylthio)naphthalene (0.017 g, 0.10 mmol) and AgCF3COO (0.044 g, 0.10 mmol) in ethanol–DMAc (v
:
v = 1
:
1, 6.0 mL) gave pale-yellow crystals of 1 by evaporation in the dark at room temperature. The crystals were manually isolated and washed with ethyl ether and dried at room temperature. Yield: 82% (based on HSCH2C10H7). Calcd for C106H108N6O22S6F24Ag14 (Fw: 3976.52): C, 32.02; H, 2.74; N, 2.11; O, 8.85; S, 4.84%. Found: C, 32.46; H, 2.53; N, 2.32; O, 8.49; S, 4.32%. IR (KBr, cm−1): 3050 (m), 2940 (w), 1670 (s), 1420 (m), 1210 (s), 840 (s), 721 (s).
Preparation of [Ag30(SCH2C10H7)18(CrO4)2(DMAc)2(CF3COO)8] (2).
Cluster complex 2 was obtained under ambient conditions. A mixture of ethanol (3.0 mL), DMAc (3.0 mL), 2-(methylthio)naphthalene (0.017 g, 0.10 mmol) and AgCF3COO (0.044 g, 0.10 mmol) was treated with K2Cr2O7 (0.029 g, 0.01 mmol). After stirring for 15 min, the resultant solution was kept in the dark. Red crystals of 2 were obtained by evaporation at room temperature. Yield: 73% (based on HSCH2C10H7). Calcd for C222H180N2O26S18F24Cr2Ag30 (Fw: 7664.86): C, 34.79; H, 2.37; N, 0.36; O, 5.43; S, 7.53%. Found: C, 34.36; H, 2.53; N, 0.42; O, 5.69; S, 7.32%. IR (KBr, cm−1): 3056 (w), 2916 (w), 1656 (s), 1201 (s), 1130 (s), 906 (m), 836 (m), 823 (m), 748 (m).
Preparation of {Ag12(SCH2C10H7)6(CF3COO)4(CH3CN)6(Cr2O7)}n (3).
Polymer 3 was prepared by a similar procedure to cluster 2, except using acetonitrile instead of DMAc. A solution of EtOH–MeCN (3 mL
:
3 mL) containing 2-(methylthio)naphthalene (0.017 g, 0.10 mmol) and AgCF3COO (0.044 g, 0.10 mmol) was added dropwise with an ethanol solution of K2Cr2O7 (0.029 g, 0.01 mmol). The resultant solution was kept in the dark for several days. Red-orange crystals were obtained in a yield of 65% (based on HSCH2C10H7). Calcd for C43H36N3O8S3F6CrAg6 (Fw: 1632.15): C, 31.64; H, 2.22; N, 2.57; O, 7.84; S, 5.89%. Found: C, 31.36; H, 2.53; N, 2.42; O, 7.69; S, 5.62%. IR (KBr, cm−1): 3046 (w), 2926 (w), 1664 (s), 1209 (s), 1030 (s), 846 (m), 738 (m).
Conclusions
This work is focused on a series of newly designed and prepared silver–chalcogenide clusters. We systematically described their preparation, structures, and optical properties. Cluster 1 was prepared under ambient conditions, and it forms a cage-like cluster with tetradecanuclearity and shows slight dual emission fluorescence at lower temperatures. In order to explore the influence of the synthesis conditions on cluster 1, we obtained other two compounds, 30-nuclearity cluster 2 and 1D polymer 3, by making use of the templating agent dichromate. Chromate ions act as intracluster and intercluster templates in 2 and 3, respectively. Clusters 2 and 3 exhibit significantly greater stability in air than cluster 1. Our experiments prove the importance of templates in the construction of diverse clusters.
Conflicts of interest
There are no conflicts to declare.
Acknowledgements
The National Science Fund for Distinguished Young Scholars (No. 21825106); the National Natural Science Foundation of China (No. 21671175); Program for Science & Technology Innovation Talents in Universities of Henan Province (164100510005); Program for Innovative Research Team (in Science and Technology) in Universities of Henan Province (19IRTSTHN022).
Notes and references
- M. Jansen, Angew. Chem., Int. Ed. Engl., 1987, 26, 1098 (
Angew. Chem.
, 1987
, 99
, 1136
) CrossRef.
- F. Scherbaum, A. Grohmann, B. Huber, C. Krüger and H. Schmidbaur, Angew. Chem., Int. Ed. Engl., 1988, 27, 1544 (
Angew. Chem.
, 1988
, 100
, 1600
) CrossRef.
- F. Scherbaum, A. Grohmann, G. Müller and H. Schmidbaur, Angew. Chem., Int. Ed. Engl., 1989, 28, 463 (
Angew. Chem.
, 1989
, 101
, 464
) CrossRef.
- H. Schmidbaur, Chem. Soc. Rev., 1995, 24, 391 RSC.
- H. Schmidbaur and A. Schier, Chem. Soc. Rev., 2012, 41, 370 RSC.
- G. G. Gao, P. S. Cheng and T. C. W. Mak, J. Am. Chem. Soc., 2009, 131, 18257 CrossRef CAS.
- L. L. Wen, H. Wang, C. Q. Wan and T. C. W. Mak, Organometallics, 2013, 32, 5144 CrossRef CAS.
- D. Rais, J. Yau, D. Michael, P. Mingos, R. Vilar, A. J. P. White and D. J. Williams, Angew. Chem., Int. Ed., 2001, 40, 3464 CrossRef CAS.
- H. Y. Yang, Y. Wang, H. Q. Huang, L. Gell, L. Lehtovaara, S. Malola, H. Häkkinen and N. F. Zheng, Nat. Commun., 2013, 4, 2422 CrossRef PubMed.
- H. Y. Yang, Y. Wang and N. F. Zheng, Nanoscale, 2013, 5, 2674 RSC.
- G. Li, Z. Lei and Q. M. Wang, J. Am. Chem. Soc., 2010, 132, 17678 CrossRef CAS.
- C. E. Anson, A. Eichhöfer, I. Issac, D. Fenske, O. Fuhr, P. Sevillano, C. Persau, D. Stalke and J. T. Zhang, Angew. Chem., Int. Ed., 2008, 47, 1326 CrossRef CAS.
- X. J. Wang, T. Langetepe, C. Persau, B. S. Kang, G. S. Sheldrick and D. Fenske, Angew. Chem., 2002, 114, 3972 (
Angew. Chem. Int. Ed.
, 2002
, 41
, 3818
) CrossRef.
- D. Fenske, C. Persau, S. Dehnen and C. E. Anson, Angew. Chem., 2004, 116, 309 (
Angew. Chem. Int. Ed.
, 2004
, 43
, 305
) CrossRef.
- D. Fenske, C. E. Anson, A. Eichhöfer, O. Fuhr, A. Ingendoh, C. Persau and C. Richert, Angew. Chem., 2005, 117, 5376 (
Angew. Chem. Int. Ed.
, 2005
, 44
, 5242
) CrossRef.
- Z. Wang, H.-F. Su, Y.-Z. Tan, S. Schein, S.-C. Lin, W. Liu, S.-A. Wang, W.-G. Wang, C.-H. Tung, D. Sun and L.-S. Zheng, PNAS, 2017, 114, 12132 CrossRef CAS PubMed.
- J. F. Corrigan, O. Fuhr and D. Fenske, Adv. Mater., 2009, 21, 1867 CrossRef CAS.
- E. N. Hooley, V. Paolucci, Z. Y. Liao, M. R. C. Temboury and T. Vosch, Adv. Opt. Mater., 2015, 3, 1109 CrossRef CAS.
- K. Zhou, C. Qin, H. B. Li, L. K. Yan, X. L. Wang, G. G. Shan, Z. M. Su, C. Xu and X. L. Wang, Chem. Commun., 2012, 48, 5844 RSC.
- Q. Q. Xu, X. Y. Dong, R. W. Huang, B. Li, S. Q. Zang and T. C. W. Mak, Nanoscale, 2015, 7, 1650 RSC.
- R. W. Huang, Q. Q. Xu, H. L. Lu, X. K. Guo, S. Q. Zang, G. G. Gao, M. S. Tang and T. C. W. Mak, Nanoscale, 2015, 7, 7151 RSC.
- B. Li, R. W. Huang, J. H. Qin, S. Q. Zang, G. G. Gao, H. W. Hou and T. C. W. Mak, Chem. – Eur. J., 2014, 20, 12416 CrossRef CAS PubMed.
- H. Liu, C. Y. Song, R. W. Huang, Y. Zhang, H. Xu, M. J. Li, S. Q. Zang and G. G. Gao, Angew. Chem., Int. Ed., 2016, 55, 3699 CrossRef CAS PubMed.
- R. W. Huang, Y. S. Wei, X. Y. Dong, X. H. Wu, C. X. Du, S. Q. Zang and T. C. W. Mak, Nat. Chem., 2017, 9, 689 CrossRef CAS.
- Z. Y. Wang, M. Q. Wang, Y. L. Li, P. Luo, T. T. Jia, R. W. Huang, S. Q. Zang and T. C. W. Mak, J. Am. Chem. Soc., 2018, 140, 1069 CrossRef CAS.
- Q. M. Wang, Y. M. Lin and K. G. Liu, Acc. Chem. Res., 2015, 48, 1570 CrossRef CAS.
- R. Vilar, Angew. Chem., Int. Ed., 2003, 42, 1460 CrossRef CAS.
- J. H. Liao, H. W. Chang, H. C. You, C. S. Fang and C. W. Liu, Inorg. Chem., 2011, 50, 2070 CrossRef CAS.
- Y.-P. Xie, J.-L. Jin, G.-X. Duan, X. Lu and T. C. W. Mak, Coord. Chem. Rev., 2017, 331, 54 CrossRef CAS.
- Y.-P. Xie, J.-L. Jin, X. Lu and T. C. W. Mak, Angew. Chem., Int. Ed., 2015, 54, 15176 CrossRef CAS.
- J.-L. Jin, Y.-P. Xie, H. Cui, G.-X. Duan, X. Lu and T. C. W. Mak, Inorg. Chem., 2017, 56, 10412 CrossRef CAS.
- J. Qiao, K. Shi and Q. M. Wang, Angew. Chem., Int. Ed., 2010, 49, 1765 CrossRef CAS.
- S. D. Bian, J. H. Jia and Q. M. Wang, J. Am. Chem. Soc., 2009, 131, 3422 CrossRef CAS PubMed.
- R. Vilar, D. Michael, P. Mingos, A. J. P. White and D. J. Williams, Angew. Chem., Int. Ed., 1998, 37, 1258 CrossRef CAS.
- S. D. Bian, H. B. Wu and Q. M. Wang, Angew. Chem., Int. Ed., 2009, 48, 5363 CrossRef CAS PubMed.
- D. Sun, H. Wang, H. F. Lu, S. Y. Feng, Z. W. Zhang, G. X. Sun and D. F. Sun, Dalton Trans., 2013, 42, 6281 RSC.
- Z. Wang, H.-F. Su, M. Kurmoo, C.-H. Tung, D. Sun and L.-S. Zheng, Nat. Commun., 2018, 9, 2094 CrossRef.
- J.-W. Liu, L. Feng, H.-F. Su, Z. Wang, Q.-Q. Zhao, X.-P. Wang, C.-H. Tung, D. Sun and L.-S. Zheng, J. Am. Chem. Soc., 2018, 140, 1600 CrossRef CAS PubMed.
- Y.-M. Su, W. Liu, Z. Wang, S.-A. Wang, Y.-A. Li, F. Yu, Q.-Q. Zhao, X.-P. Wang, C.-H. Tung and D. Sun, Chem. – Eur. J., 2018, 24, 4967 CrossRef CAS PubMed.
- X.-Y. Li, H.-F. Su, M. Kurmoo, C.-H. Tung, D. Sun and L.-S. Zheng, Nanoscale, 2017, 9, 5305 RSC.
- S.-S. Zhang, H.-F. Su, Z. Wang, L. Wang, Q.-Q. Zhao, C.-H. Tung, D. Sun and L.-S. Zheng, Chem. – Eur. J., 2017, 23, 3432 CrossRef CAS.
- S. Yuan, Y. K. Deng, X. P. Wang and D. Sun, New J. Chem., 2013, 37, 2973 RSC.
-
J. Emsley, The Elements, Clarendon, Oxford, 1989, p. 174 Search PubMed.
- S. D. Bian and Q. M. Wang, Chem. Commun., 2008, 5586 RSC.
- L. Yang, D. R. Powell and R. P. Houser, Dalton Trans., 2007, 955 RSC.
-
C. E. Housecroft and A. G. Sharpe, Inorganic Chemistry, Pearson Education Limited, 2nd edn, 2005 Search PubMed.
- D. Bruhwiler, R. Seifert and G. Calzaferri, J. Phys. Chem. B, 1999, 103, 6397 CrossRef.
- S. Z. Zhan, M. Li, X. P. Zhou, J. H. Wang, J. R. Yang and D. Li, Chem. Commun., 2011, 47, 12441 RSC.
Footnote |
† Electronic supplementary information (ESI) available. CCDC 1869846–1869848. For ESI and crystallographic data in CIF or other electronic format see DOI: 10.1039/c8nj04965c |
|
This journal is © The Royal Society of Chemistry and the Centre National de la Recherche Scientifique 2019 |