DOI:
10.1039/C8FO01902A
(Paper)
Food Funct., 2019,
10, 259-265
Ferulic acid regulates muscle fiber type formation through the Sirt1/AMPK signaling pathway
Received
28th September 2018
, Accepted 28th November 2018
First published on 29th November 2018
Abstract
Ferulic acid (FA) is a polyphenolic compound of plant cell walls. Several recent studies demonstrated that polyphenolic compounds could regulate muscle fiber type formation. In this study, we investigated the effect of FA on muscle fiber type formation and also explored its molecular mechanism in mouse C2C12 myotubes. Real-time quantitative PCR showed that FA significantly increased the mRNA abundances of myosin heavy chain (MyHC) I, MyHC IIa, sirtuin1 (Sirt1), peroxisome proliferator-activated receptor gamma coactivator-1-α (PGC-1α) and myocyte enhancer factor 2C (MEF2C), but decreased the mRNA abundance of MyHC IIb. FA increased succinate dehydrogenase (SDH) activity and decreased lactate dehydrogenase (LDH) activity. Western blot analysis showed that FA significantly increased the protein level of slow-MyHC, but significantly decreased the protein level of fast-MyHC, which were attenuated by AMP-activated protein kinase (AMPK) inhibitor Compound C, AMPKα2 siRNA or Sirt1 inhibitor EX527. We also showed that EX527 attenuated FA-induced increase in the phosphorylation levels of AMPK and liver kinase B1 (LKB1), indicating that AMPK serves as a downstream target of Sirt1. Our present study provides the first in vitro evidence that FA regulates muscle fiber type formation via the Sirt1/AMPK signal pathway.
Introduction
Skeletal muscle is a heterogeneous tissue composed of different types of fibers, which can be distinguished according to the myosin heavy chain (MyHC) isoform expression. There are four types of muscle fibers in mammals including slow oxidative type I with MyHC I (slow-MyHC), fast oxidative type IIa with MyHC IIa, intermediate type IIx with MyHC IIx and fast glycolytic type IIb with MyHC IIb.1,2 It has been reported that slow oxidative muscle fiber proportion is positively correlated to insulin sensitivity3 and high-quality meat of livestock and poultry.4 Therefore, more and more attention has been paid to promoting slow muscle fiber formation and increasing slow muscle fiber content through nutrition strategies.
Ferulic acid (FA, 3-methoxy-4-hydroxycinnamic acid) is a common polyphenolic compound present in barley grain, wheat bran, rice endosperm, maize bran and other plant cell walls.5 It is covalently linked to polysaccharides by ester bonds.6,7 FA can be released from plant cell walls by the action of feruloyl esterases.8 Numerous studies have shown that FA has a powerful antioxidant effect.5,9,10 FA has also been shown to have an effect of improving mitochondrial function.11,12 It has been proved that improvement of mitochondrial function is beneficial for the functioning of oxidative myofibers.13,14 In addition, several recent studies have reported that polyphenolic compounds such as resveratrol and apple polyphenols can regulate muscle fiber type formation.15,16 However, the effect of FA on muscle fiber type formation is not clear.
In the present study, we investigated the effect of FA on muscle fiber type formation in mouse C2C12 myotubes. We also investigated its molecular mechanism.
Materials and methods
Reagents
The slow-MyHC (1
:
500) and fast-MyHC (1
:
250) antibodies were obtained from Sigma (St Louis, MO, USA). The troponin I slow type (Troponin I-SS) (1
:
1000) antibody was obtained from Santa Cruz Biotechnology (Santa Cruz, CA, USA). PGC-1α (1
:
500), Sirt1 (1
:
1000), total AMPK (1
:
1000), p-AMPK (1
:
1000), total LKB1 (1
:
1000) and p-LKB1 (1
:
1000) antibodies were obtained from Cell Signaling Technology (Danvers, MA, USA). The β-actin (1
:
3000) antibody was obtained from Santa Cruz Biotechnology (Santa Cruz, CA, USA).
Cell culture
C2C12 myoblasts were grown in Dulbecco's modified Eagle's medium (DMEM) supplemented with 10% FBS and 1% antibiotics (100 U ml−1 penicillin and 100 μg L−1 streptomycin) at 37 °C under a 5% CO2 atmosphere. When the cells were grown to 80–90% confluence, cell differentiation was induced using a differentiation medium containing DMEM with 2% horse serum (Hyclone, Logan, UT, USA). After 1 d of differentiation, the cells were treated with FA (dissolved in DMSO). Compound C (AMPK inhibitor) or EX527 (Sirt1 inhibitor) was added 1 h before FA treatment. The medium was then replaced with a fresh differentiation medium daily for 5 days before analysis.
Small RNA interference (siRNA)
Once C2C12 myoblasts reached 80–90% confluence, they were transfected with 50 nM of negative control (NC) siRNA or AMPKα2-specific siRNA (GenePharm, Shanghai, China) using Lipofectamine 3000 (Invitrogen, California, USA) according to the manufacturer's protocol. The siRNA sequences used were 5′-GCAUACCAUCUUCGAGUAATT-3′ (forward) and 5′-UUACUCGAAGAUGG UAUGCTT-3′ (reverse) for AMPKα2, and 5′-UUCUCCGAACGUGUCACGUTT-3′ (forward) and 5′-ACGUGACACGUUCGGAGAATT-3′ (reverse) for NC.
RNA extraction and reverse transcription
Total RNA from the C2C12 myotubes was isolated using RNAiso Plus reagent (TaKaRa, Dalian, China) according to the manufacturer's protocol. The concentration of RNA was determined using a Beckman Coulter DU 800 system (Beckman Coulter, Fullerton, CA, USA). One microgram of total RNA was transcribed into single-stranded cDNA using the PrimeScript® RT reagent kit with gDNA Eraser (TaKaRa) according to the manufacturer's instructions. The cDNA was subsequently used as a template for PCR amplification.
Real-time quantitative PCR
Real-time quantitative PCR was performed in triplicate using the SYBR Select Master Mix (Applied Biosystems, Foster, CA, USA) and an ABI 7900HT real-time PCR detection system. The gene specific primers used are listed in Table 1. The cycling conditions used in real-time quantitative PCR were 45 cycles at 95 °C for 15 s and 60 °C for 30 s. The relative expression of each gene was calculated according to the comparative Ct value method17 and normalized to the expression of glyceraldehyde-3-phosphate dehydrogenase (GAPDH) in the same sample.
Table 1 List of genes, primer sequences, GenBank accession numbers, and product sizes in this study
Gene name |
Primer |
Sequence |
GenBank accession no. |
Product size (bp) |
MyHC I
|
Forward |
5′-CTTCTACAGGCCTGGGCTTAC-3′ |
NM_080728
|
128 |
Reverse |
5′-CTCCTTCTCAGACTTCCGCAG-3′ |
MyHC IIb
|
Forward |
5′-CTTGTCTGACTCAAGCCTGCC-3′ |
NM_010855
|
158 |
Reverse |
5′-TCGCTCCTTTTCAGACTTCCG-3′ |
MHC IIa
|
Forward |
5′-TTCCAGAAGCCTAAGGTGGTC-3′ |
NM_001039545
|
94 |
Reverse |
5′-GCCAGCCAGTGATGTTGTAAT-3′ |
MEF2C
|
Forward |
5′-GATCTCCGCGTTCTTATCCC-3′ |
L13171
|
91 |
Reverse |
5′-CCAATGACTGAGCCGACTG-3′ |
PGC-1α
|
Forward |
5′-CCAGTACAACAATGAGCCTGC-3′ |
NM_008904
|
118 |
Reverse |
5′-CAATCCGTCTTCATCCACG-3′ |
Sirt1
|
Forward |
5′-ACAGTGACAGTGGCACATGC-3′ |
NM_019812
|
130 |
Reverse |
5′-AATCCAGATCCTCCAGCACA-3′ |
AMPKα2
|
Forward |
5′-TCTGGAGGTGAATTGTTCGAC-3′ |
NM_178143
|
146 |
Reverse |
5′-ACATTCTCTGGCTTCAGGTCC-3′ |
GAPDH
|
Forward |
5-AGGGCATCTTGGGCTACAC-3′ |
NM_008084
|
211 |
Reverse |
5′-TGGTCCAGGGTTTCTTACTCC-3′ |
Western blotting analysis
Cells were lysed in RIPA lysis buffer (Pierce, Rockford, IL, USA) containing the protease inhibitor cocktail (Sigma). After centrifugation at 12
000g for 10 min at 4 °C, the supernatant obtained was used for western blot analysis. The protein content was determined using the BCA protein assay kit (Pierce). The proteins were then electrophoresed, transferred, immunoblotted, and visualized as previously described.18 β-Actin was used as a housekeeping protein to control equal loading. The density of the protein bands was determined with Gel-Pro Analyzer Version 4.2 (Media Cybernetics, Rockville, MD, USA).
Metabolic enzyme activity assay
Metabolic enzyme activity assays were performed on cell lysates. The activities of succinate dehydrogenase (SDH) and lactate dehydrogenase (LDH) were measured using commercial assay kits (Nanjing Jiancheng Bioengineering Institute, Nanjing, China). All data were normalized for the corresponding protein concentration of cell lysates.
Statistical analysis
Data are presented as mean ± SE (standard error). The data of Fig. 4B, C, 5 and 6 were analyzed by one-way ANOVA followed by Tukey's tests using SPSS11.5 software. Student's t test was performed to assess the statistical significance between groups in Fig. 1, 2, 3 and 4A. P values < 0.05 were considered significant.
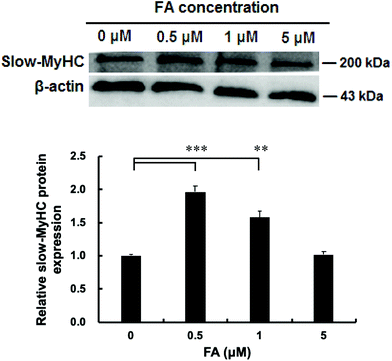 |
| Fig. 1 Effect of FA on the protein expression level of slow-MyHC. C2C12 myoblasts were treated with different concentrations of FA after 1 d of differentiation, and then cultured in the differentiation medium for 5 d. Western blotting analysis was used to analyze the slow-MyHC protein expression normalized to β-actin. Results were the mean and standard errors from three independent experiments. **P < 0.01 and ***P < 0.001 as compared with the control. | |
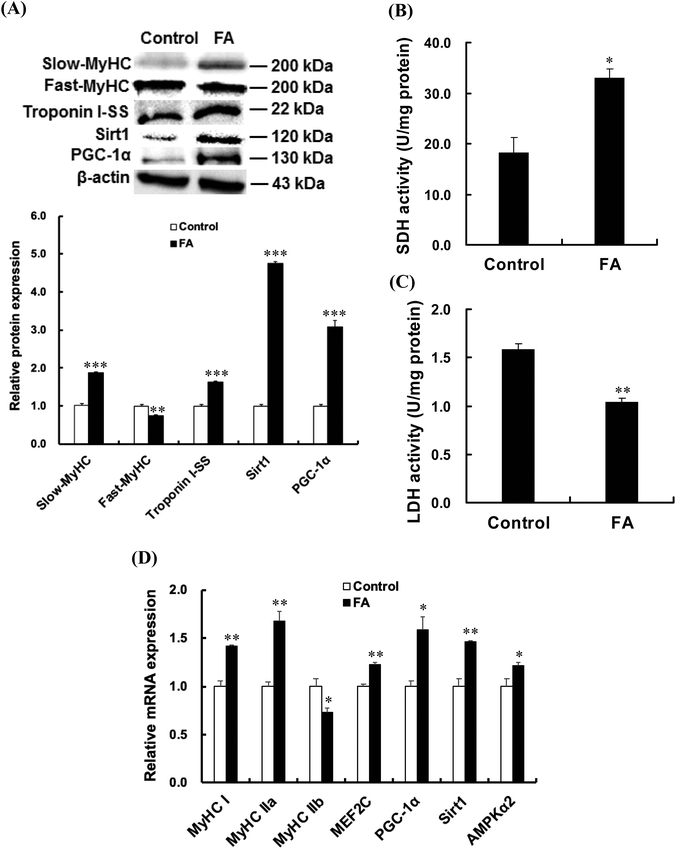 |
| Fig. 2 FA regulates muscle fiber type formation. C2C12 myoblasts were treated with 0.5 μM FA or an equal volume of DMSO (control) after 1 d of differentiation, and then cultured in the differentiation medium for 5 d. (A) Effect of FA on the protein expressions of slow-MyHC, fast-MyHC, Troponin I-SS, Sirt1 and PGC-1α. Equal loading was monitored with the anti-β-actin antibody. (B) Effect of FA on the activity of SDH. (C) Effect of FA on the activity of LDH. (D) Effect of FA on mRNA abundances of MyHC I, MyHC IIa, MyHC IIb, MEF2C, PGC-1α, Sirt1 and AMPKα2. The amount of target gene mRNA was normalized to the amount of GAPDH mRNA. Results were the mean and standard errors from three independent experiments. *P < 0.05, **P < 0.01 and ***P < 0.001 as compared with the control. | |
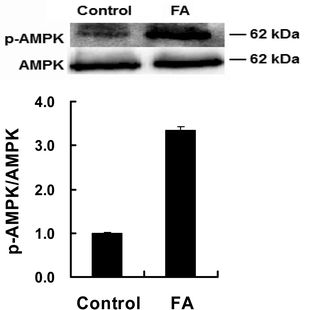 |
| Fig. 3 Effect of FA on p-AMPK protein expression. Samples are prepared as described in Fig. 2. Western blotting analysis was used to analyze the protein expressions of p-AMPK and AMPK. Results were the mean and standard errors from three independent experiments. ***P < 0.001 as compared with the control. | |
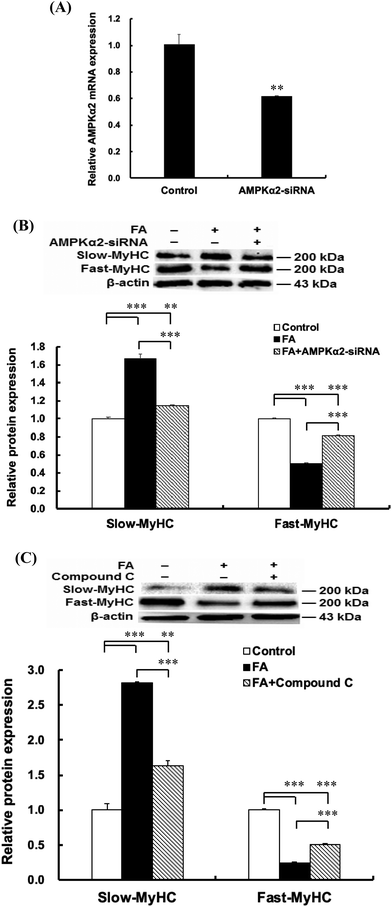 |
| Fig. 4 AMPK signaling pathway contributes to the regulation of muscle fiber type formation by FA. About 90% confluent C2C12 myoblasts cultured in the differentiation medium were transfected with AMPKα2 siRNA (50 nM) or treated with Compound C (50 μM), followed by treatment with 0.5 μM FA or an equal volume of DMSO (control) for 5 d. (A) Effect of AMPKα2 siRNA on AMPKα2 mRNA expression. (B) AMPKα2 siRNA attenuated the effect of FA on the slow-MyHC and fast-MyHC protein expressions. (C) AMPK inhibitor compound C attenuated the effect of FA on the slow-MyHC and fast-MyHC protein expressions. Results were the mean and standard errors from three independent experiments. **P < 0.01 and ***P < 0.001. | |
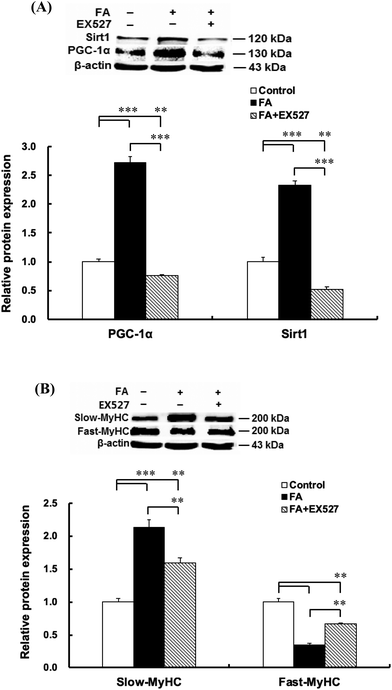 |
| Fig. 5 Sirt1 inhibitor EX527 attenuates the effect of FA on muscle fiber type formation. About 90% confluent C2C12 myoblasts were cultured in the differentiation medium. Twenty-four hours later, cells cultured in the differentiation medium were pretreated with Sirt1 inhibitor EX527 (25 μM) for 1 h and then treated with 0.5 μM FA or an equal volume of DMSO (control) for 5 d. Sirt1 (A), PGC-1α (A), slow-MyHC (B) and fast-MyHC (B) protein expressions were analyzed by western blot. Results were the mean and standard errors from three independent experiments. **P < 0.01 and ***P < 0.001. | |
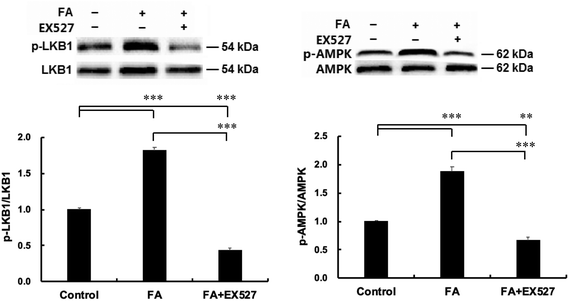 |
| Fig. 6 Sirt1 is required for FA-induced AMPK activation. Samples were prepared as described in Fig. 5. p-LKB1 (A) and p-AMPK (B) protein expressions were analyzed by western blot. Results were the mean and standard errors from three independent experiments. **P < 0.01 and ***P < 0.001. | |
Results
Determination of the optimum level of FA
As shown in Fig. 1, the protein level of slow-MyHC was significantly increased when supplementing with 0.5 μM and 1 μM FA, compared with the unsupplemented control. The maximal increase appeared in the 0.5 μM FA treatment group (Fig. 1). No significant difference was observed between 0 μM and 5 μM FA treatment groups (Fig. 1). Then we chose 0.5 μM of FA for use in our subsequent research.
FA regulates muscle fiber type formation
As shown in Fig. 2A, FA treatment significantly increased the protein expressions of slow-MyHC, troponin I-SS, Sirt1 and PGC-1α, and decreased the protein expression of fast-MyHC. FA treatment significantly increased the activity of SDH (Fig. 2B) and decreased the activity of LDH (Fig. 2C). Real-time quantitative PCR analysis showed that FA significantly increased the mRNA abundances of MyHC I and MyHC IIa but decreased the mRNA abundance of MyHC IIb (Fig. 2D).
AMPK signaling pathway contributes to the regulation of muscle fiber type formation by FA
As shown in Fig. 2D, the AMPKα2, PGC-1α, Sirt1 and MEF2C mRNA abundances in the FA treated group were higher than those in the control group. Compared with the control group, FA treatment significantly increased the p-AMPK protein level (Fig. 3), indicating that FA activates the AMPK signaling pathway.
To explore the mechanism by which FA regulates muscle fiber type formation, C2C12 myoblasts were transfected with AMPKα2 siRNA 24 h before the addition of FA or treated with AMPK inhibitor Compound C 1 h before the addition of FA. The results showed that AMPKα2 siRNA significantly decreased the mRNA abundance of AMPKα2 (Fig. 4A). In addition, AMPKα2 siRNA or AMPK inhibitor Compound C abolished FA-induced upregulation of slow-MyHC and downregulation of fast-MyHC (Fig. 4B and C). Taken together, these results suggested that regulation of muscle fiber type formation by FA is dependent on AMPK signaling.
Inhibition of Sirt1 by EX527 disrupts the effect of FA on muscle fiber type formation
Inhibition of Sirt1 by EX527 significantly decreased the Sirt1 protein level (Fig. 5A). As shown in Fig. 5, EX527 treatment attenuated FA-induced increase in the protein expressions of slow-MyHC and PGC-1α and decrease in the protein expression of fast-MyHC.
Sirt1 is required for FA-induced AMPK activation
As shown in Fig. 6, EX527 treatment attenuated FA-induced activation of LKB1, a primary upstream kinase of AMPK, and also attenuated FA-induced AMPK activation.
Discussion
In this study, we showed that FA significantly increased the mRNA abundances of MyHC I and MyHC IIa, decreased the mRNA abundance of MyHC IIb, increased the protein level of slow-MyHC and decreased the protein level of fast-MyHC. We also showed that FA increased the protein level of slow skeletal Troponin I (troponin I-SS). For the metabolic characteristics of muscle fibers, we showed that FA increased the activity of SDH and decreased the activity of LDH (a key glycolytic enzyme). To the best of our knowledge, our present study provides the first evidence that FA promotes slow oxidative muscle fiber formation and inhibits fast glycolytic muscle fiber formation in C2C12 myotubes. However, FA was reported to promote hypertrophic growth of fast skeletal muscle in adult zebrafish.19 The reason may be that the function of FA is different in mammals and aquatic animals.
The 5′-AMP-activated protein kinase (AMPK) is a heterotrimetric enzyme (α1, α2, β1, β2, γ1, γ2, and γ3) activated by phosphorylation of its α catalytic subunit.20 The AMPK signalling pathway is a well-studied mechanism that regulates myosin heavy chain expression, resulting in a slower, more oxidative phenotype.21 Additionally, AMPK may regulate the expression of PGC-1α, a principal factor in regulating muscle fiber type determination.22 PGC-1α can physically interact with myocyte enhancer factor 2C (MEF2C) to promote the formation of slow-twitch muscle fibers.23 In the present study, we showed that FA not only upregulated the mRNA abundance of AMPKα2, but also increased the protein levels of p-AMPK and PGC-1α. In order to further explore the mechanism by which FA affects myofiber specific gene expression, AMPK signaling was inhibited by AMPKα2 siRNA or AMPK inhibitor compound C. Our data showed that the inhibition of AMPK signalling abolished the effect of FA on muscle fiber type formation, indicating that the regulation of muscle fiber type formation by FA requires AMPK.
In addition, Sirt1 can directly activate LKB1, resulting in the phosphorylation of AMPK.24 It has been reported that overexpression of Sirt1 resulted in more oxidative fibers25 and upregulated PGC-1α expression.26 In the present study, we found that FA-induced increase in the protein expressions of slow-MyHC and PGC-1α and FA-induced decrease in the protein expression of fast-MyHC were attenuated by Sirt1 inhibitor EX527. We also found that EX527 attenuated FA-induced increase in the phosphorylation levels of AMPK and LKB1. These results indicated that the regulation of muscle fiber type formation by FA requires Sirt1 and AMPK serves as a downstream target of Sirt1. Our data support a working model for FA (Fig. 7), that is, FA activates Sirt1, which leads to the activation of LKB1 and AMPK. The activation of Sirt1 and AMPK converges on enhancing the expression of PGC-1α,27 thereby promoting slow oxidative muscle fiber formation.23,28
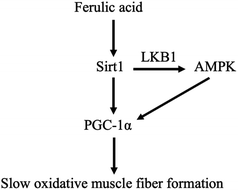 |
| Fig. 7 Scheme illustrating the regulation of muscle fiber type formation by ferulic acid. | |
Conclusion
In summary, we provide the first evidence that FA regulates muscle fiber type formation through the Sirt1/AMPK signaling pathway. Our study not only brings a novel insight into the function of FA in mammals, but also indicates the necessity of further studying the role of FA in skeletal muscle biology in vivo.
Conflicts of interest
There are no conflicts of interest to declare.
Acknowledgements
This work was supported by the National Key R&D Program of China (No. 2018YFD0500403) and the National Natural Science Foundation of China (No. 31672432).
References
- S. Schiaffino and C. Reggiani, Molecular diversity of myofibrillar proteins: Gene regulation and functional significance, Physiol. Rev., 1996, 76, 371–423 CrossRef CAS PubMed.
- L. Lefaucheur, P. Ecolan, L. Plantard and N. Gueguen, New insights into muscle fiber types in the pig, J. Histochem. Cytochem., 2002, 50, 719–730 CrossRef CAS PubMed.
- C. A. Stuart, M. P. McCurry, A. Marino, M. A. South, M. E. A. Howell, A. S. Layne, M. W. Ramsey and M. H. Stone, Slow-twitch fiber proportion in skeletal muscle correlates with insulin responsiveness, J. Clin. Endocrinol. Metab., 2013, 98, 2027–2036 CrossRef CAS PubMed.
- Y. J. Nam, Y. M. Choi, S. H. Lee, J. H. Choe, D. W. Jeong, Y. Y. Kim and B. C. Kim, Sensory evaluations of porcine longissimus dorsi muscle: Relationships with postmortem meat quality traits and muscle fiber characteristics, Meat Sci., 2009, 83, 731–736 CrossRef CAS PubMed.
- S. Mathew and T. E. Abraham, Ferulic acid: an antioxidant found naturally in plant cell walls and feruloyl esterases involved in its release and their applications, Crit. Rev. Biotechnol., 2004, 24, 59–83 CrossRef CAS PubMed.
- I. Mueller-Harvey, R. D. Hartley, P. J. Harris and E. H. Curzon, Linkage of p-coumaroyl and feruloyl groups to cell wall polysaccharides of barley straw, Carbohydr. Res., 1986, 148, 71–85 CrossRef CAS.
- D. W. S. Wong, V. J. Chan, S. B. Batt, G. Sarath and H. Liao, Engineering Saccharomyces cerevisiae to produce feruloyl esterase for the release of ferulic acid from switchgrass, J. Ind. Microbiol. Biotechnol., 2011, 38, 1961–1967 CrossRef CAS PubMed.
- I. Benoit, E. G. Danchin, R. J. Bleichrodt and R. P. de Vries, Biotechnological applications and potential of fungal feruloyl esterases based on prevalence, classification and biochemical diversity, Biotechnol. Lett., 2008, 30, 387–396 CrossRef CAS PubMed.
- M. Heinonen, D. Rein, M. T. Satue-Gracia, S. W. Huang, J. B. German and E. N. Frankel, Effect of protein on the antioxidant activity of phenolics compounds in a lecithin-liposome oxidation system, J. Agric. Food Chem., 1998, 46, 917–922 CrossRef CAS.
- R. J. Jariwalla, Rice-bran products: phytonutrients with potential applications in preventive and clinical medicine, Drugs Exp. Clin. Res., 2001, 27, 17–26 CAS.
- E. Cione, P. Tucci, V. Senatore, M. Perri, S. Trombino, F. Iemma, N. Picci and G. Genchi, Synthesized esters of ferulic acid induce release of cytochrome c from rat testes mitochondria, J. Bioenerg. Biomembr., 2008, 40, 19–26 CrossRef CAS PubMed.
- V. L. K. Muralidhara, Ameliorative Effects of ferulic acid against lead acetate-induced oxidative stress, mitochondrial dysfunctions and toxicity in prepubertal rat brain, Neurochem. Res., 2014, 39, 2501–2515 CrossRef PubMed.
- J. D. Brown, C. R. Hancock, A. D. Mongillo, J. B. Baron, R. A. Digiovanni, A. C. Parcell, W. W. Winder and D. M. Thomson, Effect of LKB1 deficiency on mitochondrial content, fiber type, and muscle performance in the mouse diaphragm, Acta Physiol., 2011, 201, 457–466 CrossRef CAS PubMed.
- P. Mishra, G. Varuzhanyan, A. H. Pham and D. C. Chan, Mitochondrial dynamics is a distinguishing feature of skeletal muscle fiber types and regulates organellar compartmentalization, Cell Metab., 2015, 22, 1033–1044 CrossRef CAS PubMed.
- C. Zhang, J. Luo, B. Yu, P. Zheng, Z. Huang, X. Mao, J. He, J. Yu, J. Chen and D. Chen, Dietary resveratrol supplementation improves meat quality of finishing pigs through changing muscle fiber characteristics and antioxidative status, Meat Sci., 2015, 102, 15–21 CrossRef CAS PubMed.
- W. Mizunoya, S. Okamoto, H. Miyahara, M. Akahoshi, T. Suzuki, M. Q. H. Ohtsubo, Y. Komiya, M. Qahar, T. Waga, K. Nakazato, Y. Ikeuchi, J. E. Anderson and R. Tatsumi, Fast-to-slow shift of muscle fiber-type composition by dietary apple polyphenols in rats: Impact of the low-dose supplementation, Anim. Sci. J., 2017, 88, 489–499 CrossRef CAS PubMed.
- K. J. Livak and T. D. Schmittgen, Analysis of relative gene expression data using real-time quantitative PCR and the 2–ΔΔCt method, Methods, 2001, 25, 402–408 CrossRef CAS PubMed.
- X. L. Chen, Y. L. Luo, Z. Q. Huang, G. Jia, G. M. Liu and H. Zhao, Akirin2 regulates proliferation and differentiation of porcine skeletal muscle satellite cells via ERK1/2 and NFATc1 signaling pathways, Sci. Rep., 2017, 7, 45156 CrossRef CAS PubMed.
- Y. Wen and H. Ushio, Ferulic acid promotes hypertrophic growth of fast skeletal muscle in zebrafish model, Nutrients, 2017, 9, 1066 CrossRef PubMed.
- D. G. Hardie, S. A. Hawley and J. W. Scott, AMP-activated protein kinase: development of the energy sensor concept, J. Physiol., 2006, 574, 7–15 CrossRef CAS PubMed.
- K. S. C. Röckl, M. F. Hirshman, J. Brandauer, N. Fujii, L. A. Witters and L. J. Goodyear, Skeletal muscle adaptation to exercise training AMP-activated protein kinase mediates muscle fiber type shift, Diabetes, 2007, 56, 2062–2069 CrossRef PubMed.
- P. J. Atherton, J. Babraj, J. Singh, M. J. Rennie and H. Wackerhage, Selective activation of AMPK-PGC-1alpha or PKB-TSC2-mTOR signaling can explain specific adaptive responses to endurance or resistance training-like electrical muscle stimulation, FASEB J., 2005, 19, 786–788 CrossRef CAS PubMed.
- J. Lin, H. Wu, P. T. Tarr, C. Y. Zhang, Z. Wu, O. Boss, L. F. Michael, P. Puigserver, E. Isotani, E. N. Olson, B. B. Lowell, R. Bassel-Duby and B. M. Spiegelman, Transcriptional co-activator PGC-1α drives the formation of slow-twitch muscle fibres, Nature, 2002, 418, 797–801 CrossRef CAS PubMed.
- N. B. Ruderman, D. Carling, M. Prentki and J. M. Cacicedo, AMPK, insulin resistance, and the metabolic syndrome, J. Clin. Invest., 2013, 123, 2764–2772 CrossRef CAS PubMed.
- A. Chalkiadaki, M. Igarashi, A. S. Nasamu, J. Knezevic and L. Guarente, Muscle-specific Sirt1 gain-of-function increases slow-twitch fibers and ameliorates pathophysiology in a mouse model of duchenne muscular dystrophy, PLoS Genet., 2014, 10, e1004490 CrossRef PubMed.
- J. T. Rodgers, C. Lerin, W. Haas, S. P. Gygi, B. M. Spiegelman and P. Puigserver, Nutrient control of glucose homeostasis through a complex of PGC-1α and SIRT1, Nature, 2005, 434, 113–118 CrossRef CAS PubMed.
- M. D. J. Chau, J. Gao, Q. Yang, Z. Wu and J. Gromada, Fibroblast growth factor 21 regulates energy metabolism by activating the AMPK-SIRT1-PGC-1α pathway, Proc. Natl. Acad. Sci. U. S. A., 2010, 107, 12553–12558 CrossRef CAS PubMed.
- M. J. Potthoff, H. Wu, M. A. Arnold, J. M. Shelton, J. Backs, J. McAnally, J. A. Richardson, R. Bassel-Duby and E. N. Olson, Histone deacetylase degradation and MEF1 activation promote the formation of slow-twitch myofibers, J. Clin. Invest., 2007, 117, 2459–2467 CrossRef CAS PubMed.
|
This journal is © The Royal Society of Chemistry 2019 |