DOI:
10.1039/C8NJ04433C
(Paper)
New J. Chem., 2019,
43, 121-129
Synthesis of dendrimer functionalized adsorbents for rapid removal of glyphosate from aqueous solution
Received
31st August 2018
, Accepted 10th November 2018
First published on 13th November 2018
Abstract
Recently, the growing abuse of glyphosate herbicides in agricultural production has caused widespread accumulation of glyphosate in the environment, which is threatening the health or life of human beings and animals. To solve this problem, novel dendrimer grafted adsorbents were proposed for the rapid and efficient removal of glyphosate from water samples. These adsorbents were synthesized by grafting poly(amidoamine) dendrimers (PAMAM) onto poly(styrene-divinyl benzene-glycidylmethacrylate) beads. The morphological structure and chemical composition of the adsorbents were investigated by SEM, the BET method, FTIR spectroscopy, XPS and elemental analysis. Batch adsorption experiments were performed in glyphosate standard solutions with different pH, temperatures, and initial concentrations. Under the optimized conditions, adsorption equilibrium was attained rapidly within 5 min. Experimental data were correlated well with the pseudo-second-order model and the Langmuir model. The effect of temperature was also discussed by the thermodynamic parameters. Unlike other glyphosate adsorbents, their adsorption capabilities could be easily controlled by varying the generation of PAMAM dendrimer used. In addition, regeneration experiments showed that the adsorbents exhibited good reproducibility. When applied in the purification of contaminated drinking water, the removal efficiency of the adsorbents could be more than 95%.
1. Introduction
Glyphosate (Gly) is a highly effective and non-selective herbicide in agricultural production. For a long time, it was considered an environmentally friendly herbicide, and thus a great amount of glyphosate was commercially produced and consumed.1 However, the continuous abuse of glyphosate herbicide led to the accumulation of residual glyphosate in water, soil and various kinds of food.2 Recently it was observed that the accumulated glyphosate has been found to threaten crop safety, ecological stability and environmental hygiene. Furthermore, glyphosate is also reported to be the cause of cancer, endocrine system diseases, neurological problems and genetic diseases in human beings.3–5 Although glyphosate was initially considered as a “harmless herbicide”, many governments and organizations have established standards for its maximum residue levels (MRLs) allowed in water. For example, in America and Canada, the MRLs of glyphosate in water are 700 μg L−1 and 280 μg L−1, respectively.6 Therefore, it is very important to remove glyphosate from contaminated water.
With increasing attention paid to the toxicity of glyphosate, various methods for the elimination of glyphosate have been reported, including oxidation,7 biodegradation,8,9 membrane filtration10,11 and adsorption. Among these methods, adsorption is recognized as a promising method on account of its low cost, simple process and environment-friendly operation.12 So far, a variety of adsorption materials have been applied for glyphosate treatment, such as activated carbon,13 iron oxide/SBA-15,14 metal–organic frameworks,15 magnetic materials16 and zeolite 4A.17 All of these materials have their own merits, but some of them also possess disadvantages such as low capacity, deregeneration and slow binding kinetics.
Recently, dendrimers have been successfully applied in various adsorbents and attracted wide attention from researchers. Dendrimers18,19 are a special kind of highly branched polymers (HPBs) with a regular tree-like structure in three dimensions. They have an initial core, broad internal cavities, controlled molecular chains and a high density of surface groups. As a result of their unique structure, dendrimers possess the characteristics of good dispersion, low toxicity, biocompatibility, excellent electrical–optical properties and easy modification. These properties have increased dendrimer applications in various fields, such as drug delivery,20 catalyst encapsulation,21 sensors22 and adsorption materials.23–27 According to published works, the first dendrimers, poly(amidoamine) (PAMAM) dendrimers, are the most popular among various dendrimers. PAMAM is the most common dendrimer with ethylenediamine as the core. Each half and whole generation of PAMAM dendrimers end with an ester group and a terminal amino group, respectively. Abundant terminal groups make PAMAM an excellent candidate for glyphosate removal. Controlled molecular chains allow PAMAM adsorbents to have controllable adsorption capabilities. In addition, terminal amino groups can be protonated in acid solutions, which helps to increase the adsorption efficiency and shorten the adsorption time of glyphosate.
However, considering its excellent dispersion behavior in water, isolation of dendrimers from well-dispersed solution is challenging. Therefore, it is impracticable to use PAMAM for adsorption directly. To overcome these problems, organic polymer particles were developed as a matrix. Generally, organic polymer particles28 are a kind of porous substrate composed of various monomers, such as acrylates, methacrylate, styrene, divinylbenzene, and glycidylmethacrylate. Due to their high stabilities and solvent compatibilities, they are widely applied as substrates of adsorbents and stationary phases.29,30 Immobilizing PAMAM on the polymer matrix can not only help to isolate PAMAM from solution easily but also have a synergistic effect on glyphosate adsorption.
In this work, novel adsorbents for the rapid and efficient removal of glyphosate were proposed by immobilizing PAMAM dendrimers onto polymer beads. The synthesized adsorbents were characterized and tested for glyphosate removal from solutions. Adsorption conditions including pH, temperature, PAMAM generation used, and adsorption time were optimized. Based on the results, the adsorption mechanism, adsorption kinetics, adsorption equilibrium and thermodynamic parameters were discussed. Regeneration and reproducibility of the adsorbents were also performed by 5 adsorption/desorption cycles. Finally, the synthesized adsorbents were applied for glyphosate adsorption from contaminated drinking water.
2. Experimental section
2.1 Materials
Polystyrene-divinylbenzene-glycidyl methacrylate (PS-GMA) particles (55% cross-linking degree) were synthesized according to the dispersion polymerization method31 and utilized as the substrate for dendrimer grafting. Ethylenediamine (AR), methyl acrylate (AR), methanol (AR) and glyphosate (99.5%) were purchased from Aladdin Chemical Co. Ltd (Shanghai, China). HCl (37.2%) and sodium hydroxide (AR) were purchased from Hushi Laboratorial Equipment Co. Ltd (Shanghai, China). Glyphosate standard solutions were prepared by dissolving an appropriate amount of glyphosate in deionized water. Contaminated water samples were obtained by adding glyphosate standard solution to drinking water. Deionized water was generated using a GenPure Pro UV/UF purifier (Thermo Fisher Scientific, USA) with a specific resistivity of 18.2 MΩ cm.
2.2 Preparation of different generation PAMAM dendrimers
PAMAM dendrimers were prepared by following the scheme of Tomalia et al.18 With ethylenediamine as a nitrogen core, PAMAM dendrimers were fabricated by alternate Michael addition and amidation reactions. Each reaction generated a half generation (G). In order to guarantee the purity of the product, the product of each reaction was purified using a rotary evaporation device. With repeated reactions, 1–3 generations of dendritic molecules were synthesized. The specific structure is shown in Fig. 1.
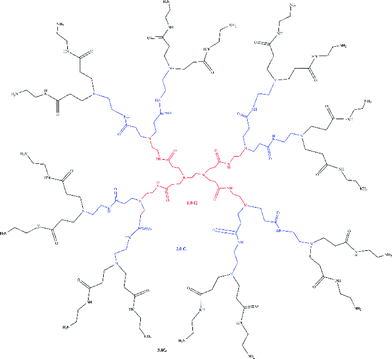 |
| Fig. 1 Structure of PAMAM dendrimers. | |
2.3 Fabrication of PAMAM grafted adsorbents
PAMAM grafted adsorbents were fabricated by an open loop reaction of PAMAM and PS-GMA particles.32 To be specific, 3.0 g PS-GMA beads and 6 mL PAMAM dendrimers were dissolved in 30 mL alcohol and ultrasonicated for 10 min. Afterwards the mixture was placed into a 100 mL round-bottom flask. The flask was put into a water bath at 70 °C for 1 h. After that, the mixture was washed with water and methanol, and the particles were finally dried under vacuum at 60 °C for 4 h. By selecting different generation PAMAM dendrimers, a series of PAMAM functionalized adsorbents were obtained. The PS-GMA beads grafted with 1.0G, 2.0G, and 3.0G PAMAM were denoted as G1-PS-GMA, G2-PS-GMA, and G3-PS-GMA, respectively.
2.4 Physical and chemical characterization
The surface features and diameter of the particles were investigated by scanning electron microscopy (SEM) and the Brunauer–Emmett–Teller (BET) method. The SEM images of PS-GMA and G1-PS-GMA particles were obtained by using Hitachi SU-70 (Hitachi Japan). The accelerating voltage used for detection was 3 kV. The surface area, pore volume and pore size of PS-GMA and G1-PS-GMA particles were investigated and compared using a surface area and porosity analyzer ASAP 2020 HD88 (Micromeritics, Atlanta, Georgia, USA).
Chemical compositions of microspheres were characterized by Fourier transform infrared spectroscopy (FTIR), X-ray photoelectron spectroscopy (XPS) and elemental analysis. Functional groups on the surface of the adsorbents were derived by using an IR spectrophotometer IS50 (Thermo Fisher Nicolet, USA), with samples in the form of KBr pellets containing a 2 wt% predried sample. The valence states of the functional groups on the surface of particles were affirmed with an ESCALAB 250Xi instrument using a magnesium anode (Mg 1253.6 eV) X-ray source. Contents of carbon, hydrogen, nitrogen and oxygen elements in PS-GMA and G1-PS-GMA particles were analyzed by utilizing an elemental analyzer (Flash EA1112, Thermofinnigan).
2.5 Adsorption experiments
Adsorption experiments were performed with glyphosate standard solutions. Before batch experiments, the pH of the solution was optimized first. The influence of pH was explored with 100 mg G1-PS-GMA adsorbents and 50 mg L−1 standard solutions at room temperature. The pH of the solutions was adjusted to be in the range of 1–5 by 0.1 mol L−1 hydrochloric acid and 0.1 mol L−1 sodium hydroxide solutions.
At the optimized pH, the effect of temperature was investigated by adsorption experiments at three different temperatures (25, 50 and 75 °C) with 50 mg G1-PS-GMA adsorbents and glyphosate standard solutions (varying from 5 mg L−1 to 100 mg L−1). The influence of PAMAM dendrimers was studied with 50 mg different adsorbents (G1-PS-GMA, G2-PS-GMA and G3-PS-GMA) in glyphosate standard solutions (5–50 mg L−1). Adsorption kinetics was performed in a 10 mL centrifuge tube containing 10 mg adsorbents and 8 mL standard solutions for varied adsorption times (0.5–30 min).
2.6 Detection and calculation
All the adsorption processes were carried out in an ultrasonic bath and finished by filtering through a 0.22 μm membrane filter. The filtrate was collected for ion chromatography (IC) analysis. Residual glyphosate concentrations in the filtrate were measured using a Thermo Fisher Scientific (Waltham, MA, USA) ICS 3000, equipped with a DP pump, a six-port valve fitted with a 25 μL sampling loop, a column heater and a DS6 heated conductivity detector. Suppression was achieved with a Thermo Fisher Scientific ASRS-4 mm suppressor. The temperature of the column was set as 30 °C. The flow rate of the eluent was 1.0 mL min−1. An IonPac AS18 (4 × 250 mm) column was selected for glyphosate determination, with 32 mmol L−1 NaOH as the eluent. Data were treated with a Chromeleon 7.2 chromatogram workstation (Thermo Fisher Scientific).
The equilibrium adsorption capability qe (mg g−1) and percentage of adsorbed glyphosate (Gly%) were calculated according to the following equations:
| 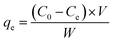 | (1) |
| 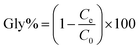 | (2) |
where
C0 (mg L
−1) and
Ce (mg L
−1) are the initial concentration and equilibrium concentration, respectively,
V (mL) is the total volume of solution, and
W (mg) is the weight of adsorbents.
3. Results and discussion
3.1 Morphological analysis of modified adsorbents
The modification of PS-GMA beads was achieved by grafting PAMAM onto the surface of the beads. The morphological structures of bare PS-GMA beads and modified adsorbents were investigated using SEM images as shown in Fig. 2. The bare PS-GMA beads were uniform and monodisperse, with an average particle size of 6 μm. The surface of the beads was smooth and the pores were uniformly distributed on the microsphere surface. After PAMAM grafting, the monodispersity still remained and the surface did not change significantly as shown in Fig. 2C and D. The result demonstrated that the grafting process almost led to no change in the morphological structure. Specific surface characterization of the adsorbent beads was also performed with nitrogen adsorption–desorption measurements according to the Brunauer–Emmett–Teller (BET) method and the results are given in Table 1. Surface area, pore volume, and pore size increased slightly after grafting modification. The higher surface area provides more adsorption and reaction sites, consequently leading to a higher adsorption capacity of the adsorbents.
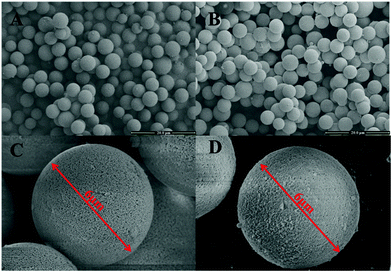 |
| Fig. 2 SEM images of PS-GMA beads (A) ×2000 times, (C) ×10 000 times and adsorbents (B) ×2000 times, (D) ×10 000 times. | |
Table 1 Porosity characteristics of adsorbents
|
Surface areaa (m2 g−1) |
Pore volumeb (cm3 g−1) |
Pore sizec (nm) |
Calculated by the Brunauer–Emmett–Teller (BET) method.
Referred to Barrett–Joyner–Halenda (BJH) adsorption cumulative volume of pores.
Referred to BJH adsorption average pore diameter.
|
Pristine PS-GMA |
209.1676 |
0.3155 |
6.4449 |
Adsorbent beads |
308.1625 |
0.4675 |
6.9294 |
3.2 Chemical composition of fabricated adsorbents
Elemental analysis, XPS and FTIR spectroscopy were carried out to elucidate the composition of the adsorbents. By grafting with different generation PAMAM dendrimers, a series of PAMAM functionalized adsorbents were synthesized. The elementary compositions of pristine beads (PS-GMA) and G1-PS-GMA, G2-PS-GMA, and G3-PS-GMA adsorbents were investigated by elemental analysis (Table 2). As there is no nitrogen element in pristine beads, nitrogen content in adsorbents is dependent on the PAMAM generation. With the increase of PAMAM generation, the proportion of the nitrogen element also increased significantly, indicating that higher PAMAM generations may bring more functional groups. Furthermore, the content of nitrogen element in adsorbents was also determined by grafting efficiency. Thus the content of nitrogen element did not increase with PAMAM generations proportionally, but only reflects the growth trend.
Table 2 Elemental analysis of adsorbents
|
Carbon (%) |
Hydrogen (%) |
Oxygen (%) |
Nitrogen (%) |
Pristine beads |
88.50 |
7.70 |
3.80 |
0 |
G1-PS-GMA |
84.69 |
7.48 |
7.41 |
0.42 |
G2-PS-GMA |
82.56 |
7.33 |
9.58 |
0.53 |
G3-PS-GMA |
77.67 |
6.97 |
14.60 |
0.76 |
XPS analysis further affirmed the states of nitrogen element in pristine beads, new adsorbents and used adsorbents. The N1s spectra exhibit two different nitrogen-containing species in the results (Fig. 3). According to the literature, the peak at 399.2 eV in new adsorbents corresponds to terminal free amines (–NH2) in grafted PAMAM dendrimers.33 In addition to the peak of free amines, another peak at 400.9 eV was also observed for used adsorbents. It resulted from the protonated amines in acidic solutions (pH = 3) during the adsorption process.34 Due to no nitrogen element in pristine beads, no N1s signals were observed in unmodified beads. The results were consistent with elemental analysis.
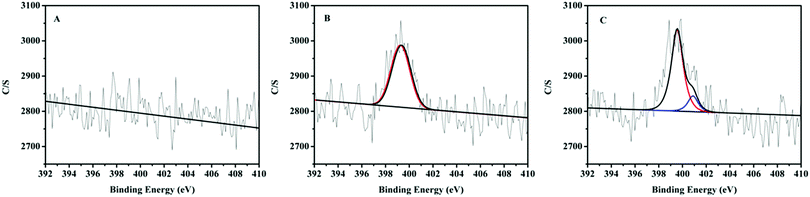 |
| Fig. 3 XPS spectra of nitrogen: (A) pristine beads, (B) new adsorbents, and (C) used adsorbents. | |
In order to estimate the presence of the functional groups in adsorbents and their involvement before and after adsorption, the new adsorbents and used adsorbents were compared by FTIR spectroscopy. It is obvious from Fig. 4 that the adsorption band between 1455 and 1605 cm−1 resulted from stretching vibration of benzene rings in polymer substrates. A significant peak at 1728 cm−1 corresponded to stretching vibrations of C
O groups in grafted PAMAM dendrimers. Absorption peaks from 3036 cm−1 to 2849 cm−1 can be assigned to the vibration of –C–H bonds. A wide band at 3421 cm−1 was attributed to the overlapping peak of –N–H and –O–H groups. Compared to new adsorbents, there are two strong peak enhancements at 3421 cm−1 and 1800–1000 cm−1 in the case of used adsorbents. It could be interpreted as the increase of the C–N bond (1100–1000 cm−1), C
O bond (1680–1750 cm−1), PO43− groups (1790 cm−1) and the –N–H bond (3000–3500 cm−1) in the adsorbents binding with glyphosate molecules.
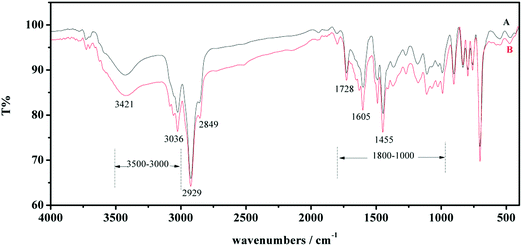 |
| Fig. 4 FTIR spectra of new adsorbents (A) and used adsorbents (B). | |
3.3 Adsorption studies
3.3.1 Effect of pH on the adsorption.
The formation of glyphosate and PAMAM grafting adsorbents highly depends on the pH of the solutions. Thus, the pH of the solutions determines the removal efficiency of glyphosate. To maximize the removal of glyphosate by the adsorbents, it is important to select an optimum pH. Therefore, pH was optimized by using 100 mg synthesized adsorbents and 50 mg L−1 glyphosate solutions. The pH of the solutions varied from 1 to 5. According to the results in Fig. 5, the glyphosate uptake was dependent on solution pH. It was observed that the residual glyphosate levels were the lowest at pH 3. In other words, the best pH for glyphosate adsorption is 3.
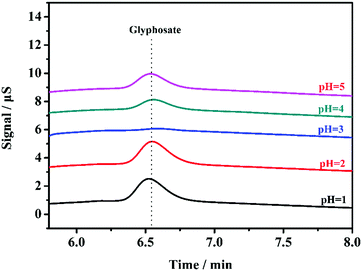 |
| Fig. 5 Chromatogram for residual glyphosate after adsorption. Columns: IonPac AS18 (250 mm × 4.0 mm); eluent: 32 mM NaOH (1.0 mL min−1); detection: suppressed conductivity. | |
According to deprotonation reactions of glyphosate at different pH, the pH of the solution may determine the major species of glyphosate. Deprotonation reactions and relative pKa values of glyphosate are shown in Fig. 6A. At the optimized pH, the dominant state of glyphosate is H2L−. Meanwhile, terminal amino groups in PAMAM dendrimers turned into positively charged protonated amino groups on the surface of adsorbents (pKa for surface NH3+ species is 7.6). Accordingly, glyphosate was adsorbed by electrostatic interactions (Fig. 6B).
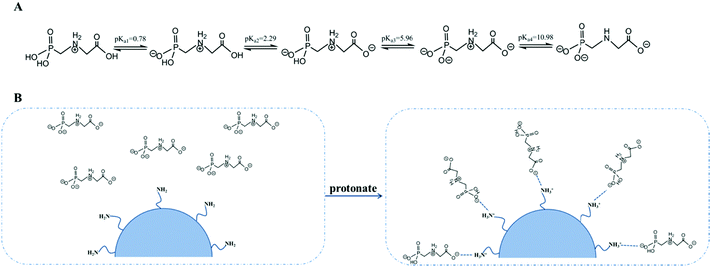 |
| Fig. 6 Deprotonation reactions of glyphosate (A) and glyphosate adsorption mechanism (B). | |
3.3.2 Effect of temperature on adsorption.
Temperature is considered one of the most important factors for adsorption efficiency. Adsorption experiments at three different temperatures (25, 50, and 75 °C) were performed by dispersing 50 mg grafting adsorbent in glyphosate solutions, while the pH of the solution was adjusted to 3. Adsorption experiments were conducted with different initial concentrations. It was observed that the adsorption capacity decreased upon increasing the temperature (Fig. 7). The result could be interpreted that glyphosate adsorption onto PAMAM grafted adsorbents is an exothermic process. Lower temperature can promote the adsorption process.
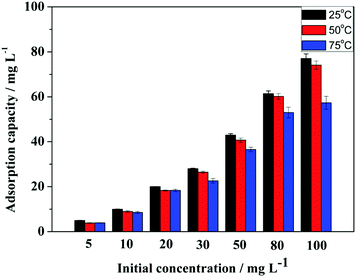 |
| Fig. 7 Adsorption capacity at different temperatures. | |
3.3.3 Effect of PAMAM dendrimers on adsorption.
With abundant terminal amino groups, PAMAM grafting onto the PS-GMA beads provided a lot of adsorptive sites. The comparison between pristine PS-GMA beads and PAMAM grafting beads for glyphosate adsorption at different initial concentrations is shown in Fig. 8. According to the result, it is obvious that the pristine PS-GMA beads almost have no adsorption of glyphosate. But PAMAM grafting beads exhibit satisfactory adsorption capability. This difference indicated that PAMAM grafting improves the adsorption performance. Besides, the adsorption efficiency of adsorbents grafted with different generation PAMAM dendrimers (G1-PS-GMA, G2-PS-GMA, and G3-PS-GMA) was also tested by glyphosate removal experiments. Adsorption percentage in Fig. 9 shows an obvious increase from G1-PS-GMA to G3-PS-GMA. This relationship is derived from the fact that adsorption capacity is controllable by varying PAMAM generations. More amino groups in higher PAMAM generations lead to higher adsorption capacity. The adsorption percentage of glyphosate can reach 100% by selecting adsorbents with high capacity.
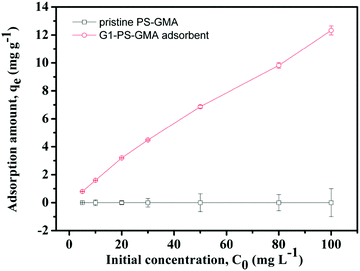 |
| Fig. 8 Adsorption efficiency of pristine PS-GMA beads and G1-PS-GMA adsorbents. | |
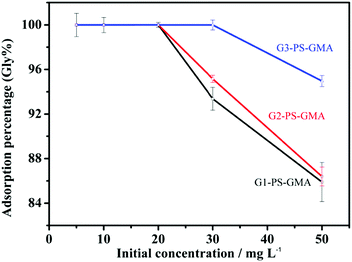 |
| Fig. 9 Adsorption efficiency of adsorbents modified by different generations of PAMAM. | |
3.4 Adsorption kinetics
With G1-PS-GMA beads as adsorbents, the impact of adsorption time was investigated. Adsorption capability over 30 min is shown in Fig. 10. According to the curve in Fig. 10A, a rapid adsorption took place at the beginning and adsorption equilibrium for glyphosate is reached within 5 min.
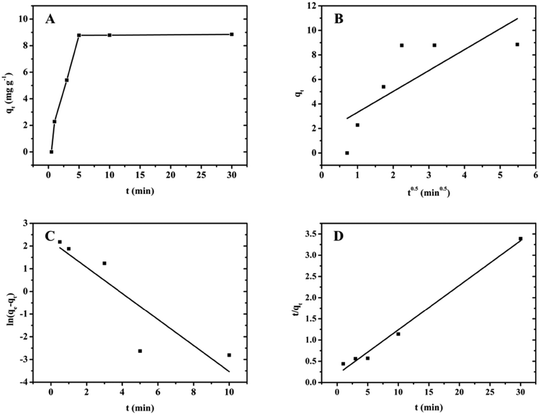 |
| Fig. 10 (A) Glyphosate adsorption kinetics by G1-PS-GMA, (B) intra-particle kinetics model, (C) pseudo-first-order kinetics model and (D) pseudo-second-order kinetics model. | |
To investigate the mechanism of glyphosate adsorption onto the modified beads, the results were fitted with 3 kinetic models, including the intraparticle diffusion model (eqn (3)), pseudo-first-order model (eqn (4)) and the pseudo-second-order model (eqn (5)).
Intraparticle diffusion model:
Pseudo-first-order model:
| ln(qe − qt) = ln(qe) − K1t | (4) |
Pseudo-second-order model:
| 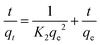 | (5) |
where
qt (mg g
−1) and
qe (mg g
−1) are the amount of glyphosate adsorbed at
t min and equilibrium, respectively.
Kp (mg (g min
0.5)
−1),
K1 (min
−1) and
K2 (g (mg min)
−1) are the rate constants of the intraparticle diffusion model, pseudo-first-order model and pseudo-second-order model, respectively. The results of linear fitting are shown in
Fig. 10 and the obtained parameters are listed in
Table 3.
Table 3 Adsorption kinetics model parameters
Experimental qe (mg g−1) |
Intraparticle diffusion |
Pseudo-first-order |
Pseudo-second-order |
K
p
|
C
|
R
2
|
K
1
|
q
e
|
R
2
|
K
2
|
q
e
|
R
2
|
8.85 |
1.705 |
1.614 |
0.512 |
0.575 |
9.141 |
0.725 |
0.058 |
9.515 |
0.988 |
The constant C in the intraparticle diffusion model indicates whether the adsorption is controlled by internal diffusion or not.35 If C is equal to 0, the adsorption procedure was solely governed by intraparticle diffusion. In contrast, the adsorption mechanism is complicated. According to the results in Table 3, the constant C is not zero, which implied that glyphosate adsorption is not only controlled by intraparticle diffusion.
Compared to the pseudo-first-order model, the linear plot of t/qtversus t in the pseudo-second-order model a showed higher correlation coefficient (R2 = 0.988). Therefore, the pseudo-second-order model is more suitable for fitting data, indicating that the adsorption rate is controlled by the chemical adsorption mechanism.36 In addition, adsorption amounts at equilibrium (qe) calculated by the pseudo-second-order model was also close to experimental data.
3.5 Adsorption isotherms
Adsorption isotherms are often used to describe the relationship between adsorption capacity and solution concentration. Generally, there are two commonly used adsorption isotherm models for adsorption equilibrium isotherm data research. The equations are expressed as follows:
Langmuir equation:
|  | (6) |
Freundlich equation:
|  | (7) |
where
qmax (mg g
−1) is the maximum adsorption capacity of the adsorbent,
b is the Langmuir adsorption equilibrium constant, and
KF and
n are the Freundlich constant related to adsorption and interaction between the adsorbate and adsorbent, respectively. Fitting curves of the two models are presented in
Fig. 11. And the fitted values of
qmax,
b,
KF, and 1/
n, and the correlation coefficients (
R2) are listed in
Table 4. According to the calculated correlation coefficients, the adsorption procedure better fitted the Langmuir model. Therefore, it can be considered that the adsorbents have uniform surface properties and glyphosate molecules undergo single-layer adsorption on the solid surface.
37 In the Langmuir equation, the maximum adsorption capacity of adsorbents (
qmax) decreased with higher temperature, which is consistent with the conclusion of the effect of temperature.
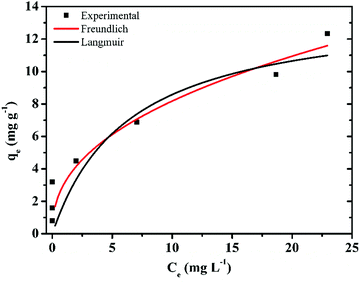 |
| Fig. 11 Isotherm model fitting for glyphosate adsorption on the G1-PS-GMA adsorbent at 25 °C. | |
Table 4 Langmuir and Freundlich model parameters
T (°C) |
Langmuir model |
Freundlich model |
q
max
|
b
|
R
2
|
K
F
|
1/n |
R
2
|
25 |
14.0404 |
0.1570 |
0.9667 |
3.1092 |
0.4200 |
0.9426 |
50 |
12.8065 |
0.1342 |
0.9746 |
2.0202 |
0.5241 |
0.9827 |
75 |
11.2552 |
0.1062 |
0.9509 |
1.6252 |
0.4814 |
0.9360 |
The adsorption process in the Langmuir model could be evaluated by the Langmuir dimensionless constant separation factor (RL). The value of RL indicates whether the type of isotherm is favorable (0 < RL < 1), linear (RL = 1) or unfavorable (RL > 1).16 The RL values in the Langmuir model were calculated using the following equation:
|  | (8) |
where
Ce is the equilibrium concentration of glyphosate (mg L
−1) and
b is the Langmuir adsorption equilibrium constant. The calculated values of
RL at different temperatures and concentrations are shown in
Table 5. With
RL values varying in the range of 0.181–1.000, adsorptions of glyphosate onto the adsorbents at different initial concentrations and temperatures are proved to be favorable.
Table 5
R
L values calculated at different temperatures and concentrations
Temperature C0 (mg L−1) |
25 °C |
50 °C |
75 °C |
R
L
|
5 |
1.000 |
0.866 |
0.897 |
10 |
1.000 |
0.879 |
0.868 |
20 |
1.000 |
0.815 |
0.851 |
30 |
0.762 |
0.676 |
0.560 |
50 |
0.457 |
0.444 |
0.412 |
80 |
0.255 |
0.273 |
0.259 |
100 |
0.217 |
0.224 |
0.181 |
3.6 Thermodynamic parameters
In consideration of the results at different temperatures, the glyphosate adsorption process is temperature dependent. To investigate the temperature effect on glyphosate adsorption, the standard Gibbs free energy change (ΔG) was calculated using the following equation: | ΔG = −RT ln KC | (9) |
where R is the universal gas constant (8.314 J mol−1 K−1) and T is the adsorption temperature (K). KC is thermodynamic equilibrium constant defined as eqn (10): |  | (10) |
According to the van’t Hoff equation, the average entropy change (ΔS) and standard enthalpy change (ΔH) can also be calculated:
| 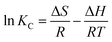 | (11) |
The result of thermodynamic parameters is shown in Table 6. ΔG in negative values implied that the adsorption procedure occurred spontaneously. The negative value of ΔH means that the glyphosate adsorption is an exothermic process, which is consistent with the adsorption results at different temperatures.
Table 6 Thermodynamic parameters
Temperature (°C) |
ΔG (kJ mol−1) |
ΔH (kJ mol−1) |
ΔS (J mol−1 K−1) |
25 |
−4.103 |
−13.88 |
−31.28 |
50 |
−3.344 |
75 |
−2.264 |
3.7 Regeneration and reproducibility
Considering the concept of green chemistry, desorption and regeneration of the adsorbents were investigated. Based on the result of the pH effect, 10–80 mmol L−1 sodium hydroxide solutions were used to release adsorbed glyphosate. The recycled adsorbents were washed to neutral and dried for 4 h at 60 °C under vacuum before being used for the second cycle. Residual glyphosate in each cycle was determined by ion chromatography. The peak areas in the first cycle and second cycle were defined as Acyc1 and Acyc2, respectively. According to the ratio (Acyc2/Acyc1) calculate in Fig. 12, adsorbed glyphosates were totally released in 50 mmol L−1 sodium hydroxide solution. Under the optimal conditions, adsorption and desorption processes were repeated for more than 5 cycles. According to a previous adsorption experiment, 85.9% of the glyphosate in the solution was removed by a new G1-PS-GMA adsorbent. After 5 adsorption/desorption cycles, the removal efficiency of glyphosate was 82.55%. Therefore, the extraction procedure of the synthesized adsorbents exhibited good reproducibility.
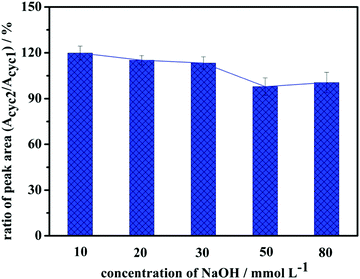 |
| Fig. 12 Residual glyphosate peak area ratio in cycle 2 to cycle 1. | |
3.8 Application in water samples
Since glyphosate existing in drinking water is most dangerous to humans and animals, water purification is of great significance. Under the optimized conditions, the synthesized adsorbents were used to purify 20 mg L−1 glyphosate standard solution and drinking water spiked with 20 mg L−1 of glyphosate. The adsorption efficiencies in standard solution and drinking water were 100% and 96.87%, respectively. As glyphosate was adsorbed by electrostatic interactions, efficiency decrease may result from competing ions in water solutions. Anions competed to bind protonated surface groups of the adsorbents, affecting the efficiency of glyphosate adsorption. Even though, abundant active sites can guarantee an adsorption efficiency of more than 95% in drinking water.
4. Conclusions
In this work, PAMAM dendrimer functionalized adsorbents based on polymer beads were synthesized and successfully used for the removal of glyphosate from water samples. The synthesized adsorbents appeared very effective for the maximum adsorption of glyphosate by electrostatic interactions at pH 3, with a minimum adsorption equilibrium time of 5 min. Lower temperature was proved to be favorable for the adsorption process. The capacities of the adsorbents could be easily controlled by varying generations of grafted PAMAM dendrimers. The adsorption kinetics and isotherm data fitted well with the pseudo-second-order model and Langmuir model, respectively. The adsorption of glyphosate on the adsorbents was determined to be a spontaneous process. Regeneration and reproducibility experiments showed that the adsorbents could be reused for at least 5 cycles. Adsorption efficiency in contaminated drinking water is more than 95%, which proved that adsorbents have potential applications for glyphosate removal in low salt solution purification.
Conflicts of interest
The authors declare no competing financial interest.
Acknowledgements
This work was performed under the financial support of the Zhejiang Provincial Natural Science Foundation of China (No. LZ16B050001, LY15B050001, and LY15B010005), Zhejiang provincial key subject of TCM (No. 2017-XK-A23) and Key Laboratory of Health Risk Appraisal for Trace Toxic Chemicals of Zhejiang Province.
References
- J. P. K. Gill, N. Sethi and A. Mohan, Environ. Chem. Lett., 2017, 15, 85–100 CrossRef CAS.
- A. H. C. v. Bruggen, M. M. He, K. Shin, V. Mai, K. C. Jeong, M. R. Finckh and J. G. Morris, Sci. Total Environ, 2018, 616–617, 255–268 CrossRef.
- R. O. McClellan, Crit. Rev. Toxicol., 2016, 46, 1–2 CrossRef PubMed.
- D. Cattani, V. L. de Liz Oliveira Cavalli, C. E. Heinz Rieg, J. T. Domingues, T. Dal-Cim, C. I. Tasca, F. R. Mena Barreto Silva and A. Zamoner, Toxicology, 2014, 320, 34–45 CrossRef CAS.
- R. Mesnage, N. Defarge, J. S. de Vendomois and G. E. Seralini, Food Chem. Toxicol., 2015, 84, 133–153 CrossRef CAS.
- X. K. Ding and K. L. Yang, Anal. Chem., 2013, 85, 5727–5733 CrossRef CAS PubMed.
- H. C. Lan, Z. F. Jiao, X. Zhao, W. J. He, A. M. Wang, H. J. Liu, R. P. Liu and J. H. Qu, Sep. Purif. Technol., 2013, 117, 30–34 CrossRef CAS.
- M. Manogaran, M. Y. Shukor, N. A. Yasid, K. A. Khalil and S. A. Ahmad, 3 Biotech, 2018, 8 DOI:10.1007/s13205-018-1123-4.
- G. M. Fu, R. Y. Li, K. M. Li, M. Hu, X. Q. Yuan, B. Li, F. X. Wang, C. M. Liu and Y. Wan, Prep. Biochem. Biotechnol., 2016, 46, 780–787 CrossRef CAS.
- J. N. Shen, J. Huang, H. M. Ruan, J. D. Wang and B. Van der Bruggen, Desalination, 2014, 342, 118–125 CrossRef CAS.
- J. Yuan, J. Duan, C. P. Saint and D. Mulcahy, Environ. Technol., 2018, 39, 1384–1392 CrossRef CAS PubMed.
- J. Qu, J. Environ. Sci., 2008, 20, 1–13 CrossRef CAS.
- M. M. Nourouzi, T. G. Chuah and T. S. Y. Choong, Desalin. Water Treat., 2010, 24, 321–326 CrossRef CAS.
- S. Fiorilli, L. Rivoira, G. Cali, M. Appendini, M. C. Bruzzoniti, M. Coisson and B. Onida, Appl. Surf. Sci., 2017, 411, 457–465 CrossRef CAS.
- D. Feng and Y. Xia, J. Sep. Sci., 2018, 41, 732–739 CrossRef CAS.
- N. U. Yamaguchi, R. Bergamasco and S. Hamoudi, Chem. Eng. J., 2016, 295, 391–402 CrossRef.
- S. Zavareh, Z. Farrokhzad and F. Darvishi, Ecotoxicol. Environ. Saf., 2018, 155, 1–8 CrossRef CAS.
- D. A. Tomalia, A. M. Naylor and W. A. Goddard, Angew. Chem., Int. Ed. Engl., 1990, 29, 138–175 CrossRef.
- D. A. Tomalia, H. Baker, J. Dewald, M. Hall, G. Kallos, S. Martin, J. Roeck, J. Ryder and P. Smith, Polym. J., 1985, 17, 117–132 CrossRef CAS.
- P. Kesharwani, A. Gothwal, A. K. Iyer, K. Jain, M. K. Chourasia and U. Gupta, Drug Discovery Today, 2018, 23, 300–314 CrossRef CAS.
- E. Murugan and I. Pakrudheen, Sci. Adv. Mater., 2015, 7, 891–901 CrossRef CAS.
- S. H. Wang, C. Y. Shen, Y. M. Lin and J. C. Du, Smart Mater. Struct., 2016, 25, 085018 CrossRef.
- Q. Cao, Y. C. Liu, C. Z. Wang and J. S. Cheng, J. Hazard. Mater., 2013, 263, 311–321 CrossRef CAS.
- Y. J. Jiang, Q. M. Gao, H. G. Yu, Y. R. Chen and F. Deng, Microporous Mesoporous Mater., 2007, 103, 316–324 CrossRef CAS.
- B. Fadhel, M. Hearn and A. Chaffee, Microporous Mesoporous Mater., 2009, 123, 140–149 CrossRef CAS.
- S. Y. Zhou, A. L. Xue, Y. Zhang, M. S. Li, K. Li, Y. J. Zhao and W. H. Xing, Appl. Clay Sci., 2015, 107, 220–229 CrossRef CAS.
- D. D. Guo, C. Y. Lou, Z. P. Huang, N. Muhammad, Q. M. Zhao, S. C. Wu and Y. Zhu, New J. Chem., 2018, 42, 8653–8660 RSC.
- M. T. Gokmen and F. E. Du Prez, Prog. Polym. Sci., 2012, 37, 365–405 CrossRef CAS.
- J. Plotka-Wasylka, M. Marc, N. Szczepanska and J. Namiesnik, Crit. Rev. Anal. Chem., 2017, 47, 373–383 CrossRef CAS.
- A. V. Zatirakha, A. D. Smolenkov and O. A. Shpigun, Anal. Chim. Acta, 2016, 904, 33–50 CrossRef CAS.
- D. D. Guo, C. Y. Lou, P. M. Zhang, J. J. Zhang, N. N. Wang, S. C. Wu and Y. Zhu, J. Chromatogr. A, 2016, 1456, 113–122 CrossRef CAS.
- D. D. Guo, C. Y. Lou, N. N. Wang, M. L. Chen, P. M. Zhang, S. C. Wu and Y. Zhu, Talanta, 2017, 168, 188–195 CrossRef CAS.
- S. J. Yuan, J. T. Gu, Y. Zheng, W. Jiang, B. Liang and S. O. Pehkonen, J. Mater. Chem. A, 2015, 3, 4620–4636 RSC.
- B. M. Lombardi, R. M. T. Sanchez, P. Eloy and M. Genet, Appl. Clay Sci., 2006, 33, 59–65 CrossRef CAS.
- L. Xiong, Y. Yang, J. X. Mai, W. L. Sun, C. Y. Zhang, D. P. Wei, Q. Chen and J. R. Ni, Chem. Eng. J., 2010, 156, 313–320 CrossRef CAS.
- L. Wang and A. Q. Wang, J. Hazard. Mater., 2008, 160, 173–180 CrossRef CAS.
- C. Y. Zhou, D. M. Jia, M. Liu, X. W. Liu and C. H. Li, J. Chem. Eng. Data, 2017, 62, 3585–3592 CrossRef CAS.
|
This journal is © The Royal Society of Chemistry and the Centre National de la Recherche Scientifique 2019 |