DOI:
10.1039/C7NJ03745G
(Paper)
New J. Chem., 2018,
42, 717-724
Synthesis of nanoporous cobalt/carbon materials by a carbonized zeolitic imidazolate framework-9 and adsorption of dyes†
Received
1st October 2017
, Accepted 27th November 2017
First published on 11th December 2017
Abstract
Herein, four nanoporous cobalt/carbon materials were synthesized by one-step calcination of the zeolitic imidazolate framework-9 (ZIF-9) at 600, 700, 800, and 900 °C. The adsorption performance of the obtained Z9-600, Z9-700, Z9-800, and Z9-900 towards methylene green (MeG) in an aqueous solution was investigated. The results indicate that Z9-600 demonstrates maximum adsorption as compared to other three materials. The adsorption capacity of Z9-600 for another eleven organic dyes (malachite green, rhodamine B, rhodamine 6G, crystal violet, methyl blue, methyl orange, acid red 18, acid orange 7, orange G, congo red, and rosaniline) was studied systematically. Valid analysis based on the data demonstrates that the pseudo-second-order model, the intra-particle diffusion model, and the Langmuir model are suitable for describing the adsorption process of Z9-600. The regeneration efficiency of Z9-600 for MeG is >93% after 4 cycles of reuse. These highly-effective, economical, and recyclable features demonstrate that nanoporous cobalt/carbon ZIFs are promising adsorbents for the treatment of wastewater containing dyes.
1. Introduction
As is known, organic dyes are serious water pollutants due to their carcinogenicity, teratogenicity, and difficult degradation;1 therefore, it is urgent and necessary to efficiently remove dyes from wastewater before they are released into the environment.2 Currently, for this purpose, several techniques including chemical treatment, biological degradation, and physical means have been explored. Among these approaches, adsorption is considered as one of the most simple, effective, and attractive methods.3
Many kinds of materials, such as porous silica, zeolites, activated carbon, and porous polymers, have been used as adsorbents.4 However, the adsorption capacities of these materials are limited. Thus, it is still essential to develop effective and high capacity adsorbents. Recently, a new type of material called zeolitic imidazolate frameworks (ZIFs) has been developed.5 Owing to the tunable porous network of ZIFs, several ZIFs such as ZIF-67, ZIF-8, and ZIF-9 have been proven to be promising materials for dye adsorption.6–8
ZIF-derived cobalt/carbon composites, derived from the calcination of ZIFs, have uniform microstructures as ZIF precursors as well as better thermal and chemical stabilities.9–12 In terms of various sizes and charges of dyes, nanoporous cobalt/carbon ZIFs have been convinced to be competent adsorbents.13 It has been proven that these ZIFs exhibit not only excellent adsorption performance to dyes but also a simple separation process.14 The separation process for the recovery of adsorbents only requires one additional magnet, therefore avoiding separation steps such as precipitation, filtration, and centrifugation.15 Several nanoporous cobalt/carbon materials (formed by calcining ZIF-67 at different temperatures) have been used for the adsorption of methyl blue, rhodamine B, and malachite green.6–8,16,17 However, there are no systematic researches.
ZIF-9 is constructed from Co2+ ions and the N-atoms from benzimidazole (bim) anions and possesses a hexagonal symmetry with sodalite topology.18 In this study, ZIF-9 was used as a precursor for the preparation of nanoporous cobalt/carbon ZIFs due to the inclusion of cobalt, high content of carbon, and good thermal stability of it. Z9-600, Z9-700, Z9-800, and Z9-900 were synthesized by one-step calcination of ZIF-9 at 600, 700, 800, and 900 °C (under a nitrogen flow), respectively. The structures and compositions of the synthesized materials were characterized by PXRD, Raman spectroscopy, SEM, and N2 adsorption. Remarkably, Z9-600, Z9-700, Z9-800, and Z9-900 exhibit high adsorption capacities to MeG. Furthermore, the adsorption performance of optimal Z9-600 to other eleven organic dyes was systematically studied. In addition, the kinetic model, regeneration, and reusability tests of Z9-600 to MeG were also performed.
2. Experimental
The chemicals used and physical characterization methods are provided in the ESI.† ZIF-9 was prepared according to previously reported procedures.18 The synthesis and characterization of ZIF-9 are also provided in the ESI† (see Fig. S1).
2.1 Carbonization of ZIF-9
The dry ZIF-9 powders were directly calcined in a horizontally tubular furnace under a nitrogen flow (ca. 45 mL min−1) at the required temperature for 2 h at a heating rate of 5 °C min−1. After cooling down to room temperature, the final products (black powders) were obtained and named Z9-600, Z9-700, Z9-800, and Z9-900, corresponding to the heating temperatures of 600, 700, 800, and 900 °C, respectively.
2.2 Dye adsorption
Herein, twelve organic dyes, i.e. cationic dyes: rhodamine 6G (R6G), rhodamine B (RHB), methylene green (MeG), malachite green (MaG), and crystal violet (CV), and anionic dyes: methyl blue (MB), methyl orange (MO), acid red 18 (AR), acid orange 7 (AO), orange G (OG), and congo red (CR), and neutral dye: rosaniline (RO), were studied for adsorption. Their molecular structures, weights, and calibrated UV-vis absorption wavelengths (λ) are given in Table S1 (ESI†).6,19–22 At first, 4.0 × 10−4 mol L−1 aqueous solutions of twelve dyes were prepared beforehand. During adsorption, dye solutions at various concentrations were obtained by the successive dilution of the abovementioned stock solutions. Typically, calcinated ZIF-9 materials (15 mg) were added to the dye solutions (10 mL); then, 3 mL supernatants were taken from the solutions at pre-determined time intervals and monitored by UV-vis absorption spectroscopy at the calibrated maximum wavelengths (λ = 565, 554, 656, 617, 583, 627, 464, 507, 484, 478, 495, and 543 nm, corresponding to abovementioned dyes in sequence).23
To assess the effect of the ionic strength of the solution, adsorption experiments were performed in MeG dye solutions with different concentrations of NaCl (from 0.1 to 0.5 mol L−1). The temperature effect of Z9-600 on MeG adsorption was systematically investigated at 20, 40, and 60 °C.
2.3 Desorption experiments
The regeneration of used Z9-600 saturated with MeG was conducted using the solvent desorption technique. The MeG-adsorbed Z9-600 was dispersed in the regeneration agent DMF.24 The desorption experiments were performed until the amount of desorbed MeG remained unchanged. The entire process was completed within 30 min.
2.4 Collection and recyclability experiments
Z9-600 was separated and obtained from the solutions using an external magnet because of the presence of magnetic cobalt.6 The desorbed Z9-600 was washed with pure water twice and then dried for re-use. The recyclability experiments were performed based on the dye adsorption methods.
3. Results and discussion
3.1 Structural characterization
As shown as Fig. 1 (left hand side), the PXRD patterns of Z9-600, Z9-700, Z9-800, and Z9-900 reveal the presence of metallic Co and graphitic carbon in the samples according to the JCPDS file No. 42-1467, and the characteristic peaks of four materials are in agreement with previous reports.25 The peak intensities of Co become gradually enhanced as the pyrolysis temperature increases; this indicates that the deposited Co nanoparticles are sintered to form large Co particles at high temperatures. As shown in Fig. 1 (right hand side), the five Raman peaks located at 192, 470, 510, 608, and 682 cm−1 obtained for the as-synthesized composites featured cobalt content.26 Moreover, two distinguishable bands assigned to D and G at 1350 and 1590 cm−1 represent disordered and graphitic carbon, respectively.27 The values of ID/IG for Z9-600, Z9-700, and Z9-800 composites exhibit an obvious increase of 0.92, 0.97, and 1.04, respectively. According to available reports, this is due to the formation of defective nanocrystalline graphite, which attributes to D band, with an increase in temperature. For Z9-900, the value of ID/IG decreases to 0.95 due to the removal of defects during its transformation from nanocrystalline graphite to graphite at 900 °C.8 A similar phenomenon has also been reported in a ZIF-8-based material when the pyrolysis temperature increases from 700 to 900 °C. The ID/IG ratio first increases and then decreases and reaches 0.88 at 900 °C.27 The ID/IG ratio of a ZIF-67-based material calcined at 900 °C is 0.80.28 The slightly higher ID/IG value of Z9-900 in this study may be attributed to the disordered graphitic carbon within the network.29
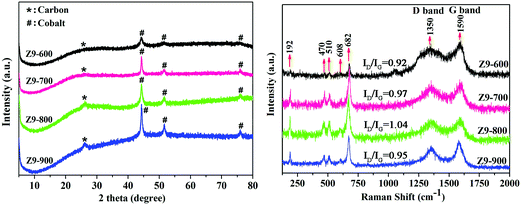 |
| Fig. 1 PXRD patterns (left) and Raman spectra (right) of Z9-600, Z9-700, Z9-800, and Z9-900. | |
After carbonization, Z9-600, Z9-700, Z9-800, and Z9-900 retain a similar shape as the ZIF-9 precursors (Fig. 2). However, the size of the materials decreases, and the surface becomes more concaved; this may be attributed to the internal decomposition of the carbon sources during carbonization.29
 |
| Fig. 2 SEM images of Z9-600, Z9-700, Z9-800, and Z9-900. | |
The nitrogen adsorption–desorption isotherms of the four composites showed pseudo-type I mixed with type IV isotherms (Fig. 3, left).30 The drastic increase at the beginning of the isotherms (indicative of high N2 uptake) and distinct hysteresis loops at the relative pressures of 0.5–1.0 illustrate that the materials are micro- and meso-porous. This was also confirmed by the pore size distribution (Fig. 3 right). Porosity properties of the four samples are shown in Table S2 (ESI†). The pore volumes and Brunauer–Emmett–Teller (BET) surface areas are in the order of Z9-600 > Z9-700 > Z9-800 > Z9-900, which may lead to different guest-molecule adsorptions.
 |
| Fig. 3 N2 adsorption–desorption isotherms at 77 K (left) and pore size distribution (right) of Z9-600, Z9-700, Z9-800, and Z9-900. | |
3.2 Adsorption experiments
The obtained four nanoporous cobalt/carbon materials were utilized as adsorbents for treating the MeG aqueous solution. Typically, a 15 mg sample of the ZIF was mixed with 10 mL of the MeG solution in a pre-determined concentration. After adsorption was allowed to occur over a set time, 3 mL of supernatant was taken from the solution and analyzed using a UV-visible spectrophotometer at λ = 656 nm and then put back in the solution each time. The adsorption capacity was calculated by the following equation:31where qt (mg g−1) is the adsorption capacity at time t (h), C0 and Ct (mg L−1) are the initial and time concentration of dyes, V (L) is the volume of the dye solution, and m (g) is the weight of the used adsorbent.
As shown in Fig. 4, for Z9-600, Z9-700, Z9-800, and Z9-900, the adsorption capacities increase rapidly at the beginning and then remain constant, with equilibrium times of 24, 30, 48, and 60 h and qt values of 57.5, 57.1, 56.7, and 55.9 mg g−1, respectively. It is obvious that all four materials express high adsorption capacities, with Z9-600 exhibiting the optimum performance in terms of adsorption speed and capacity. Of the four materials, Z9-600 has the largest specific surface area and pore volume, which may enable the diffusion of the dye molecules.23
 |
| Fig. 4 Time-dependent adsorption capacities of Z9-600, Z9-700, Z9-800, and Z9-900 towards MeG (2.0 × 10−4 mol L−1) at room temperature. | |
Thus, Z9-600 was chosen as a representative adsorbent, and its adsorption capabilities were tested towards a further eleven organic dyes. As shown in Fig. 5, the maximum adsorptions of MaG, CR, RO, AR, OG, CV, AO, MO, MB, RHB, and R6G of Z9-600 were 61.4, 47.0, 46.2, 42.5, 29.5, 26.8, 23.2, 21.3, 54.6, 29.5, and 26.8 mg g−1, respectively. It can be seen that Z9-600 exhibits good adsorption performance towards MaG, CR, RO, AR, and MB.
 |
| Fig. 5 Time-dependent adsorption capacities of Z9-600 towards eleven dyes. The initial concentration of MaG and RO were 2.0 × 10−4 mol L−1, and that of the other dyes was 1.0 × 10−4 mol L−1. | |
The adsorption capacity of the as-synthesized ZIF-9 towards MeG was also investigated. Because of its low density, ZIF-9 floated on the surface of the solution, and a negligible amount of MeG was adsorbed after 12 hours.
3.3 Removal efficiency
The removal efficiency of the twelve dyes by Z9-600 were estimated using31As shown in Fig. 6, the removal percentages of all twelve dyes are higher than 90%. In particular, MeG, MaG, AO, and CR are almost totally removed (reach or above 99%); this indicates special high removal efficiency of Z9-600 towards these four dyes.
 |
| Fig. 6 The removal efficiency of Z9-600 towards twelve dyes. The initial concentrations of MeG, MaG, and RO were 2.0 × 10−4 mol L−1, and that of the other dyes was 1.0 × 10−4 mol L−1. | |
3.4 Adsorption kinetics
To quantitatively analyze the adsorption kinetics of Z9-600, the effect of contact time on MeG adsorption with various initial MeG concentrations was investigated. As seen in Fig. 7 (left), the adsorption capacities first increased, then remained constant, with equilibrium times of 12 h for 0.5 × 10−4, 0.8 × 10−4, and 1.0 × 10−4 mol L−1 and 30 h for 2.0 × 10−4 and 2.5 × 10−4 mol L−1 solutions. The equilibrium absorption capacity increased from 14.1 to 71.6 mg g−1 with the increasing dye concentrations; this suggested that high MeG concentrations could cause favorable adsorption.
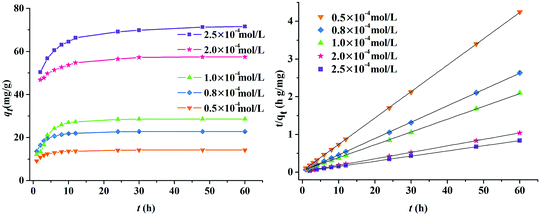 |
| Fig. 7 The effect of the initial MeG concentration on the adsorption ability by Z9-600 (left), and the pseudo-second-order kinetic plots for the adsorption of MeG (right). | |
The pseudo-second-order kinetic model could be used to fit the experiment data:32
where
qe (mg g
−1) is the adsorption capacity at equilibrium and
K (g mg
−1 h
−1) is the equilibrium rate constant. The values of
qe and
K were determined from the plots (
Fig. 7 right) and are summarized in Table S3 (ESI
†). The high correlation coefficients (>0.99) indicate that all the adsorption processes fit well with the pseudo-second-order kinetic model. According to the literature, the adsorption mechanism of Z9-600 should depend on both the adsorbate and adsorbent.
33 The pseudo-second-order kinetic model was also suitable for the adsorption processes of the other dyes (
Fig. 8). The adsorption capacities at equilibrium, rate constants, and correlation coefficients for the other dyes are listed in Table S3 (ESI
†).
 |
| Fig. 8 The pseudo-second-order kinetic plots for the other eleven dye adsorption by Z9-600. | |
Intraparticle diffusion refers to the movement of dye molecules from the bulk solution to the solid phase. The following intraparticle diffusion model was used to fit the adsorption kinetic data:34
where
Kid (
Ki1 and
Ki2) is the intraparticle diffusion rate constant (mg g
−1 h
−0.5), which can be found from the slope of
qtversus t0.5, and
Ci (
C1 and
C2) represents the intercept. The intraparticle diffusion kinetic models of Z9-600 for the series concentration of MeG and the other eleven dyes are shown in
Fig. 9 and 10. All adsorbents exhibited bilinearity, consisting of two stages. In these two stages, the values of
Ki1 are found to be much higher than those of
Ki2,
C1 is smaller than
C2, and the correlation coefficients
R12 are higher than
R22; this illustrates a fast adsorption stage followed by an equilibrium stage. The corresponding parameters are listed in Table S3 (ESI
†). The straight lines at the first stage do not pass through the origin; this indicates that the intraparticle diffusion is not a rate-determining step and the adsorption rate is controlled by intraparticle diffusion and liquid film diffusion simultaneously.
31
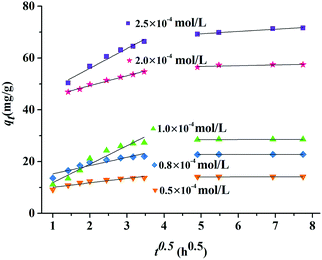 |
| Fig. 9 Intraparticle diffusion kinetic model by Z9-600 for a series concentrations of MeG. | |
 |
| Fig. 10 Intraparticle diffusion kinetic models for the rest of eleven dye adsorptions by Z9-600. | |
To further explore the adsorption process of the MeG by Z9-600, Langmuir and Freundlich models were adopted to analyze the experimental results.17,35 The fitting plots of two isotherm models are given in Fig. 11, and the calculated parameters are listed in Table S4 (ESI†).
 |
| Fig. 11 Fitting of Langmuir (left) and Freundlich (right) isothermal adsorption models of MeG by Z9-600. | |
The Langmuir isotherm is
| Ce/qe = 1/qmKL + Ce/qm, | (5) |
where
qm (mg g
−1) is the maximum adsorption capacity and
KL (L mg
−1) is the Langmuir constant. These can be calculated from the slope and the intercept of lines. The dimensionless separation factor
RL can be obtained by
RL value indicates the favorable adsorption (unfavorable > 1, linear = 1, favourable 0 <
RL < 1, and irreversible = 0).
33 The caculated
RL value was 0.9, confirming the favorable use of the Langmuir isotherm for the adsorption process of MeG.
The Freundlich equation is expressed as
| ln qe = ln KF + (ln Ce)/n | (7) |
where
KF (mg g
−1) is the Freundlich constant and 1/
n is the heterogeneity factor. The
KF and
n were calculated from the intercept and slope of the plots, respectively. By comparing the fitting results, it can be seen that the
RL2 of te Langmuir model is higher than the
RF2 of the Freundlich model. That is, the Langmuir model is more suitable for describing the adsorption of MeG by Z9-600. This illustrates that the surface of Z9-600 is homogenous and exerts monolayer adsorption.
16
3.5 Effects of ionic strength on the adsorption capacity
Salts are commonly found in wastewater, and the existence of salts can lead to the change of ionic strengths;24 thus, the effect of salt concentration on the adsorption of Z9-600 in a MeG aqueous solution was investigated. As can be seen from Fig. 12, the adsorption capacity and the equilibrium time vary less, even when the concentration of NaCl reaches to 0.5 mol L−1, which is almost 100 times that of C0 (the initial concentration of MeG). This indicates that a salt does not obviously hinder or facilitate the MeG adsorption by Z9-600, and the effect of ionic strength on the adsorption capacity can be ignored.
 |
| Fig. 12 The effect of ionic strength (0.1, 0.2, and 0.5 mol L−1 NaCl) on the adsorption of MeG by Z9-600. | |
3.6 Effect of adsorption temperature
The effect of temperature on the adsorption capacity of Z9-600 towards MeG was also analyzed. As shown in Fig. 13, the adsorption equilibrium times are 30, 24, and 8 h corresponding to the temperature of 20, 40, and 60 °C, respectively. When the temperature was higher, the time for reaching equilibrium was shorter. This could be ascribed to faster diffusion rates of MeG at higher temperatures.26
 |
| Fig. 13 The effect of temperature on MeG (C0 = 2.0 × 10−4 mol L−1) adsorption by Z9-600. | |
3.7 Regeneration and reusability of Z9-600
The desorption of the dye from the exhausted adsorbent and the regenerability and the reusability of the adsorbent are crucial parameters.36 In this study, the desorption experiment of exhausted Z9-600 was conducted using DMF as the regeneration agent. The entire process was accomplished within 30 mins until the amount of desorbed MeG was unchanged. The regenerated Z9-600 was separated and obtained from DMF using an external magnet, washed with pure water for 2 times, collected, and dried for re-use. As shown in Fig. 14, the adsorption capacity and removal efficiency of the regenerated Z9-600 were obtained. After 4 cycles, the adsorption efficiency decreases about 7%, but remains at a high level. This indicates that Z9-600 exhibits high efficiency in MeG adsorption and can be readily and easily regenerated.37 The PXRD patterns of the regenerated Z9-600 after 4 cycles revealed that the structure of Z9-600 was unchanged (Fig. S2, ESI†).
 |
| Fig. 14 The adsorption capacity and removal efficiency of the regenerated Z9-600 for the adsorption of MeG (C0 = 2.0 × 10−4 mol L−1). | |
3.8 Comparison with other adsorbents
To evaluate the dye adsorption capacity of Z9-600, a comparison of the maximum adsorption capacity (qe) and reusability with those of other adsorbents is shown in Table 1. The results indicate that the adsorption capacities of Z9-600 and ZIF-67-800 towards MeG, MaG, RHB, MB, MO, and CR are superior to those of other kinds of absorbents such as as-synthesized ZIFs, alginate/Fe3O4 composites, and carbon nanospheres.6,7,24,38,39 Another advantage is that nanoporous cobalt/carbon ZIFs can be reused with high regeneration efficiency. The adsorption performances of Z9-600 to MaG, RHB, and MB are lower than those of ZIF-67-800. This may be attributed to the larger pore volume and BET surface area of ZIF-67-800, which facilitate faster molecular diffusion of dyes.
Table 1 Comparison with other adsorbents
Dyes |
Type of adsorbent |
q
e (mg g−1) |
Reusability |
Ref. |
—: Not be provided in reference. Yes: reusable. No: not reusable. |
MeG |
ZIF-7 |
5.7 |
No |
38
|
ZIF-8 |
6.8 |
No |
7
|
ZIF-67 |
7.0 |
No |
7
|
Z9-600 |
71.6 |
Yes |
This work |
|
MaG |
Alginate/Fe3O4 composites |
50.0 |
Yes |
24
|
Z9-600 |
61.4 |
Yes |
This work |
ZIF-67-800 |
561.8 |
Yes |
6
|
|
RHB |
Carbon nanospheres |
2.0 |
No |
39
|
ZIF-67 |
13.6 |
No |
7
|
ZIF-8 |
21.4 |
No |
7
|
Z9-600 |
29.5 |
Yes |
This work |
ZIF-67-800 |
400.0 |
Yes |
6
|
|
MB |
ZIF-67 |
12.9 |
No |
7
|
ZIF-8 |
16.6 |
No |
7
|
Z9-600 |
54.6 |
Yes |
This work |
ZIF-67-800 |
502.0 |
Yes |
6
|
|
MO |
ZIF-67 |
16.3 |
No |
7
|
ZIF-8 |
9.2 |
No |
7
|
Z9-600 |
21.3 |
Yes |
This work |
|
CR |
ZIF-9 |
43.6 |
— |
17
|
Z9-600 |
47.0 |
Yes |
This work |
4. Conclusions
In this study, four nanoporous cobalt/carbon materials (Z9-600, Z9-700, Z9-800, and Z9-900) were prepared directly by the calcination of the zeolitic imidazolate framework-9 at different temperatures. Z9-600 exhibited an adsorption capability (towards the dye MeG) that was superior to those of Z9-700, Z9-800, and Z9-900. Z9-600 also shows good adsorption capability to MaG, CR, RO, AR, and MB. The adsorption behavior of Z9-600 towards all twelve dyes was found to fit the pseudo-second-order model and intraparticle diffusion kinetic model. In addition, Z9-600 could be separated, collected, and regenerated through simple steps. Consequently, nanoporous cobalt/carbon ZIFs are expected to be employed as efficient, economic, and recyclable adsorbents in the treatment of wastewater containing dyes. Moreover, further studies are currently under way in our group.
Conflicts of interest
There are no conflicts to declare.
Acknowledgements
This work was supported by the National Natural Science Foundation of China (Grant Numbers. 21471029 and 21601019).
References
- Y. Xu, J. J. Jin, X. L. Li, Y. D. Han, H. Meng, J. B. Wu and X. Zhang, J. Sep. Sci., 2016, 39, 3647 CrossRef CAS PubMed.
- V. Rizzi, F. D'Agostino, P. Fini, P. Semeraro and P. Cosma, Dyes Pigm., 2017, 140, 480 CrossRef CAS.
- R. Rakshit, E. Khatun, M. Pal, S. Talukdar, D. Mandal, P. Saha and K. Mandal, New J. Chem., 2017, 422, 944 Search PubMed.
- A. Mathew, S. Parambadath, M. J. Barnabas, H. J. Song, J. S. Kim, S. S. Park and C. S. Ha, Dyes Pigm., 2016, 131, 177 CrossRef CAS.
- D. Krishna, C. Jayachandrababu, D. S. Sholl and S. Nair, J. Am. Chem. Soc., 2017, 139, 5906 CrossRef PubMed.
- C. P. Ruan, K. L. Ai and L. H. Lu, Chin. J. Anal. Chem., 2016, 44, 224 CAS.
- Y. Li, K. Zhou, M. He and J. F. Yao, Microporous Mesoporous Mater., 2016, 234, 287 CrossRef CAS.
- R. Qiang, Y. C. Du, D. T. Chen, W. J. Ma, Y. Wang, P. Xu, J. Ma, H. T. Zhao and X. J. Han, J. Alloys Compd., 2016, 681, 384 CrossRef CAS.
- Y. V. Kaneti, S. Dutta, Md. S. A. Hossain, M. J. A. Shiddiky, K.-L. Tung, F.-K. Shieh, C.-K. Tsung, K. C.-W. Wu and Y. Yamauchi, Adv. Mater., 2017, 29, 1700213 CrossRef PubMed.
- R. R. Salunkhe, Y. V. Kaneti, J. Kim, J. H. Kim and Y. Yamauchi, Acc. Chem. Res., 2016, 49, 2796 CrossRef CAS PubMed.
- R. R. Salunkhe, J. Tang, Y. Kamachi, T. Nakato, J. H. Kim and Y. Yamauchi, ACS Nano, 2015, 9, 6288 CrossRef CAS PubMed.
- N. L. Torad, M. Hu, Y. Kamachi, K. Takai, M. Imura, M. Naito and Y. Yamauchi, Chem. Commun., 2013, 49, 2521 RSC.
- K.-Y. A. Lin and H. A. Chang, J. Taiwan Inst. Chem. Eng., 2016, 53, 40 CrossRef.
- Z. B. Wu, X. Z. Yuan, J. Zhang, H. Wang, L. B. Jiang and Z. M. Zeng, ChemCatChem, 2017, 9, 64 Search PubMed.
- N. L. Torad, M. Hu, S. Ishihara, H. Sukegawa, A. A. Belik, M. Imura, K. Ariga, Y. Sakka and T. Yamauchi, Small, 2014, 10, 2096 CrossRef CAS PubMed.
- S. Z. Xu, Y. L. Lv, X. F. Zeng and D. P. Cao, Chem. Eng. J., 2017, 323, 502 CrossRef CAS.
- J. Dai, S. Z. Xiao, J. Liu, J. He, J. D. Lei and L. Y. Wang, RSC Adv., 2017, 7, 6288 RSC.
- S. S. Yan, S. X. Ouyang, H. Xu, M. Zhao, X. L. Zhang and J. H. Ye, J. Mater. Chem. A, 2016, 4, 15126 CAS.
- L. Y. Wang, M. Q. Fang, J. Liu, J. He, L. H. Deng, J. D. Li and J. D. Lei, RSC Adv., 2015, 5, 50942 RSC.
- S. Yao, T. Xu, N. Zhao, L. R. Zhang, Q. S. Huo and Y. L. Liu, Dalton Trans., 2017, 46, 3332 RSC.
- J. S. Qin, S. R. Zhang, D. Y. Du, P. Shen, S. J. Bao, Y. Q. Lan and Z. M. Su, Chem. – Eur. J., 2014, 20, 5625 CrossRef CAS PubMed.
- L. A. Mercante, M. H. M. Facure, D. A. Locilento, R. C. Sanfelice, F. L. Migliorini, L. H. C. Mattoso and D. S. Correa, New J. Chem., 2017, 41, 9087 RSC.
- J. Kalmar, G. Lente and I. Fabian, Dyes Pigm., 2016, 127, 170 CrossRef.
- K.-Y. A. Lin and H. A. Chang, Chemosphere, 2015, 139, 624 CrossRef CAS PubMed.
- W. Chaikittisilp, N. L. Torad, C. L. Li, M. Imura, N. Suzuki, S. Ishihara, K. Ariga and Y. Yamauchi, Chem. – Eur. J., 2014, 20, 4217 CrossRef CAS PubMed.
- K.-Y. A. Lin and S. Y. Chen, ACS Sustainable Chem. Eng., 2015, 3, 3096 CrossRef CAS.
- W. J. Ma, Y. C. Du, N. Wang and P. Miao, Environ. Sci. Pollut. Res., 2017, 24, 16276 CrossRef CAS PubMed.
- X. Ma, X. Zhao, J. S. Huang, L. T. Sun, Q. Li and X. R. Yang, ACS Appl. Mater. Interfaces, 2017, 9, 21747 CAS.
- H. Y. Jing, X. D. Song, S. Z. Ren, Y. T. Shi, Y. L. An, Y. Yang, M. Q. Feng, S. B. Ma and C. Hao, Electrochim. Acta, 2016, 213, 252 CrossRef CAS.
- F. Hao, L. Li, X. H. Zhang and J. H. Chen, Mater. Res. Bull., 2015, 66, 88 CrossRef CAS.
- Y. Cheng, Q. C. Feng, M. Yin, X. Y. Ren, J. Z. Wang and Y. H. Zhou, New J. Chem., 2016, 40, 9125 RSC.
- W. J. Guo, N. Sun, Y. K. Du, L. Y. Wang and M. S. Pei, New J. Chem., 2017, 41, 3352 RSC.
- S. F. Azha, M. Shahadat and S. Ismail, Dyes Pigm., 2017, 145, 550 CrossRef CAS.
- H. Zhang, F. Y. Zhang and Q. Huang, RSC Adv., 2017, 7, 5790 RSC.
- T. Yao, S. Guo, C. F. Zeng, C. Q. Wang and L. X. Zhang, J. Hazard. Mater., 2015, 292, 90 CrossRef CAS PubMed.
- G. B. Hong and Y. K. Wang, Appl. Surf. Sci., 2017, 423, 800 CrossRef CAS.
- X. P. Luo, S. Y. Fu, Y. M. Du, J. Z. Guo and B. Li, Microporous Mesoporous Mater., 2017, 237, 268 CrossRef CAS.
- W. J. Ma, Q. Jiang, P. Yu, L. F. Yang and L. Q. Mao, Anal. Chem., 2013, 85, 7550 CrossRef CAS PubMed.
- J. Qu, Q. Zhang, Y. Xia, Q. Cong and C. Luo, Environ. Sci. Pollut. Res., 2015, 22, 1408 CrossRef CAS PubMed.
Footnote |
† Electronic supplementary information (ESI) available. See DOI: 10.1039/c7nj03745g |
|
This journal is © The Royal Society of Chemistry and the Centre National de la Recherche Scientifique 2018 |
Click here to see how this site uses Cookies. View our privacy policy here.