Nafion particles doped with methyl viologen: electrochemistry†
Received
3rd October 2017
, Accepted 4th December 2017
First published on 4th December 2017
Abstract
Nafion sub-micro particles doped with methyl viologen (MV2+) are synthesized using the re-precipitation method and characterized by scanning electron microscopy and UV-Vis spectroscopy. The electrochemical behavior of MV2+ incorporated into Nafion particles was investigated at both the ensemble and single particle levels. The charge transferred to single MV-Nafion particles was observed using the nano-impact method and shown to be quantitative. Finally, the charge transferred via individual MV-Nafion particle was substantially enhanced in the presence of permanganate. The mechanism of the catalytic nano-impact reaction of mediated permanganate reduction by single MV-Nafion particle is revealed as proceeding via MV+˙ reduced from MV2+ incorporated in Nafion particles through one electron transfer followed by the reduction of permanganate.
1. Introduction
Nanoscale and sub-microscale particles have received a tremendous amount of attention in recent decades due to their structure-dependent properties which can deviate significantly from those of bulk materials. They have been shown to play a key role in many technical applications, such as catalysis, energy conversion and storage, spectroscopy, and the pharmaceutical industry.1,2 Organic nanoparticles are prominent candidates for drug delivery in the biomedical field,3 such as for delivering hydrophobic drugs to target cells,4 and as carriers for the controlled release of drugs under specific stimuli.5 Organic particles, including polymer particles, are usually prepared through the polymerization of suitable monomers or re-precipitation methods. These particles are conventionally characterized as an ensemble, thus, the performance of individual particles is poorly understood, and single particle peculiarities are averaged out during an ensemble analysis. Therefore, there is an urgent demand to detect and characterise particles in real-time which requires an efficient technique for particle detection and quantification.
Nafion is a perfluorinated sulfonic-acid ionomer, which is most commonly used as a proton-exchange membrane and as a cation exchange polymer in sustainable energy technologies (fuel cells, batteries and solar cells) and chemical sensors due to its attractive electrochemical and thermal properties.6,7 The structure of Nafion consists of an electrically neutral fluorocarbon backbone (polytetrafluoroethylene) and a randomly tethered side-chain with a pendant ionic group, SO3−. Nafion membranes incorporating redox functional molecules (e.g. methyl viologen,8–12 Ru(bpy)32+,13–15 and platinum,16etc.) have been extensively investigated. A solid lipid particle based on Nafion with the incorporation of a complex of platinum coordinated with PTA has been reported and should favour the delivery and activity of platinum-based drugs against cancers.17 However, there are no investigations on the properties of nanoscale or sub-microscale Nafion entities or their derivatives doped with electroactive molecules by electrochemistry.
Methyl viologen (MV2+) plays an important role as an electron relay in systems in which electron transfer is initiated by electrochemical processes. It has been extensively studied in photoelectrochemical energy storage8,18–20 and electrochemical biosensors.21–23 The electrochemical behavior of methyl viologen involves the reduction of MV2+ by a reversible one-electron reaction to a radical cation, which can be further reduced to the neutral form that tends to adsorb on the electrode surface (Scheme 1).24 MV2+ is reported as an electrocatalyst for the reductions of permanganate,12 oxygen21 and Fe(CN)63−.25 Furthermore the electrochemistry of MV2+ incorporated into different supports such as silica particles26–28 and Nafion films8–12 has previously been studied. The latter were usually investigated by modifying an electrode with a MV2+ incorporated Nafion film.
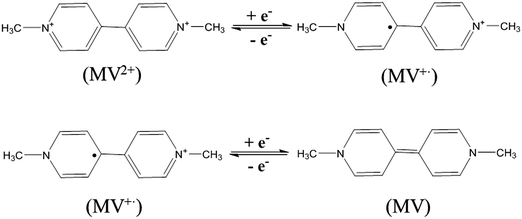 |
| Scheme 1 The structure and the electrochemical reduction of methyl viologen. | |
In this work, Nafion sub-micro particles doped with MV2+ are synthesized using the re-precipitation method.29,30 The reduction of MV2+ incorporated in Nafion particles and the catalytic reactions in the presence of permanganate are electrochemically investigated at both the ensemble and single particle levels. The nano-impact method studying single particle electrochemical behaviour31 not only provides an estimation of the amount of electroactive molecules doped onto each particle, but also shows current spike amplification from MV2+ reduction catalysed by the reaction of permanganate with the impacting MV-Nafion particles, demonstrating the potential use of MV-Nafion particles for electrocatalysis. Thus, the synthesized MV-Nafion particles may hold great potential in the applications of energy transformation technologies and sensors.
2. Experimental
2.1 Chemicals
Methyl viologen (N,N′-dimethyl-4,4′-bipyridinium dichloride, MV2+˙Cl2) was purchased from Aldrich and used as received. Nafion® perfluorinated resin solution was supplied by Aldrich (5 wt% in lower aliphatic alcohol and water (45%)). The phosphate buffer solution (pH 7.4) was composed of 10 mM each of monobasic and dibasic potassium phosphate, together with 0.1 M KCl. All solutions were made using ultrapure water of resistivity 18.2 MΩ cm (Millipore) at 25 °C and degassed thoroughly with N2 (oxygen-free, BOC Gases Plc) before use.
2.2 Synthesis of MV-Nafion particles
Nafion particles bulk modified with MV2+ were synthesized by the re-precipitation method.29,30 5 μL of 100 mM MV2+ aqueous solution was mixed with 100 μL condensed Nafion (12.5 wt%).32 The mixture was then injected dropwise into 2 mL deionized water under vigorous stirring at 600 rpm for 5 min. The obtained transparent solution was subjected to sonication for 30 min at room temperature, followed by centrifugation at 10
000 rpm for 10 min. To remove excess MV2+, the precipitate was washed with water three times. MV-Nafion particles were then obtained and dispersed in 1 mL deionized water to make a suspension.
2.3 Characterization of MV-Nafion particles
The size of the MV-Nafion particles was characterized by scanning electron microscopy (SEM). A scanning microscope (JEOL JSM-6500F) was used and an accelerating voltage of 5 kV was applied. SEM was conducted on a dropcast of the particles onto a glassy carbon substrate. Characterization of the Nafion particles with and without encapsulated MV2+ was further performed by UV-Vis spectroscopy (U-2001, Hitachi, Mannheim, Germany) with a UV-probe 2.5 as an operating interface. Unreacted MV2+ (λ = 257 nm)33,34 in the supernatant was analyzed. For all such measurements a quartz cell with a 1 cm optical path was used.
2.4 Carbon fibre micro wire electrode fabrication
Cylindrical microelectrodes of approximately 1 mm in length were utilised for impact experiments and fabricated with the previous method designed by Ellison et al.35 First, a 7.0 μm diameter carbon fibre (Goodfellow Cambridge Ltd) was connected to a conducting metal wire using a silver epoxy (RS Components Ltd) conductive adhesive. The adhesive was set by heat treatment in an oven for 15 min at approximately 60 °C. Then the wire was threaded through a plastic pipette tip so that only the carbon fibre extended out of the end. Cyanoacrylate adhesive was then used to seal the interstice between the carbon fibre/metal wire and the plastic pipette tip. After holding the tip in air overnight to set the adhesive, the carbon fibre tip was cut down so that a length of approximately 1 mm protruded past the sealed pipette end.
2.5 Electrochemical analysis
Cyclic voltammetry of dropcast MV-Nafion particles.
All electrochemical experiments were conducted in a conventional three-electrode setup in a Faraday cage under a N2 atmosphere. A μAutolab II (Metrohm-Autolab BV, Utrecht, The Netherlands) was used to control the electrochemical experiments using NOVA 1.10 software. The temperature of the electrochemical cell was maintained at 25 °C. For cyclic voltammetry experiments, a glassy carbon (GC) electrode (CH instruments, Austin, USA) of 3.0 mm diameter was used as the working electrode, with a saturated calomel electrode (SCE, ALS distributed by BASi, Tokyo, Japan) as the reference electrode and a platinum foil (99.99% GoodFellow, Cambridge, UK) as the counter electrode. The GC electrode was polished with aqueous slurries of 1, 0.3 and 0.05 μm alumina for 3 to 5 min each in descending order of size. Alumina residue was removed from the electrode by rinsing with plenty of water and the electrode was dried with nitrogen. A small aliquot (2.5 to 20 μL) of an aqueous suspension of the MV-Nafion particles was placed on the clean GC electrode by drop-casting and dried under a nitrogen atmosphere. Once dried, the modified electrode was thoroughly rinsed with ultrapure water and placed in a deoxygenated 0.01 M PBS (pH 7.4) and 0.10 M KCl. The potential was swept between 0 V and −1.3 V vs. SCE. This experiment was repeated using an equal amount of undoped Nafion particles for comparison. Note that the voltammograms shown in this paper (Fig. 2, 4, 5 and Fig. S1, S2, ESI†) are the first scans unless specified otherwise.
Nano-impact experiments.
Nano-impact experiments were also conducted by using the μAutolab II (Metrohm Autolab BV, Utrecht, The Netherlands) in a Faraday cage with a three-electrode system at 298 K. A carbon microwire electrode (ca. 7 μm in diameter and ca. 1 mm in length), fabricated according to the procedure introduced in our previous work,35 was used as the working electrode. The same reference and counter electrodes were applied as above.5 mL of 10 mM PBS (pH 7.4) with 0.10 M KCl was degassed thoroughly with nitrogen. A known concentration of MV-Nafion particles was then added. The cell was sealed and sonicated for 2 min to obtain a well dispersed suspension. Chronoamperograms were recorded for the duration of 50 s with a 0.5 ms measurement interval at the bias potential of between −0.5 and −1.3 V vs. SCE was performed with an atmosphere of N2 maintained above the solution. Control experiments were carried out in the same buffer solution with Nafion particles without doping with MV2+.
Transient current response or “spikes” resulting from particles colliding with the micro-wire electrode were analyzed using SignalCounter software developed by Dario Omanovic (Centre for Marine and Environmental Research, Ruder Boskovic Institute, Croatia).36 Note that the employed potentiostat (Metrohm micro Autolab Type II) was characterized in our previous work37 where we showed that the overall amount of charge is conserved even if the measured signals exceed the bandwidth of the potentiostat.
3. Results and discussion
In this section, the morphological characteristics of the MV-Nafion particles synthesized in this work are addressed by scanning electron microscopy (SEM) and the amount of MV2+ doped into Nafion structure is inferred by UV-Vis spectroscopy. Then, the redox properties of MV-Nafion particles ensembles coated on a GCE are studied using cyclic voltammetry. Subsequently, nano-impact chronoamperometry is employed to investigate the electrochemical reduction of MV-Nafion particles in solution with charge transfer from/to the carbon fibre wire electrode. The charge transferred to a single MV-Nafion particle suspended in solution as measured via chronoamperometry is compared with the ensembles’ response on a macroelectrode as measured using cyclic voltammetry and the amount of embedded MV2+ inferred. Finally, the electrocatalytic behavior of MV-Nafion particles was studied in the presence of permanganate at both the ensemble and single particle levels.
3.1 Characterization of Nafion particles doped with MV2+
The MV-Nafion particles synthesized by the re-precipitation method were characterized by SEM and UV-Vis spectroscopy. The morphology of the particles is shown in the SEM image provided in Fig. 1a and appears roughly spheroidal but also with some irregular shapes. The average radius was estimated based on the longest dimension to be 0.43 ± 0.26 μm based on the 243 particles that were identified from the electron micrographs. This may slightly underestimate the size of the particles in the solution because of the water swelling effect which has been found to make Nafion membranes swell about 10–20%.38 The size distribution of the measured particles is shown in Fig. 1a. During the synthesis process of the MV-Nafion particles, Nafion in alcohol/water then mixed with MV2+ was introduced into water in which the polymer of Nafion has very low solubility. Polymer chains tend to avoid contact with water and consequently it is thought that in order to achieve minimum exposure they fold into roughly spherical shapes.3 The particle drying process for SEM analysis is likely responsible for the relatively broad size distribution and the irregular shape. Note that dynamic light scattering analysis was attempted but no appropriate size information was given, which may be ascribed to the polydispersity of the Nafion particles.
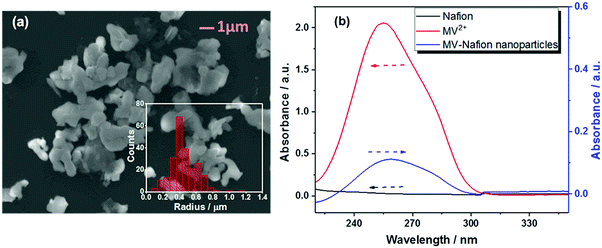 |
| Fig. 1 (a) SEM image of MV-Nafion particles (the scale bar indicates 1.0 μm) along with a histogram that depicts the size distribution of 243 individual MV-Nafion particles. The average radius based on the longest dimension is 0.43 ± 0.26 μm. (b) UV-Vis spectra of the Nafion alcohol/water solution (black curve), 1.25 × 10−4 M MV2+ aqueous solution (red curve) and 3.1 × 10−11 M MV-Nafion particle suspension in water (blue curve). | |
UV-Vis spectroscopy demonstrates that the Nafion solution (in alcohol/water) exhibits no absorbance peak (black curve), while for an MV2+ aqueous solution (red curve), one absorption band centered at 257 nm is observed as shown in Fig. 1b. The absorption band is characteristic of the dication form of methyl viologen, which is assigned to the charge transfer π–π* transitions.33,34 The characteristic absorption peak of MV2+ was also observed in the suspension of MV-Nafion particles (blue curve), evidencing that the MV2+ is incorporated in the Nafion structures. MV2+ molecules are incorporated into Nafion probably due to the strong electrostatic interactions between the two cationic pyridinium groups of MV2+ and the sulfonate sites of Nafion.8–11,39 The maximum uptake of MV2+ molecules into one Nafion particle was estimated to be (2.0 ± 0.2) × 106 (Section 2, ESI†).
3.2 Cyclic voltammetric studies
Having characterized the synthesized MV-Nafion particles, the electrochemical behavior of ensembles of MV-Nafion particles was investigated using cyclic voltammetry by drop-casting a suspension of MV-Nafion particles onto the surface of a GC electrode. The voltammetric response of a 1.0 mM MV2+ solution at a bare GC electrode between 0 V and −1.4 V vs. SCE was recorded. As shown in Fig. 2 (red solid curve), two pairs of redox peaks were seen centered at around −0.71 V and −1.04 V vs. SCE corresponding to the MV2+/MV+˙, and MV+˙/MV redox transitions, respectively. The dication MV2+ undergoes a reversible one-electron reduction to a radical cation MV+˙ and a second reduction to MV (Scheme 1). A one-electron transfer occurs in each redox reaction in light of the reported mechanism of the electrochemical reduction of methyl viologen.8,9,39,40 Next, a GC electrode dropcast with 1.8 × 108 MV-Nafion particles was also anodically scanned. Assuming a homogeneous coverage over the surface this amount of material would constitute about 15 layers of particles on the surface (Section 2, ESI†). There are two characteristic reduction peaks observed at around −0.69 V and −1.22 V vs. SCE as shown in Fig. 2a (blue dashed curve). A control experiment was conducted by dropcasting the same amount of an unmodified Nafion particle suspension onto the GC electrode surface. No redox signal was observed due to the absence of MV2+ (Fig. 2, black dot curve), evidencing the successful incorporation of MV2+ in the MV-Nafion particles.
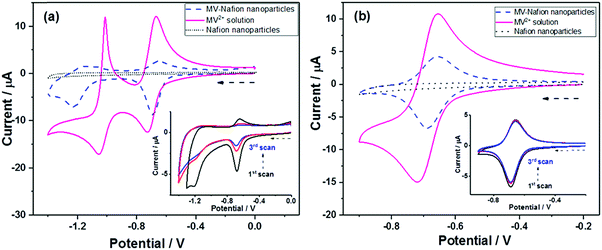 |
| Fig. 2 Voltammograms of a GC electrode (d = 3 mm) in 1.0 mM MV2+ solution (red solid curve), GC electrode modified with 1.8 × 108 Nafion particles (black dot curve) or MV-Nafion particles (blue dashed curve) in 10 mM phosphate buffer (pH 7.4) with 0.1 M KCl at the scan rate of 0.1 V s−1. (a) Potential scan range 0 to −1.4 V; (b) potential scan range −0.2 to −0.9 V. Insets in (a) and (b) show the first three scans of the dropcast MV-Nafion particles at the two potential windows. | |
Fig. 2(b) shows a voltammogram seen over a reduced potential window corresponding to the MV2+/MV+˙ couple only. It is noticeable that the charge passed in both the oxidizing and reducing directions is similar suggesting that the MV+˙ is retained in the particles after formation. In contrast, Fig. 2(a) shows much smaller oxidation peaks in comparison with the reduction peaks indicating that the neutrally charged MV is rapidly lost from the particles.
Based on the maximum uptake of MV2+ (2.0 ± 0.2 × 106) into one Nafion particle from the UV-Vis analysis, the expected maximum charge from 1.8 × 108 MV-Nafion particles can be estimated to be 5.9 ± 0.7 × 10−5 C (assuming one-electron transfer from MV2+ to the radical ion MV+˙). In contrast, the charge (Qcv) obtained by integrating the first reduction peak in the voltammogram (blue dashed curve) of Fig. 2 is 5.2 ± 0.6 × 10−6 C, only a fraction (ca. 10%) of the expected maximum charge.41 Similarly, only a small fraction of the current response was also observed for different dropcast amounts of MV-Nafion particles with a range from 4 to 30 layers (Fig. S1, ESI†). The drop-cast technique studying the ensembles of particles not only leads to the low efficiency of the redox reaction, but also makes it impossible to estimate the amount of the MV2+ incorporated in each MV-Nafion particle. Therefore, the nano-impact method which enables studying single particles is applied in the following section.
The voltammetric response of the drop-cast MV-Nafion particles at a series of scan rates ranging from 0.005 V s−1 to 0.800 V s−1 was also investigated (Fig. S2, ESI†). The first reduction peak current of MV2+ to MV+ was found to vary linearly with the square root of the scan rate suggesting that it is under diffusional control.8–11 The diffusion coefficient was estimated to be 1.4 × 10−8 cm2 s−1 according to the Randles–Sevcik equation (Section 5, ESI†), which is a little larger than the reported values of methyl viologen incorporated in the Nafion film (1.8–8.0 × 10−9 cm2 s−1) but given the approximation of the transport within the particles layer as linear diffusion this represents a consistent agreement.8,9,39 Furthermore the value of Dapp includes contributions from actual physical diffusion as well as diffusion via electron transfer between neighboring electroactive MV2+ centers.9 The concentration of methyl viologen and the hydrophilic channels in Nafion are speculated to play significant roles in determining the Dapp.8,9,11
3.3 Nano-impacts of individual MV-Nafion particle
With the aim of characterizing the extent of electron transfer to individual MV-Nafion particles, nano-impact experiments were performed using a carbon micro wire working electrode via chronoamperometry. Chronoamperograms were recorded in deaerated 10 mM PBS (pH 7.4) and 0.1 M KCl containing 3.1 × 10−12 M MV-Nafion particles at various applied potentials with the duration time of 50 s. A representative chronoamperogram at −0.8 V is shown in Fig. 3. Typical reduction (Faradaic) spikes are magnified and shown in the inset. For comparison, the chronoamperogram is recorded at −0.8 V in a suspension of Nafion particles without MV2+ incorporated. No spikes in the current transients are observed (red line). In contrast, a total of 350 spikes were observed at −0.8 V over 23 scans for 3.1 × 10−12 M MV-Nafion particles. This suggests that the current spikes are from the electron transfer to an individual MV-Nafion particle upon collision with the micro electrode.
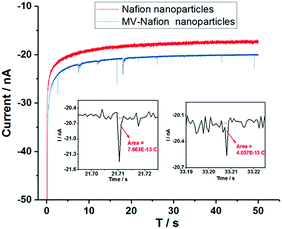 |
| Fig. 3 Representative chronoamperometric scans for Nafion particles (red curve) and the MV-Nafion particle (blue curve) suspension (concentration of particles in both cases is 3.1 × 10−12 M) with 10 mM PBS (pH 7.4) and 0.1 M KCl at −0.8 V (vs. SCE). The inset shows a zoom-in of a single impact spike. | |
The charge passed during the collision between particles and the micro electrode was determined by integrating the area under each individual transient signal. The histograms of the integrated charges under transient signals collected at −0.8 V is shown (Fig. S3a, ESI†), with the mean charge value of 0.40 ± 0.03 pC (the error of the mean charge is the standard error of the mean given by SD/(n)1/2 where SD is the standard deviation and n is the number of spikes). The corresponding number of reduced MV2+ (NMV2+) molecules in each MV-Nafion particle can be inferred to be 2.5 ± 0.2 × 106 based on one electron transfer for each MV2+ molecule. This is close to the value of 2.0 ± 0.2 × 106 estimated from the UV-Vis analysis.
To find the influence of the electrode potential on the impact experiment, chronoamperograms were recorded at different potentials from −0.5 V to −1.3 V, in deaerated 10 mM PBS (pH 7.4) and 0.1 M KCl containing 3.1 × 10−12 M MV-Nafion particles. By integrating the area of each spike as before, the mean charge of the individual spikes was calculated and was plotted as a function of potential, as illustrated in Fig. 4 (the number of spikes at each potential are labelled inside). No spikes were observed for the potentials of −0.5 V and −0.6 V. Therefore, in Fig. 4, a charge value of 0 pC is given at these potentials. At the onset potentials of MV2+ reduction in the CV of the GC electrode modified particles ensembles (Fig. 4, blue curve), spikes are observed, evidencing that the detected current spikes correspond to Faradaic currents associated with MV2+ reduction by the individual impact with MV-Nafion particles. Furthermore the average charge increases with a negative shift of the applied potential to the region where MV2+ undergoes a two electron reduction. The value of the charge at −1.3 V (0.69 ± 0.09 pC) is approximately 2 times of the charge measured at −0.8 V (0.4 ± 0.03 pC), confirming a second electron transfer resulted from the subsequent reduction of MV(I) to MV(0) upon a particle impacting at a higher potential such as −1.3 V, as illustrated in Scheme 2.
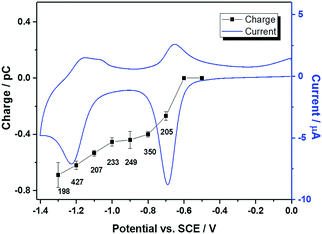 |
| Fig. 4 The average charge of the spikes as a function of applied potential (black squares). The numbers of spikes are labelled. The blue line is the cyclic voltammogram of MV-Nafion particles ensembles on a GC electrode. | |
 |
| Scheme 2 Likely mechanism of the reduction of single MV-Nafion particles. | |
3.4 Electrocatalytic reduction of permanganate by MV-Nafion particles at both the ensemble and single particle levels
It has been reported that the radical cation methyl viologen (MV+˙) can be oxidized by oxidants, such as permanganate,12 or Fe(CN)63−
25 in aqueous solution. Based on this, the electrochemical catalytic performance of the MV-Nafion particles in permanganate solution was investigated at both the ensemble and single particle levels.
First, the electrochemical behavior of Nafion particles and MV-Nafion particles drop-casted on a GC electrode in the absence and presence of 1.0 mM KMO4 was separately studied via cyclic voltammetry at the potential range of −0.2 V to −0.9 V vs. SCE. The first one-electron reductive reaction of MV2+ to MV+˙ is addressed. As shown in Fig. 5, no peak is observed at the GC electrode modified with Nafion particles in the absence (green dot line) and presence (blue dashed line) of 1.0 mM MnO4−. The black solid line in Fig. 5 shows a typical redox characteristic of the 1.8 × 108 MV-Nafion particle coated electrode surface with the peak centered at −0.7 V corresponding to the MV2+/MV+ redox transition. Upon addition of 1.0 mM MnO4−, the cathodic peak current at a potential of around −0.7 V increases greatly while the corresponding oxidation peak current is depressed upon the reverse scan (red dot dashed line). Therefore the electrocatalytic reduction of MV2+ by permanganate can be surmised to occur, which is consistent with the reported electrocatalytic first reduction of MV2+ on a GC electrode coated with a Nafion film.12 The catalytic wave results from the oxidation of the radical cation (MV+˙) by the permanganate oxidant.12,25 Note that the voltammogram for the catalytic reaction is strongly peak shaped with the catalysis seemingly switching off at high negative potentials.42 This likely reflects the onset of the second peak ‘killing’ the catalysis or possible inhibition by the product.
 |
| Fig. 5 Cyclic voltammograms of Nafion particles/GCE and MV-Nafion particles/GCE (1.8 × 108 particles) in the absence and presence of 1.0 mM KMnO4 supported with 10 mM PBS (pH 7.4) and 0.1 M KCl at the scan rate of 0.1 V s−1. | |
Next, the electrochemical behavior of MV-Nafion particles in the presence of MnO4− at the single particle level was investigated via the nano-impact method using the chronoamperometric technique.31,43–45
The chronoamperometric nano-impact method reflects the electron transfer to individual MV-Nafion particles. Fig. 6a shows typical chronoamperometric profiles of a carbon microwire electrode at −0.8 V in a suspension of MV-Nafion particles (3.1 × 10−12 M) which contains 0 mM (blue curve) and 1.0 mM MnO4− (red curve), respectively. It can be seen that a series of well-resolved spikes are observed (Fig. 6a, red curve), and the current spikes are significantly enhanced in the presence of MnO4−. The corresponding average charge of individual spikes from a total of 215 spikes in 16 scans is around 7.3 ± 0.48 pC, which is ca. 18 times of the value (0.40 ± 0.03 pC) in the absence of permanganate as reported above. The histograms of charge passed per spike of MV-Nafion particle in the absence and presence of permanganate are shown in Fig. 6b. This significant enhancement of the current spikes in the presence of the permanganate is ascribed to the electrocatalytic effects of permanganate on the reduction reactions of MV-Nafion particles. The difference in the charge transferred during the reduction processes of MV-Nafion particles in the absence and presence of permanganate are explained according to the chemical reaction between MnO4− and MV+˙.12 Note that the slightly higher background current seen in the presence of the MnO4− is likely attributed to the slow reduction of the MnO4− on the wire electrode surface.
 |
| Fig. 6 (a) Chronoamperometric profiles showing the reductive faradaic spikes of MV-Nafion (3.1 × 10−12 M) in 0 mM (blue curve) and 1.0 mM KMnO4 (red curve) solution with 10 mM PBS buffer (pH 7.4) and 0.1 M KCl at −0.8 V vs. SCE. (b) Histogram showing the reduction charges transferred per individual impact spike of the MV-Nafion particles in 0 and 1.0 mM KMnO4 solution. Inset: A zoom-in image. | |
As illustrated in Scheme 3, in the presence of MnO4−, the one-electron reduction product of MV2+ namely the cation radical MV+˙ within the Nafion particles is oxidized by MnO4− likely via the reaction at the particle surface coupled with diffusion of MV+˙ in the particle. Then, the resulting MV2+ is further reduced electrochemically upon collision with the carbon fibre micro electrode leading to a catalytic cycle, and thus contributing to the enhanced charge of the spikes. Permanganate thus enhances the charge transfer by a chemical reaction and electrocatalytic reduction whereas in the absence of permanganate, the simple one electron reduction of MV-Nafion particles occurs, as in Scheme 2. This is consistent with the experimentally observed increase of the reduction peak at −0.7 V in the cyclic voltammograms (Fig. 5).
 |
| Scheme 3 Illustration of the electrocatalytic reduction of permanganate mediated by an individual MV-Nafion particle at a microelectrode. | |
4. Conclusions
MV-Nafion particles can be synthesized via the re-precipitation method and characterized with SEM and UV-Vis spectroscopy. Voltammetry of the drop-casted MV-Nafion particles evidenced the successful incorporation of MV2+ into the Nafion particle structure but only a small fraction of MV2+ was detected due to the likely agglomerated and irregular ‘mat’ associated with the dropcast technique. In contrast, the nano-impact experiments which enable the detection of single MV-Nafion particles provide a quantitative determination of the amount of MV2+ molecules in each particle. Furthermore, the electrocatalytic reduction of MV2+ incorporated in the MV-Nafion particle was investigated at the ensemble and single particle levels in the presence of permanganate and the charge transferred per spike was enhanced evidencing the catalysis of MV+˙ by MnO4−.
Conflicts of interest
There are no conflicts to declare.
Acknowledgements
This research was supported by the European Research Council (ERC) under the European Union's Seventh Framework Programme (FP/2007–2013), ERC Grant Agreement No. 320403. The Shanxi Scholarship Council of China (No. 20161619) is gratefully acknowledged. X. Li thanks the China Scholarship Council for support.
References
- Q. Zhang, E. Uchaker, S. L. Candelaria and G. Cao, Chem. Soc. Rev., 2013, 42, 3127–3171 RSC.
- A. R. Kirtane and J. Panyam, Nat. Nanotechnol., 2013, 8, 805–806 CrossRef CAS PubMed.
- D. Tuncel and H. V. Demir, Nanoscale, 2010, 2, 484–494 RSC.
- Y. Namiki, T. Namiki, H. Yoshida, Y. Ishii, A. Tsubota, S. Koido, K. Nariai, M. Mitsunaga, S. Yanagisawa, H. Kashiwagi, Y. Mabashi, Y. Yumoto, S. Hoshina, K. Fujise and N. Tada, Nat. Nanotechnol., 2009, 4, 598–606 CrossRef CAS PubMed.
- R. Tong, H. D. Hemmati, R. Langer and D. S. Kohane, J. Am. Chem. Soc., 2012, 134, 8848–8855 CrossRef CAS PubMed.
- A. Kusoglu and A. Z. Weber, Chem. Rev., 2017, 117, 987–1104 CrossRef CAS PubMed.
- K. A. Mauritz and R. B. Moore, Chem. Rev., 2004, 104, 4535–4586 CrossRef CAS PubMed.
- A. M. Hodges, O. Johansen, J. W. Loder, A. W. Mau, J. Rabani and W. H. Sasse, J. Phys. Chem., 1991, 95, 5966–5970 CrossRef CAS.
- J. G. Gaudiello, P. K. Ghosh and A. J. Bard, J. Am. Chem. Soc., 1985, 107, 3027–3032 CrossRef CAS.
- R. J. Mortimer and J. L. Dillingham, J. Electrochem. Soc., 1997, 144, 1549–1553 CrossRef CAS.
- O. Johansen, J. W. Loder, A. W. Mau, J. Rabani and W. H. Sasse, Langmuir, 1992, 8, 2577–2581 CrossRef CAS.
- T.-H. Lu and I. W. Sun, Talanta, 2000, 53, 443–451 CrossRef CAS PubMed.
- D. A. Buttry and F. C. Anson, J. Am. Chem. Soc., 1982, 104, 4824–4829 CrossRef CAS.
- C. R. Martin, I. Rubinstein and A. J. Bard, J. Am. Chem. Soc., 1982, 104, 4817–4824 CrossRef CAS.
- I. Rubinstein and A. J. Bard, J. Am. Chem. Soc., 1981, 103, 5007–5013 CrossRef CAS.
- S.-A. Sheppard, S. A. Campbell, J. R. Smith, G. W. Lloyd, F. C. Walsh and T. R. Ralph, Analyst, 1998, 123, 1923–1929 RSC.
- M. Sguizzato, E. Esposito, M. Drechsler, E. Gallerani, R. Gavioli, P. Mariani, F. Carducci, R. Cortesi and P. Bergamini, J. Chem., 2017, 1–6 Search PubMed.
- N. Plumeré, O. Rüdiger, A. A. Oughli, R. Williams, J. Vivekananthan, S. Pöller, W. Schuhmann and W. Lubitz, Nat. Chem., 2014, 6, 822–827 CrossRef PubMed.
- A. Ruff, J. Szczesny, S. Zacarias, I. A. C. Pereira, N. Plumeré and W. Schuhmann, ACS Energy Lett., 2017, 2, 964–968 CrossRef.
- C. Tapia, R. D. Milton, G. Pankratova, S. D. Minteer, H.-E. Åkerlund, D. Leech, A. L. De Lacey, M. Pita and L. Gorton, ChemElectroChem, 2017, 4, 90–95 CrossRef CAS.
- R. Maalouf, H. Chebib, Y. Saïkali, O. Vittori, M. Sigaud and N. Jaffrezic-Renault, Biosens. Bioelectron., 2007, 22, 2682–2688 CrossRef CAS PubMed.
- X. Wang, S. V. Dzyadevych, J.-M. Chovelon, N. J. Renault, L. Chen, S. Xia and J. Zhao, Electrochem. Commun., 2006, 8, 201–205 CrossRef CAS.
- L. M. Niu, F. Liu, W. Wang, K. Q. Lian, L. Ma, H. M. Shi and W. J. Kang, Electrochim. Acta, 2015, 153, 190–199 CrossRef CAS.
- C. L. Bird and A. T. Kuhn, Chem. Soc. Rev., 1981, 10, 49–82 RSC.
- J. White and A. J. Bard, J. Electroanal. Chem. Interfacial Electrochem., 1986, 197, 233–244 CrossRef CAS.
- M. Passon, A. Ruff, P. Schuler, B. Speiser and I. Dreiling, ChemElectroChem, 2014, 1, 263–280 CrossRef.
- A. Ruff, B. Speiser and I. Dreiling, J. Electroanal. Chem., 2013, 710, 10–16 CrossRef CAS.
- A. Ruff, P. Schuler and B. Speiser, J. Solid State Electrochem., 2013, 17, 79–97 CrossRef CAS.
- W. Cheng, X.-F. Zhou and R. G. Compton, Angew. Chem., Int. Ed., 2013, 52, 12980–12982 CrossRef CAS PubMed.
- D. Horn and J. Rieger, Angew. Chem., Int. Ed., 2001, 40, 4330–4361 CrossRef CAS PubMed.
- S. V. Sokolov, S. Eloul, E. Kätelhön, C. Batchelor-McAuley and R. G. Compton, Phys. Chem. Chem. Phys., 2017, 19, 28–43 RSC.
- 5 mL of Nafion solution (5 wt%) was heated in water bath at less than 100 °C to condense it to 2 mL and condensed Nafion (12.5 wt%) was obtained.
- T. Watanabe and K. Honda, J. Phys. Chem., 1982, 86, 2617–2619 CrossRef CAS.
- J. H. Ross and R. I. Krieger, J. Agric. Food Chem., 1980, 28, 1026–1031 CrossRef CAS.
- J. Ellison, C. Batchelor-McAuley, K. Tschulik and R. G. Compton, Sens. Actuators, B, 2014, 200, 47–52 CrossRef CAS.
- K. Tschulik, B. Haddou, D. Omanović, N. V. Rees and R. G. Compton, Nano Res., 2013, 6, 836–841 CrossRef CAS.
- E. Kätelhön, A. Feng, W. Cheng, S. Eloul, C. Batchelor-McAuley and R. G. Compton, J. Phys. Chem. C, 2016, 120, 17029–17034 Search PubMed.
- H. G. Haubold, T. Vad, H. Jungbluth and P. Hiller, Electrochim. Acta, 2001, 46, 1559–1563 CrossRef CAS.
- T. Ohsaka, H. Yamamoto, M. Kaneko, A. Yamada, M. Nakamura, S. Nakamura and N. Oyama, Bull. Chem. Soc. Jpn., 1984, 57, 1844–1849 CrossRef CAS.
- L. L. C. Garcia, L. C. S. Figueiredo-Filho, G. G. Oliveira, O. Fatibello-Filho and C. E. Banks, Sens. Actuators, B, 2013, 181, 306–311 CrossRef CAS.
- A charge of 6.8 × 10−6 C is obtained at 0.005 V s−1, which is in the same magnitude with that at 0.1 V s−1, indicating that the charge transferred is not sensitive to the scan rate. This may be attributed to the complexity of the ensemble measurement due to the formation of the likely agglomerated and irregular ‘mat’.
-
A. J. Bard and L. R. Faulkner, Electrochemical Methods: Fundamentals and Applications, Wiley, 2000 Search PubMed.
- W. Cheng and R. G. Compton, Angew. Chem., Int. Ed., 2015, 54, 7082–7085 CrossRef CAS PubMed.
- X. Li, C. Batchelor-McAuley, S. A. I. Whitby, K. Tschulik, L. Shao and R. G. Compton, Angew. Chem., Int. Ed., 2016, 55, 4296–4299 CrossRef CAS PubMed.
- S. Park, H. Kim, J. Chae and J. Chang, J. Phys. Chem. C, 2016, 120, 3922–3928 CAS.
Footnote |
† Electronic supplementary information (ESI) available. See DOI: 10.1039/c7cp06764j |
|
This journal is © the Owner Societies 2018 |
Click here to see how this site uses Cookies. View our privacy policy here.