DOI:
10.1039/C8FO01981A
(Paper)
Food Funct., 2019,
10, 355-365
Development of anti-photo and anti-thermal high internal phase emulsions stabilized by biomass lignin as a nutraceutical delivery system†
Received
10th October 2018
, Accepted 10th December 2018
First published on 14th December 2018
Abstract
Herein, oil-in-water high internal phase emulsions (HIPEs) were stabilized by lignin from different technical resources with the assistance of alkyl polyglycoside (APG) using an ultrasonic cavitation method. The results showed that the amphiphilicity of lignin determined the formation of HIPEs. Microscopic and rheological characterization indicated that the molecular weight and concentration of lignin, the APG dosage and the oil/water ratio had important influences on the microstructure and stability of the HIPEs. In addition, nutraceutical β-carotene could be encapsulated in the oil phase of the HIPEs, which exhibited outstanding protection against photo- and thermal-oxidative degradation of β-carotene. After storage for 30 days under room temperature/light or storage for 7 days under 55 °C/dark conditions, the retention of β-carotene in the HIPEs was 87% to 95%, which is significantly higher than that of β-carotene dispersed in bulk oil. In vitro digestion experiments indicated that the digestibility of the HIPEs also markedly improved. The largest release of free fatty acids was 80%. The bio-accessibility of β-carotene in these lignin-based HIPEs also reached 60%.
Introduction
Biopolymer-stabilized emulsions serve as nutraceutical carriers for functional food and dietary supplements.1,2 These emulsions have been widely studied because of the increasing consumer demand for “label-friendly” ingredients that are natural, sustainable, and ethical and improve bioavailability and palatability.3 In particular, high internal phase emulsions (HIPEs), in which the volume fraction of the internal phase (Φ) exceeds 0.74, are attracting increasing attention in recent years for their applications in food, pharmaceuticals and cosmetics.4–6 As a nutraceutical carrier, the advantages of HIPEs include remarkably high Φ and tunable viscoelasticity properties. HIPE formulations with high nutraceutical loading and specific rheological behaviors are desirable for flexible downstream applications.7 Meanwhile, the shelf life of oil-in-water (O/W) HIPEs is longer than those of other emulsion systems. This is because HIPEs have very low water content, which prevents the growth of microorganisms.8 Additionally, Tan et al. reported that HIPEs can provide improved stability as well as controlled release in the gastrointestinal tract for encapsulated nutraceuticals.9
Lignin is the second most abundant biopolymer in nature; it represents up to 30% of the world's organic biomass.10–12 As the only scalable and renewable feedstock consisting of aromatic monomers, lignin has broad-spectrum UV-absorbance and excellent antioxidant, biocompatible, environmentally friendly, and nontoxic properties.13,14 Therefore, lignin has been extensively investigated and applied in natural antioxidants, macromolecular sunscreens, bio-sorbents, biosensors, drug nanocarriers, healthcare nanofibers, and biological scaffolds.15–21 In addition, lignin has good amphiphilicity and dispersity and can be a suitable substitute for natural surfactants for stabilization of emulsions.22,23 Tortora et al. reported that lignin can form a stable microemulsion at a water/oil interface by ultrasound-assisted cross-linking.19 However, the evaluation of HIPEs stabilized with lignin is rare. Lignin cannot stabilize high amounts of oil until it forms particles under strong acid conditions. Furthermore, the effects of the lignin source and structure on the stability of HIPEs have not been investigated.14
β-Carotene is the carotenoid with the highest pre-vitamin A activity; it is widely present in many yellow and orange fruits and vegetables as well as some animal products.24 Additionally, β-carotene has good antioxidant activity; it protects cells from the damaging effects of free radicals and prevents some chronic diseases, such as cancer, heart disease, and aging.25,26 In addition, β-carotene can improve immune system response and reproductive system function.27 Meanwhile, β-carotene is in great demand in food and pharmacy-related industries. The largest market for an individual carotenoid is for β-carotene (US $261 million in 2010, projected to grow to $334 million by 2018, compound annual growth rate 3.1%).28 However, β-carotene utilization as a nutraceutical ingredient in the food industry is limited by its poor water solubility, high melting point, low bioavailability and chemical instability.29 The chemical instability of β-carotene is caused by the photo- and thermal-oxidative degradation of its polyisoprenoid structure of long-conjugated chains of carbon–carbon double bonds.30 The literature reports that emulsion delivery systems are often a better choice for loading bioactive lipids such as β-carotene into functional foods because the emulsion increases the bioavailability of β-carotene and decelerates its degradation.31 For example, Rao et al.32 found that the bio-accessibility of β-carotene was 76% in corn oil-in-water emulsions, which is about three times that in raw carrot.33 Liang et al.1 and Liu et al.31 have proved that emulsion systems are effective at protecting β-carotene under different environmental conditions, including temperature and light. Meanwhile, researchers have reported that oils containing unsaturated long-chain fatty acids, such as corn and soybean oil, can form micelles, which can improve the solubility and bio-accessibility of β-carotene in oils.34
In a previous study, we found that lignin is a potential alternative to expensive antioxidants such as quercetin.35 KoŠikova et al. also proved that lignin products display great potential as antioxidants in the human diet and in polymer blends.15 Therefore, the main objectives of this work were to use lignin from different technical resources as an emulsifier to prepare HIPEs with the assistance of eco-friendly, safe and biodegradable alkyl polyglucoside (APG) using an ultrasonic cavitation method and to investigate the effects of the emulsifier concentration, lignin structure and oil phase volume fraction on the microstructure, stability and rheological properties of O/W HIPEs. Subsequently, the ability of the HIPEs to protect bioactive β-carotene against photo- and thermal-oxidation degradation was evaluated. In addition, an in vitro digestion experiment was performed to mimic the digestion processes in gastric fluid and intestinal fluid to determine the bio-accessibility of β-carotene after emulsification.
Materials and methods
Materials
Enzymatic hydrolysis lignin (EHL) extracted from corncobs, alkali lignin (AL) extracted from bamboo and organosolv lignin (OL) extracted from pines were supplied by Shenquan Biotechnology Co. Ltd (Shandong, China), Huanlong New Material Co., Ltd (Sichuan, China) and Chemical Point UG (Deisenhofen, Germany), respectively. Sulfonated lignin (SAL) extracted from pine, calcium lignosulfonate (CaLS), and sodium lignosulfonate (NaLS) were purchased from Tongdong sheng hua Chemical Co. Ltd (Hunan, China), Xinjiang Tianhong paper industry Co., Ltd (Xinjiang, China), and Chempack Co. Ltd (Russia), respectively. The lignin samples were carefully purified by alkaline dissolution, acidification, filtration, and washing at least three times. Soybean oil (Mw = 290 g mol−1) was purchased from a local supermarket (Guangzhou, China). 50% APG (0810) solution, pancreatin and β-carotene were purchased from Sigma–Aldrich (Shanghai, China). Pepsin extracted from porcine gastric mucosa and bile salt were obtained from Aladdin (Shanghai, China). All other chemicals were purchased from Guanghua Sci-Tech Co. Ltd (Guangdong, China) and were used without further purification. Water used in all experiments was purified by deionization.
Physicochemical parameters of lignin
The molecular weight of lignin was characterized by gel permeation chromatography (Waters 1515, USA) using Ultrahydragel 120 and 250 columns with 0.10 mol L−1 of sodium nitrate as the mobile phase at a flow rate of 0.50 mL min−1. The concentration of the lignin solution was 3 g L−1. The phenolic hydroxyl and carboxy groups and the sulfonation degree of lignin were measured using the aqueous potentiometric titration method (905-Titrando, Metrohm, Switzerland) at different pH values.36 The titrating solutions were 0.5 M HCl aqueous solution and 9.6 mmol L−1 NaOH aqueous solution, respectively. The water contact angles of the lignin samples were characterized using a JC2000C1 contact angle-measuring instrument (Powereach, China). 20 wt% lignin aqueous solution was uniformly dispersed on glass slides and air-dried at room temperature. The results are shown in Table S1.†
Preparation of lignin solution and β-carotene oil phase
Briefly, lignin solutions were prepared by adding weighted lignin powders (EHL, AL and OL) to a fixed amount of 1 M NaOH aqueous solution. Subsequently, the solution was centrifuged to remove any impurities after being stirred overnight. Lignosulfonate solutions were prepared by adding weighted lignosulfonate powders (SAL, NaLS and CaLS) to a fixed amount of distilled water. Then, the solution was centrifuged to remove impurities after stirring overnight. The concentrations of the EHL, AL, OL, SAL, NaLS and CaLS solutions, measured by an MX-50 Super Hybrid Sensor (AND Co. Ltd, Japan), were 17.29 ± 0.04 wt%, 19.73 ± 0.86 wt%, 18.82 ± 0.56 wt%, 14.94 ± 0.46 wt%, 17.12 ± 0.37 wt%, and 14.46 ± 0.23 wt%, respectively. The β-carotene oil phase was prepared by dispersing β-carotene in soybean oil. After stirring in the dark for 6 hours, a homogeneous dispersion with a concentration of 0.5 mg mL−1 was produced.
Preparation of the HIPEs
The preparation of HIPEs was performed by varying five key parameters, including molecular weight and concentration of lignin and lignosulfonate, APG dosage, and O/W ratio. The HIPEs were prepared using a Scientz-IID ultrasonic cell disruption system (Ningbo Scientz biological Co. Ltd, China) with output pulsing of 2 s on and 3 s off and 40% amplitude for 5 min and an Ultra Turrax T25 homogenizer (IKA, Germany) operating at 24
000 rpm for 5 min. Experimental factors and levels for the preparation of the lignin-based HIPEs are summarized in Table S2.†
Characterization of the HIPEs
The microstructures of the HIPEs droplets dispersed in water (20 mg mL−1) were observed using optical microscopy (XPV-800E, Shanghai Bi Mu Instrument Co., Ltd China). The average diameter and size distribution of the droplets were obtained through image analysis using Nano Measurer 1.2 software.37 Storage stability was determined by storing the HIPE samples at room temperature. All the droplet sizes were measured after 7 days and 30 days and compared with those of fresh samples.
Rheology of the HIPEs
The rheological behavior of HIPEs can provide information on the stabilization mechanism, preparation conditions and properties of emulsions. Therefore, the rheological properties of the HIPEs were studied using a RV-I rheometer (Haake, Germany) with a cone and plate geometry (60 mm diameter, 1° cone angle, and 0.05 mm gap).
Shear dependence.
The shear rate was varied from 0.01 to 600 s−1. The obtained flow curves are presented in terms of viscosity and shear stress.
Amplitude dependence.
The percentage-strain was varied from 0.01 to 100% at a frequency of 1 Hz. This oscillatory test provides information about the storage and loss moduli (G′ and G′′, respectively) in the linear viscoelastic (LVE) region.
Frequency dependence.
The angular frequency was varied from 1 to 600 rad s−1 in the linear viscoelastic range. The complex viscosity (|η*|), incorporating the elastic and viscous responses, was calculated using the following equation.37 |  | (1) |
where η′ is the dynamic viscosity, η′′ is the elastic (imaginary) part of the complex viscosity, G′ is the elastic (storage) modulus, G′′ is the viscous (loss) modulus and ω is the angular frequency.
Dispersion of β-carotene in HIPEs and stability study
For the fabrication of β-carotene-encapsulated HIPEs (HIPEs-BC), HIPEs-BC were prepared by processing the oil phase (80 vol%) and water phase (1.0 wt%, 3.0 wt%, 5.0 wt% of lignin and 3.5 wt% of APG) the ultrasonic disruption system for 5 min. Subsequently, the HIPEs-BC and β-carotene/soybean oil dispersions were divided into three groups, which were stored at (1) 25 °C under light for 7 days, (2) room temperature under light for 30 days and (3) 55 °C in the dark for 7 days. At certain time intervals, 0.15 g of the HIPEs-BC was removed and extracted with a certain volume of a mixture of ethanol and hexane (volume ratio 2
:
3). After being shaken well, the hexane phase was collected, followed by absorbance measurement at 450 nm by a UV-Vis spectrophotometer (UV-2550, Shimadzu, Japan).9
In vitro digestion of HIPEs-BC
To determine the free fatty acids (FFAs) released from HIPEs-BC, a two-stage simulated in vitro digestion method with simulated gastric fluid (SGF) and intestinal fluid (STF) was used with minor modifications of a previously described method.1,9,28 Firstly, 100 mL of SGF was freshly prepared by adding 0.2 g of NaCl and 0.32 g of pepsin; then, the pH was adjusted to 1.2 using 1.0 M HCl. To initiate gastric digestion, 0.3 g of HIPEs-BC (or bulk oil) as the internal phase was first mixed with 30 mL of 10 M phosphate buffer and incubated at 37 °C while being magnetically stirred for 2 min. Then, 25 mL of SGF was added; the pH of the mixture was adjusted to 2.0 with 1.0 M HCl and the mixture was incubated at 37 °C for 1 h with continuous stirring at 100 rpm in a constant-temperature incubator (DDHZ-300, China). An automatic titration device (905 Titrando, Metrohm, China) was used to simulate the conditions in the small intestinal phase. After the digestion in simulated SGF, the samples were immediately adjusted to pH 7.5 with NaOH solution (0.25 M). Next, 8 g of a bile salt solution [375 mg of bile salts dissolved in 10 mM phosphate buffer (pH 7.0)] and 5 g of a lipase suspension [60 mg of lipase powder dispersed in 10 mM phosphate buffer (pH 7.0)] were added. During the 2 h lipolysis, the pH was kept constant (7.5) by dropwise addition of 0.25 M NaOH to neutralize the free fatty acids released from the lipid digestion. The temperature was maintained at 37 °C by a thermostatic water bath with stirring. The percentage of FFAs released from the system was determined by the following equation:9 |  | (2) |
where mlipid represents the total mass of oil present in the sample during digestion (grams), Mw, lipid is the average molecular weight of the lipids (290 g mol−1), CNaOH is the concentration of NaOH (M), and VNaOH(t) is the volume of NaOH (L) used in the intestinal digestion at time t to neutralize any FFAs produced from triacylglycerols.
Bio-accessibility of β-carotene in HIPEs
After in vitro digestion, the dispersion was centrifuged at 10
000 rpm for 20 min β-carotene was extracted from the aqueous fraction containing formulated β-carotene micelles using 10 mL n-hexane. The concentration of β-carotene was determined by the same method described above. The bio-accessibility (%) of β-carotene was calculated using the following equation:9 |  | (3) |
Results and discussion
Formation of lignin-based HIPEs
Stable HIPEs could be prepared using EHL and a small dosage of APG as an emulsifier under basic conditions. As shown in Fig. 1a, HIPE was successfully stabilized by 5.0 wt% EHL and 3.5 wt% APG using an ultrasonic cavitation method. Soybean oil was used as the internal phase, and its volume content was 80 vol%. APG alone cannot stabilize this high amount of oil, as shown in Fig. 1b. The reason is that lignin can form cross-linking structures under ultrasonic cavitation processing.19 On the other hand, cross-linked lignin may associate with APG by hydrogen bonding and hydrophobic interactions because both species contain polyhydroxyl structures and hydrophobic skeletons/chains. In contrast, the HIPEs prepared with a homogenizer were less stable, as shown in Fig. S1.†
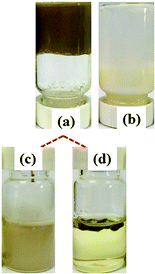 |
| Fig. 1 HIPEs stabilized by EHL and APG: (a) 5.0 wt% EHL, 3.5 wt% APG and 80 vol% oil phases; (b) 3.5 wt% APG and 80 vol% oil phases. (c) HIPEs dispersed in water; (d) HIPEs dispersed in oil. | |
This demonstrated the important role of high-intensity ultrasonic processing. As shown in Fig. 1c and d, the HIPEs could be evenly distributed in water but had no dispersibility in oil, which demonstrates that the HIPEs sample was a type of O/W emulsion.
Effects of the lignin species and concentration
The first set of HIPEs was obtained by keeping the APG dosage and oil phase constant while varying the species and concentration of lignin. The appearances, morphologies and droplet sizes of the HIPEs with lignin contents varying from 1.0 wt% to 10 wt% are shown in Fig. 2 and 3. When EHL and AL were used as emulsifiers, HIPEs could be formed by adding a small amount of APG. As the lignin content was varied from 1.0 wt% to 5.0 wt%, the morphology and the size distribution of the HIPEs showed no significant changes. When the lignin concentration was greater than 8.0 wt%, the droplets tended to be larger, and the size distribution became wider. Further increasing the lignin concentration led to an obvious decrement of the viscosity of the HIPEs, which began to appear to flow. These results indicate that APG was not sufficient to synergize high amounts of lignin to stabilize the oil phase. Interestingly, the droplet size of the HIPEs prepared with AL was smaller than that of the HIPEs stabilized with EHL at low content (≤ 5.0 wt%) and larger when the content was beyond 8.0 wt%. As shown in Table S1,† the molecular weight of EHL is larger than that of AL; AL has low steric hindrance and is more conducive to adsorption on the oil surface, forming a stable and close film. When the lignin concentration was above 8.0 wt%, the intermolecular cross-linking of EHL increased, forming a three-dimensional network structure and restraining the coalescence of the droplets. However, over-hydrophobic and too-hydrophilic lignin could not emulsify this high amount of oil. As shown in Fig. S2 and Table S1,† the emulsifying properties of OL with a contact angle of 52° and lignosulfonates, including SAL, NaLS and CaLS, with contact angles of 16 to 24°, were significantly poorer than that of EHL, with a contact angle of 45°, and AL, with a contact angle of 40°. Therefore, OL, SAL, NaLS and CaLS could not stabilize the HIPEs. The reason is that OL has lower amounts of phenolic hydroxyl and carboxyl groups and lignosulfonates contain sulfonic groups, which results in poor emulsifying ability.
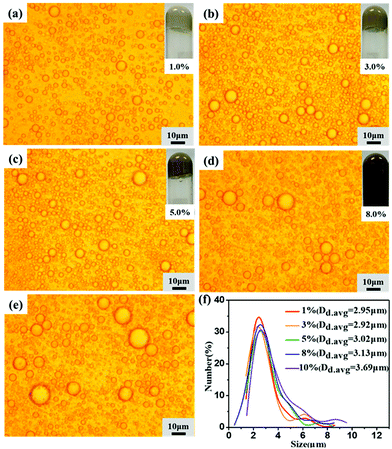 |
| Fig. 2 (a–e) Optical microscope images and (f) droplet sizes of the HIPEs prepared using 1, 3, 5, 8, and 10 wt% of EHL with 3.5 wt% APG and 80 vol% oil as the internal phase. | |
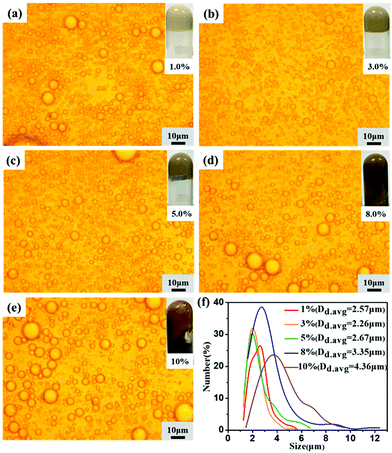 |
| Fig. 3 (a–e) Optical microscope images and (f) droplet sizes of the HIPEs prepared using 1, 3, 5, 8, and 10 wt% of AL with 3.5 wt% APG and 80 vol% oil. | |
Effects of APG dosage
The second set of HIPEs was obtained by keeping the lignin concentration and oil phase volume fraction constant while varying the APG dosage from 3.0 wt% to 3.5 wt%. The appearance, morphologies and droplet sizes of the HIPEs with different APG dosages are shown in Fig. 4 and S3.† As the APG dosage increased from 3.0 wt% to 3.5 wt%, the droplet size of the HIPEs prepared with EHL decreased from 4.45 to 2.92 μm, and that of the HIPEs stabilized with AL decreased from 3.35 to 2.26 μm. The size distributions of the HIPEs are close to log-normal. At the same APG dosage, the droplet diameter of the HIPEs prepared with AL was smaller than that of the HIPEs stabilized with EHL. Also, OL could not synergize with a low amount of APG to stabilize the oil phase, as shown in Fig. S4.†
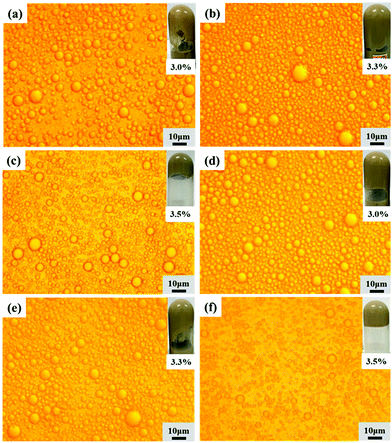 |
| Fig. 4 Photographs and optical microscope images showing the appearance of the HIPEs prepared using 3.0, 3.3, and 3.5 wt% APG dosages. In all the samples, the EHL (a, b, c) or AL (d, e, f) content is 3.0 wt% and the oil phase fraction is 80 vol%. | |
Effects of the oil phase volume fraction
A third set of HIPEs was fabricated to investigate the effects of the O/W ratio by varying the oil phase fraction from 75 vol% to 85 vol%.
The appearances, morphologies and droplet sizes of the HIPEs are shown in Fig. 5 and S5.† When the oil phase was below 80 vol%, the droplet sizes of the HIPEs with larger oil phase fractions were smaller. The reason is that the intervening film of oil between the droplets of the HIPEs with larger internal phase fractions was thinner and the droplet deformation was higher, resulting in smaller average sizes.37 However, when the oil phase volume fraction reached 82%, the system could not form HIPEs, which indicates that 3.0 wt% lignin (such as EHL or AL) and 3.5 wt% APG were not able to emulsify such a high oil content, as shown in Fig. S5.† As shown in Fig S6 and S2a,† OL has poorer emulsifying properties due to its strong hydrophobicity; the same amount (3 wt% OL + 3.5 wt% APG) could stabilize HIPEs with oil fractions ≤78%.
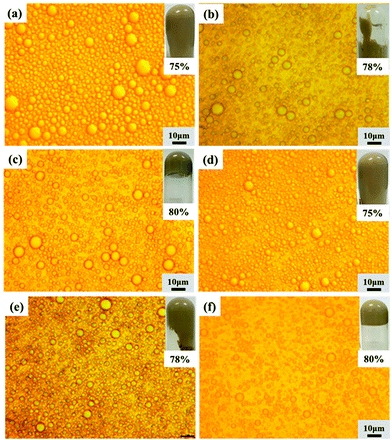 |
| Fig. 5 Photographs and optical microscope images showing the appearance of the HIPEs prepared with 75 vol%, 78 vol% and 80 vol% oil phase volumes. In all these samples, the EHL (a, b, c) or AL (d, e, f) content is 3.0 wt% and the APG dosage is 3.5 wt%. | |
Rheology of the HIPEs
The rheological properties of HIPEs have a close relationship with their stability.37 Therefore, the rheological behaviors of the HIPEs prepared with lignin/APG were analyzed.
Flow curves: shear sweep of the HIPEs.
The deformation behavior of the HIPEs with respect to strain rate represented by the viscosity is shown in Fig. 6. The viscosity of the HIPEs decreased as the shear rate increased, and they exhibited typical shear thinning behavior. As shown in Fig. 6a and b, when the lignin content was varied from 1.0 to 5.0 wt%, the viscosity of the HIPEs showed minor fluctuations. At the same content, the viscosity of the HIPEs prepared with AL was higher than that of the HIPEs stabilized with EHL. When the lignin content was above 8.0 wt%, the viscosity of the HIPEs considerably decreased. Interestingly, the viscosities of the HIPEs prepared with AL are lower than those of the HIPEs stabilized with EHL.
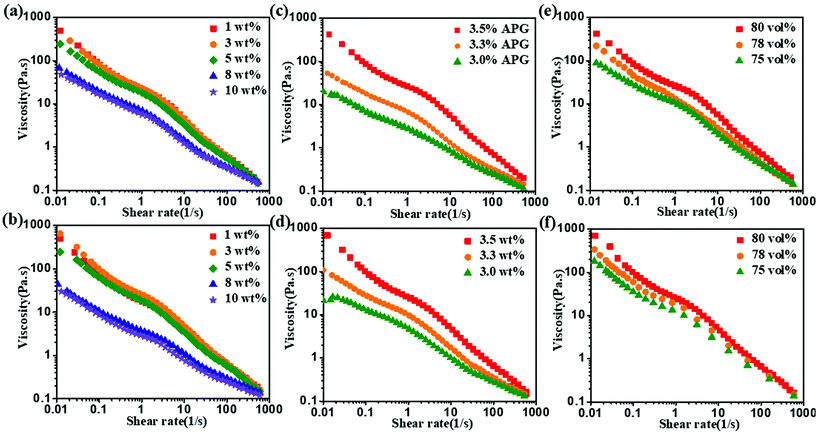 |
| Fig. 6 Flow curves of the HIPEs prepared with (a) different EHL contents and (b) different AL contents, respectively; (c) different APG dosages and 3.0 wt% EHL; (d) different APG dosages and 3.0 wt% AL; (e) different oil phase volume fractions and 3.0 wt% EHL; (f) different oil phase volume fractions and 3.0 wt% AL. | |
The influence of APG dosage on the viscosity of the HIPEs was also investigated, while the concentration of lignin was controlled at 3.0 wt%. As shown in Fig. 6c and d, the viscosity of the HIPEs increases obviously when the dosage of APG is varied from 3.0 wt% to 3.5 wt%. Meanwhile, the viscosity of the HIPEs prepared with AL is greater than the viscosity of the HIPEs stabilized with EHL. This is in accordance with reported results that a decrease in the average droplet diameter significantly improves the properties of HIPEs, such as the viscosity, yield stress and storage modulus; this is because thinner intervening liquid layers between the droplets result in tighter packing of the droplets and higher effective viscosity.40,41
For a given lignin and APG concentration, the HIPEs with smaller droplets showed higher viscosity when the oil content was below 80 vol%, as shown in Fig. 6e, f and S7a.† These data again demonstrate that thinner intervening liquid layers between the droplets result in tighter packages, lower flow abilities and higher effective viscosities.37
Oscillatory tests: amplitude sweep testing.
Amplitude sweeps provide information such as the critical strain during maximum deformation that a system can tolerate without permanent structural damage. The critical strain is a criterion for structural strength and shape retention under mechanical deformation. The results of the amplitude sweep tests performed on the HIPEs in terms of dynamic storage and loss moduli (G′ and G′′) variation with shear strain are shown in Fig. S8 and S7b.† It can be clearly seen in Fig. S8 and S7b† that all the HIPEs revealed typical gel-like behaviour, in which G′ was greater than G′′.
The specific values of the critical strain (γc), damping factor (tan(δ)), and complex viscosity (|η*|) obtained in the linear viscoelastic region (LVR) are presented in Table S3.† The γc value of the HIPEs remains constant as the lignin concentration increases from 1.0 wt% to 5.0 wt%. However, when the lignin concentration is above 8.0 wt%, the γc value of the HIPEs rapidly decreases. These results indicate that emulsions with smaller droplet sizes had thinner intervening liquid layers between the droplets, which decreased the flow ability of the emulsion and resulted in higher stability.37 Meanwhile, the γc value of the HIPEs with AL is higher when the lignin content is below 5.0 wt%. When the lignin content is greater than 8.0 wt%, the γc value of the HIPEs with EHL is higher.
For the different oil phase volume fractions, the γc value of the HIPEs increases as the oil phase varies from 75 vol% to 80 vol%. The higher fraction of the dispersed phase results in tighter droplet packing, implying that the HIPEs require more energy (including a larger γc value) to flow. Further, as the dosage of APG varies from 3.0 wt% to 3.5 wt%, the γc value of the HIPEs increases, which is similiar to the changes in the viscosities of the samples.
|η*| and tan(δ) are important parameters for investigating the storage stability of a dispersion. Larger |η*| values and lower tan(δ) values represent better consistency and elastic behavior for emulsions in the linear viscoelastic region.38 Taking all this into account, the HIPEs stabilized with 3.0 wt% EHL and AL, 3.5 wt% APG, and 80 vol% oil have better stability and elastic behavior, which is in accordance with the above results.
Small amplitude oscillatory tests: frequency sweep testing.
Frequency sweep testing gives information about the stability of a material over the measured frequencies at a constant amplitude. Therefore, frequency sweep tests of the HIPEs prepared with lignin/APG were conducted. As shown in Fig. 7a and b, as the lignin concentration varies from 1.0 wt% to 5.0 wt%, the G′ of the emulsion only shows a small fluctuation in a certain range. When the lignin concentration is greater than 8.0 wt%, the G′ of the emulsion decreases significantly. This suggests that the droplets are strongly associated with each other due to their smaller average diameter, which results in improved stiffness and droplet elasticity. Meanwhile, the HIPEs stabilized with AL have a higher G′ value than those stabilized with EHL when the lignin concentration is below 5.0 wt%. When the lignin concentration is above 8.0 wt%, the HIPEs with EHL have a higher G′ value. Additionally, as the APG dosage increases from 3.0 wt% to 3.5 wt%, the G′ value of the HIPEs with 80 vol% oil content increases, as shown in Fig. 7c and d. The influence of the O/W ratio on the G′ value was also investigated. As shown in Fig. 7e, f and S7c,† the HIPEs with a higher oil fraction exhibit a larger G′ value. These results again indicate that tighter overall droplet packing is propitious to increase the viscoelasticity and stability of the HIPEs.
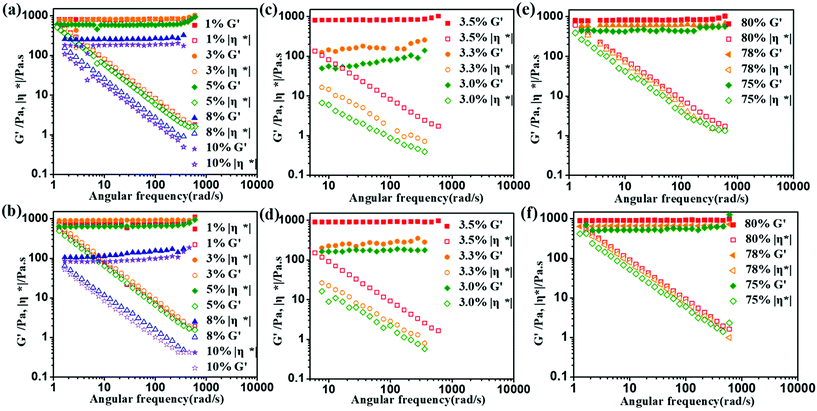 |
| Fig. 7 Frequency sweep results showing the behaviors of the storage modulus (G′) and complex viscosity (|η*|): (a) different EHL contents; (b) different AL contents; (c) different APG dosages and 3.0 wt% EHL; (d) different APG dosages and 3.0 wt% AL; (e) different oil phase volume fractions and 3.0 wt% EHL; (f) different oil phase volume fractions and 3.0 wt% AL. | |
Complex viscosity (|η*|) arises from a combination of viscous and elastic resistances; it represents the total resistance to flow of the HIPEs. As shown in Fig. 7 and S7c,† the complex viscosity of the HIPEs decreases as the angular frequency increases. The reason is that a higher frequency is primarily governed by increased dissipation of energy within the HIPEs, thereby decreasing the resistance to flow.
The morphology, size distribution and rheological analysis results imply that the amphiphilicity and concentration of lignin, the APG dosage and the O/W ratios are the main factors that influence the stability of the HIPEs. Firstly, the hydrophilic lignosulfonates, including SAL, NaLS and CaLS, and the more hydrophobic OL were difficult to adsorb on the oil surface, which resulted in de-emulsification/destabilization of the HIPEs. Secondly, when the concentration of lignin, including the EHL and AL concentrations, was below 5.0 wt%, AL with a lower molecular weight had low steric hindrance and was more conducive to adsorption on the oil surface, forming a stable and close film. This prevented coalescence of the droplets and maintained a smaller droplet size, resulting in improved viscoelasticity and stability of the HIPEs. When the lignin concentration was greater than 8.0 wt%, the intermolecular cross-linking of EHL with a larger molecular weight increased, forming a three-dimensional network structure and restraining the coalescence and flow of the droplets; this resulted in increased viscoelasticity and stability of the HIPEs system. In addition, when the APG dosage was varied from 3.0 wt% to 3.5 wt% or the oil phase was varied from 75 vol% to 80 vol%, the layer thickness of the continuous phase decreased, forming a thicker emulsion that is more elastic and can store more interfacial energy per unit volume.
Storage, photostabilities and thermal stabilities of the HIPEs
The storage stability of the HIPEs was studied at room temperature. As shown in Fig. S9 and S10,† the droplet sizes and flow abilities of the HIPEs prepared with 1.0 wt% and 3.0 wt% lignin were almost unchanged after one month. However, with prolonged preservation time, the droplet size of the HIPEs prepared with 5.0 wt% lignin increased and the viscosity obviously decreased.
Hou et al.39 reported that β-carotene is a highly polyunsaturated nutraceutical that is very susceptible to photo- and thermal-oxidative degradation during emulsification, processing, and storage. Lignin is a natural polyphenolic antioxidant which can scavenge free radicals and protect substrates from photo-oxidation and thermal oxidation.42,43 In order to study whether lignin-based HIPEs encapsulation can provide protective effects against photo- and thermal-oxidative degradation of β-carotene, the residual β-carotene content in the HIPEs during thermal and light treatment was determined and compared with those in other research studies. As shown in Fig. 8a, the percentage of remaining β-carotene in soybean oil was 94% after being stored at 25 °C/light for 7 days. The retention of β-carotene in soybean oil was 56% after 7 days of storage at 55 °C/in the dark. In the work of Liang et al., β-carotene was totally lost in medium-chain triacylglycerol (MCT) after being stored for 7 days at 25 °C in light.1 Liu et al. also reported that during storage at 55 °C/in the dark, the percentage of β-carotene remaining in medium chain triacylglycerol-in-water emulsions was only 48% after 7 days.31 These results show that the type of oil has an important influence on the oxidative degradation of β-carotene.34 The thermal oxidative degradation of β-carotene in lignin-based HIPEs was studied during storage at 55 °C/in the dark. As shown in Fig. 8b, as the lignin concentration increased from 1.0 wt% to 5.0 wt%, the β-carotene retention of the HIPEs prepared with EHL varied from 73% to 95% and that of the HIPEs stabilized with AL varied from 70% to 87%. In contrast, the residual ratio of β-carotene in bulk oil was only 56%. Interestingly, the retention of β-carotene in the HIPEs stabilized by EHL is higher than that of the HIPEs prepared with AL at the same concentration, although the content of phenolic hydroxyl groups in AL is greater than that in EHL (see Table S1†). It is proposed that EHL with a larger molecular weight can readily form larger three-dimensional net structures by ultrasonication-induced cross-linking; droplets of HIPEs-BC were uniformly dispersed in this network and, thus, the thermal resistance of β-carotene increased. The photo-stability of β-carotene in HIPEs was also determined over a period of 1 month under conditions of room temperature/light. As shown in Fig. 8c, as the lignin concentration increased from 1.0 wt% to 5.0 wt%, the final retentions of β-carotene were 91% to 95% for the EHL-stabilized HIPEs and 76% to 88% for the AL-stabilized HIPEs, respectively. The retention of β-carotene was only 58% in soybean oil. In the work of Tan et al., only 8% β-carotene was left in the sunflower oil after storage for 30 days at room temperature.9
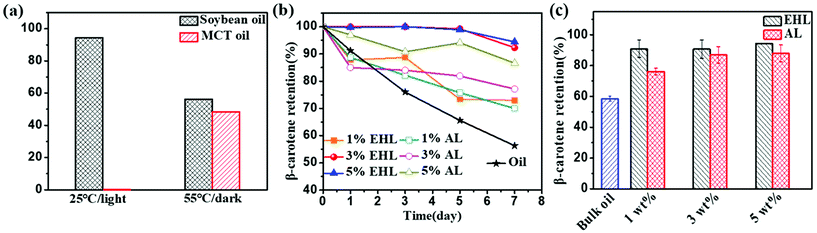 |
| Fig. 8 (a) Effects of temperature and light on the stability of β-carotene dispersed in soybean oil and MCT for 7 days. (b) The influence of lignin concentration on the degradation of β-carotene in HIPEs after storage for 7 days at 55 °C/in the dark. (c) The retention of β-carotene in HIPEs after storage for 30 days at room temperature/in light. | |
These results further indicate that lignin-based HIPEs show good anti-photo and anti-thermal oxidative degradation properties for β-carotene.
In vitro digestion of HIPEs-BC
The potential use of lignin-based HIPEs-BC as a nutraceutical delivery system was evaluated by mimicking the digestion process. In this process, the effects of lignin concentration and structure on the lipid digestion of HIPEs-BC were observed. As shown in Fig. 9a, the release profiles of FFAs in different lignin-based HIPEs-BC exhibited similar trends. At the initial stage, FFAs were rapidly released from HIPEs-BC. Subsequently, with prolonged digestion time, the release rate of the FFAs gradually decreased. When the lignin concentration was varied from 1.0 wt% to 5.0 wt%, the release levels of FFAs in the HIPEs stabilized with AL were 40% to 80% and those in the EHL-based HIPEs were 30% to 50%, respectively. In contrast, the released ratio of FFAs in the bulk soybean oil was only 18%. The reason is that the oil droplets in HIPEs have smaller sizes and larger surface areas, resulting in increased interactions with lipases and promoting the digestion of the HIPEs-BC.1 Therefore, the HIPEs-BC samples with smaller droplet sizes have higher FFA percentages. In addition, the digestion efficiency of HIPEs-BC stabilized with AL was greater than that of the HIPEs stabilized with EHL. It is possible that EHL, with a larger molecular weight, altered the efficiency of droplet disruption and coalescence in the stomach and small intestine, which led to a decrease of lipolysis.28
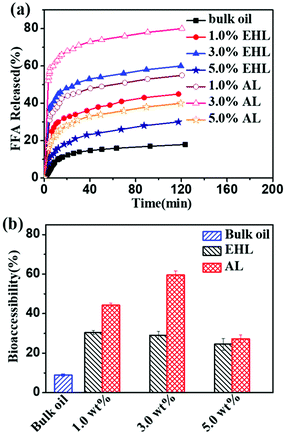 |
| Fig. 9 (a) FFA release profiles from β-carotene-encapsulated HIPEs and bulk oil as a function of small intestine digestion time. (b) Bio-accessibility of β-carotene in HIPEs and bulk oil after in vitro tests. For all samples, the oil phase is 80 vol% and the APG dosage is 3.5 wt%. | |
At the end of the digestion process, the bio-accessibility of β-carotene in the HIPEs or bulk oil was also investigated. As shown in Fig. 9b, the bio-accessibility of β-carotene in bulk oil is only 8.9%. The bio-accessibility of β-carotene in the HIPEs prepared with EHL decreases in the order of 1.0 wt% (31%) > 3.0 wt% (30%) > 5.0 wt% (20%). Furthermore, the bio-accessibility of β-carotene in the HIPEs stabilized with 3.0 wt% AL reached 60%. The bio-accessibility of β-carotene in the HIPEs stabilized with AL was higher than that in the HIPEs stabilized with EHL or bulk oil. It has been reported that the bio-accessibility of β-carotene is positively correlated with the micellization process during lipolysis.9,44 The HIPEs prepared with AL have smaller sizes and larger surface areas, which is beneficial for oil lipolysis and promotes the micellization process of β-carotene.
Conclusions
Stable O/W HIPEs have been produced using industrial lignin, including EHL and AL, and APG as stabilizers using an ultrasonic cavitation method. Hydrophilic lignosulfonates such as SAL, NaLS and CaLS and comparatively hydrophobic OL could not form stable emulsions. The rheological studies showed that the viscosity, yield stress, storage moduli, and complex viscosity of HIPEs have close relationships with the droplet size and oil fraction. The HIPEs with smaller droplet sizes or larger oil phase fractions had thinner intervening liquid layers and tighter packing of droplets. This decreased the flow ability of the emulsions and increased the interface stability. Lignin-based HIPEs were successfully applied as carriers for nutraceutical β-carotene. The retentions of β-carotene in EHL or AL stabilized HIPEs were 87% to 95% after storage for 7 days at 55 °C/in the dark or 30 days under room temperature/light conditions, while the retention in bulk oil was only 56% to 58%. The lignin-based HIPEs exhibited good protection against the photo- and thermal-oxidative degradation of β-carotene. In addition, the digestibility and digestion rate of the HIPEs were obviously higher than those of the bulk oil. The bio-accessibility of β-carotene in the HIPEs reached 60%. In summary, this work will broaden the knowledge of HIPEs in the development of new strategies to improve the storage stability and bio-accessibility of bioactive nutraceuticals in the food industry.
Conflicts of interest
The authors declare that there are no conflicts of interest.
Acknowledgements
This work was financially supported by the National Natural Science Foundation of China (NSFC) (21606089 and 21878113), Fundamental Research Funds for the Central Universities (2018JQ05), State Key Laboratory of Pulp and Paper Engineering (201701), Guangdong Province Science and Technology Research Project of China (2017B090903003), and Guangzhou Science and Technology Research Project of China (201704030126 and 201806010139).
References
- R. Liang, C. F. Shoemaker, X. Yang, F. Zhong and Q. Huang, Stability and bioaccessibility of beta-carotene in nanoemulsions stabilized by modified starches, J. Agric. Food Chem., 2013, 61, 1249–1257 CrossRef CAS PubMed.
- W. A. Fahmi Wan Mohamad, D. McNaughton, R. Buckowc and M. A. Augustin, Stability and partitioning of β-carotene in whey protein emulsions during storage, Food Funct., 2017, 8, 3917 RSC.
- H. Chen, D. J. McClements, E. Chen, S. Liu, B. Li and Y. Li, In Situ Interfacial Conjugation of Chitosan with Cinnamaldehyde during Homogenization Improves the Formation and Stability of Chitosan-Stabilized Emulsions, Langmuir, 2017, 33, 14608–14617 CrossRef CAS.
- W. Wijaya, P. Van der Meeren, C. H. Wijaya and A. R. Patel, High internal phase emulsions stabilized solely by whey protein isolate-low methoxyl pectin complexes: effect of pH and polymer concentration, Food Funct., 2017, 8, 584–594 RSC.
- T. Zeng, Z. L. Wu, J. Y. Zhu, S. W. Yin, C. H. Tang, L. Y. Wu and X. Q. Yang, Development of antioxidant Pickering high internal phase emulsions (HIPEs) stabilized by protein/polysaccharide hybrid particles as potential alternative for PHOs, Food Chem., 2017, 231, 122–130 CrossRef CAS PubMed.
- Y. Q. Hu, S. W. Yin, J. H. Zhu, J. R. Qi, J. Guo, L. Y. Wu, C. H. Tang and X. Q. Yang, Fabrication and characterization of novel Pickering emulsions and Pickering high internal emulsions stabilized by gliadin colloidal particles, Food Hydrocolloids, 2016, 61, 300–310 CrossRef CAS.
- H. Tan, G. Sun, W. Lin, C. Mu and T. Ngai, Gelatin Particle-Stabilized High Internal Phase Emulsions as Nutraceutical Containers, ACS Appl. Mater. Interfaces, 2014, 6, 13977–13984 CrossRef CAS PubMed.
-
A. F. Bot, Emulsion Gels in Food. In Product Design and Engineering: Formulation of Gels and Pastes, ed. U. Bröckel, W. Meier and G. Wagner, Wiley-VCH Verlag GmbH & Co. KGaA, Weinheim, Germany, 2013 Search PubMed.
- H. Tan, L. Zhao, S. Tian, H. Wen, X. Gou and T. Ngai, Gelatin particle-stabilized high-internal phase emulsions for use in oral delivery systems: protection effect and in vitro digestion study, J. Agric. Food Chem., 2017, 65, 900–907 CrossRef CAS PubMed.
- J. Ragauskas, G. T. Beckham, M. J. Biddy, R. Chandra, F. Chen, M. F. Davis, B. H. Davison, R. A. Dixon, P. Gilna, M. Keller, P. Langan, A. K. Naskar, J. N. Saddler, T. J. Tschaplinski, G. A. Tuskan and C. E. Wyman, Lignin valorization: improving lignin processing in the biorefinery, Science, 2014, 344, 1246843 CrossRef.
- D. Kai, M. J. Tan, P. L. Chee, Y. K. Chua, Y. L. Yap and X. J. Loh, Towards lignin-based functional materials in a sustainable world, Green Chem., 2016, 18, 1175–1200 RSC.
- L. H. Chen, J. Z. Dou, Q. L. Ma, N. Li, R. C. Wu, H. Y. Bian, D. J. Yelle, T. Vuorinen, S. Y. Fu, X. J. Pan and J. Y. Zhu, Rapid and near-complete dissolution of wood lignin at ≤ 80 °C by a recyclable acid hydrotrope, Sci. Adv., 2017, 3, 1701735 CrossRef.
- Y. Qian, X. W. Zhong, Y. Li and X. Q. Qiu, Fabrication of uniform lignin colloidal spheres for developing natural broad-spectrum sunscreens with high sun protection factor, Ind. Crop. Prod., 2017, 101, 54–60 CrossRef CAS.
- Y. Yang, Z. Wei, C. Y. Wang and Z. Tong, Lignin-based Pickering HIPEs for macroporous foams and their enhanced adsorption of copper(II) ions, Chem. Commun., 2013, 49, 7144–7146 RSC.
- B. KoŠikova, J. Lábaj, A. Gregorová and D. Slameňová, Lignin antioxidants for preventing oxidation damage of DNA and for stabilizing polymeric composites, Holzforschung, 2006, 60, 166–170 Search PubMed.
- Y. Qian, X. Q. Qiu and S. Zhu, Lignin: a nature-inspired sun blocker for broad-spectrum sunscreens, Green Chem., 2015, 17, 320–324 RSC.
- M. Wawrzkiewicz, P. Bartczak and T. Jesionowski, Enhanced removal of hazardous dye form aqueous solutions and real textile wastewater using bifunctional chitin/lignin biosorbent, Int. J. Biol. Macromol., 2017, 99, 754–764 CrossRef CAS PubMed.
- A. Jędrzak, T. Rębiś, Ł. Klapiszewski, J. Zdarta, G. Milczarek and T. Jesionowski, Carbon paste electrode based on functional GOx/silica-lignin system to prepare an amperometric glucose biosensor, Sens. Actuators, B, 2018, 256, 176–185 CrossRef.
- M. Tortora, F. Cavalieri, P. Mosesso, F. Ciaffardini, F. Melone and C. Crestini, Ultrasound driven assembly of lignin into microcapsules for storage and delivery of hydrophobic molecules, Biomacromolecules, 2014, 15, 1634–1643 CrossRef CAS PubMed.
- D. Kai, K. Zhang, L. Jiang, H. Z. Wong, Z. Li, Z. Zhang and X. J. Loh, Sustainable and Antioxidant Lignin–Polyester Copolymers and Nanofibers for Potential Healthcare Applications, ACS Sustainable Chem. Eng., 2017, 5, 6016–6025 CrossRef CAS.
- S. Quraishi, M. Martins, A. A. Barros, P. Gurikov, S. P. Raman, I. Smirnova, A. R. C. Duarte and R. L. Reis, Novel non-cytotoxic alginate–lignin hybrid aerogels as scaffolds for tissue engineering, J. Supercrit. Fluids, 2015, 105, 1–8 CrossRef CAS.
- C. Cai, X. Zhan, M. Zeng, H. M. Lou, Y. Pang, J. Yang, D. J. Yang and X. Q. Qiu, Using recyclable pH-responsive lignin amphoteric surfactant to enhance the enzymatic hydrolysis of lignocelluloses, Green Chem., 2017, 19, 5479–5487 RSC.
- S. Li, J. A. Willoughby and O. J. Rojas, Oil-in-Water Emulsions Stabilized by Carboxymethylated Lignins: Properties and Energy Prospects, ChemSusChem, 2016, 9, 2460–2469 CrossRef CAS PubMed.
- Y. Yuan, Y. Gao, L. Mao and J. Zhao, Optimisation of conditions for the preparation of β-carotene nanoemulsions using response surface methodology, Food Chem., 2008, 107, 1300–1306 CrossRef CAS.
- E. Paz, A. Martin, A. Estrella, S. Rodriguez-Rojo, A. A. Matias, C. M. M. Duarte and M. J. Cocero, Formulation of β-carotene by precipitation from pressurized ethyl acetate-on-water emulsions for application as natural colorant, Food Hydrocolloids, 2012, 26, 17–27 CrossRef.
- P. Wang, H. J. Liu, X. Y. Mei, M. Nakajima and L. J. Yin, Preliminary study into the factors modulating β-carotene micelle formation in dispersions using an in vitro digestion model, Food Hydrocolloids, 2012, 26, 427–433 CrossRef CAS.
- G. J. Handelman, The evolving role of carotenoids in human biochemistry, Nutrition, 2001, 17, 818–822 CrossRef CAS.
- Y. Fan, J. Yi, Y. Zhang and L. Zhao, Physicochemical stability and in vitro bioaccessibility of β-carotene nanoemulsions stabilized with whey protein-dextran conjugates, Food Hydrocolloids, 2017, 63, 256–264 CrossRef CAS.
- E. Meroni and V. Raikos, Physicochemical stability, antioxidant properties and bioaccessibility of β-carotene in orange oil-inwater beverage emulsions: influence of carrier oil types, Food Funct., 2018, 9, 320 RSC.
- M. J. Seliga, B. Mehrada, H. Zamania, A. Kierulfa, J. Lickerb and A. Abbaspourrad, Distribution of oil solubilized β-carotene in stabilized locust bean gum powders for the delivery of orange colorant to food products, Food Hydrocolloids, 2018, 84, 34–37 CrossRef.
- L. Liu, Y. Gao, D. J. McClements and E. A. Decker, Role of continuous phase protein, (-)-epigallocatechin-3-gallate and carrier oil on beta-carotene degradation in oil-in-water emulsions, Food Chem., 2016, 210, 242–248 CrossRef CAS.
- J. J. Rao, E. A. Decker and H. Xiao, Nutraceutical nanoemulsions: Influence of carrier oil composition (digestible versus indigestible oil) on β-carotene bioavailability, J. Sci. Food Agric., 2013, 93, 3175–3183 CrossRef CAS.
- S. Veda, A. Kamath, K. Platel, K. Begum and K. Srinivasan, Determination of bioaccessibility of b-carotene in vegetables by vitro methods, Mol. Nutr. Food Res., 2006, 50, 1047–1052 CrossRef CAS PubMed.
- C. Qian, E. A. Decker, H. Xiao and D. J. McClements, Nanoemulsion delivery systems: Influence of carrier oil on β-carotene bioaccessibility, Food Chem., 2012, 135, 1440–1447 CrossRef CAS PubMed.
- D. Liu, Y. Li, Y. Qian, Y. Xiao, S. Du and X. Q. Qiu, Synergistic Antioxidant Performance of Lignin and Quercetin Mixtures, ACS Sustainable Chem. Eng., 2017, 5, 8424–8428 CrossRef CAS.
- M. S. Zhou, K. Huang, X. Q. Qiu and D. J. Yang, Content determination of phenolic hydroxyl and carboxy in lignin by aqueous potentiometric titration method, J. Chem. Ind. Eng., 2012, 63, 258–265 CAS.
- S. Tripathi, A. Bhattacharya, R. Singh and R. F. Tabor, Rheological behavior of high internal phase water-in-oil emulsions: Effects of droplet size, phase mass fractions, salt concentration and aging, Chem. Eng. Sci., 2017, 174, 290–301 CrossRef CAS.
- K. Chen, G. Yu, F. He, Q. Zhou, D. Xiao, J. Li and Y. Feng, A pH-responsive emulsion stabilized by alginate-grafted anisotropic silica and its application in the controlled release of lambda-cyhalothrin, Carbohydr. Polym., 2017, 176, 203–213 CrossRef CAS PubMed.
- Z. Hou, Y. Gao, F. Yuan, Y. Liu, C. Li and D. Xu, Investigation into the physicochemical stability and rheological Properties of β-Carotene emulsion stabilized by soybean soluble polysaccharides and chitosan, J. Agric. Food Chem., 2010, 58, 8604–8611 CrossRef CAS PubMed.
- A. Y. Malkin and V. G. Kulichikhin, Structure and rheology of highly concentrated emulsions: a modern look, Russ. Chem. Rev., 2015, 84, 803 CrossRef CAS.
- R. Foudazi, S. Qavi, I. Masalova and A. Y. Malkin, Physical chemistry of highly concentrated emulsions, Adv. Colloid Interface Sci., 2015, 220, 78–91 CrossRef CAS PubMed.
- X. J. Pan, J. F. Kadla, K. Ehara, N. Gilkes and J. N. Saddler, Organosolv Ethanol Lignin from Hybrid Poplar as a Radical Scavenger: Relationship between Lignin Structure, Extraction Conditions, and Antioxidant Activity, J. Agric. Food Chem., 2006, 54, 5806–5813 CrossRef CAS PubMed.
- Z. L. Li, J. B. Zhang, L. Qin and Y. Y. Ge, Enhancing antioxidant performance of lignin by enzymatic treatment with laccase, ACS Sustainable Chem. Eng., 2018, 6, 2591–2595 CrossRef CAS.
- D. J. McClements and Y. Li, Review of in vitro digestion models forrapid screening of emulsion-based systems, Food Funct., 2010, 1, 32–59 RSC.
Footnote |
† Electronic supplementary information (ESI) available: Physicochemical parameters of lignin from different technical resources. Viscoelastic parameters for HIPEs prepared by different types and concentrations of lignin, APG dosages and oil phase fractions. Appearances of the HIPEs prepared using different types and concentrations of lignin at 3.5 wt% APG/80 vol% oil phase. The droplet sizes and size distributions of HIPEs prepared using different APG dosages at 3.0 wt% lignin/80 vol% oil phase. Appearances of HIPEs prepared using different APG dosages at 3.0 wt% OL/80 vol% oil phase. Appearances and droplet sizes of the HIPEs prepared by different oil phase fractions at 3.0 wt% AL, EHL, OL and 3.5 wt% APG. Rheological spectra of HIPEs prepared with different oil phases at 3.0 wt% OL and 3.5 wt% APG. Optical microscope images of HIPEs stabilized by different types and concentrations of lignin at 3.5 wt% APG/80 vol% oil after stored for 7 days and 30 days. See DOI: 10.1039/c8fo01981a |
|
This journal is © The Royal Society of Chemistry 2019 |