Solid–liquid equilibria for a pyrrolidinium-based common-cation ternary ionic liquid system, and for a pyridinium-based ternary reciprocal ionic liquid system: an experimental study and a thermodynamic model
Received
11th July 2017
, Accepted 8th November 2017
First published on 11th December 2017
Abstract
The present paper describes an experimental study and a thermodynamic model for the phase diagrams of the common-cation ternary system [C4MPyrr]Cl–[C4MPyrr]Br–[C4MPyrr]BF4 (where [C4MPyrr] refers to 1-butyl-1-methyl-pyrrolidinium) and of the ternary reciprocal system [C2Py], [C4Py]‖Cl, Br (where [CnPy] refers to 1-alkyl-pyridinium). Phase equilibria were measured by Differential Scanning Calorimetry (DSC) for two isoplethal sections in the common-cation pyrrolidinium-based ternary system. Phase diagram measurements were recently performed for the four common-ion binary subsystems and the two diagonal sections in the pyridinium-based ternary reciprocal system. In each case, the Modified Quasichemical Model was used to model the liquid solution, and the Compound Energy Formalism was used for the relevant solid solutions. For the ternary reciprocal system, the missing thermodynamic properties of the pure compounds were assessed using the Volume-based Thermodynamics (VBT) from Glasser and Jenkins, making it possible to estimate the exchange Gibbs free energy for the reaction [C2Py]Br (liquid) + [C4Py]Cl (liquid) = [C2Py]Cl (liquid) + [C4Py]Br (liquid). The experimental diagonal sections [C4Py]Br–[C2Py]Cl and [C4Py]Cl–[C2Py]Br were satisfactorily reproduced using solely the optimized model parameters for the four common-ion binary subsystems.
1. Introduction
Ionic liquids (ILs) are defined as a class of salts generally composed of a large asymmetric organic cation and an organic or inorganic anion,1 with a melting temperature below 100 °C.2 They possess unique physico-chemical properties such as a very low vapor pressure (i.e. a low volatility), and a high chemical and thermal stability over a broad temperature range (−40 to 200 °C).3 Over the recent years, ionic liquids have been considered for use in a variety of fields including analytics,4 chemical processing,5 solvents and catalysts,6 electrochemistry,7 biomass processing,8etc. As reported by Stark and Seddon,9 at a zeroth order approximation, the cation of an ionic liquid controls its physical properties (including density, viscosity, and temperature of fusion), while the anion governs the chemistry and reactivity. Therefore, fine-tuning the properties of ionic liquids may be required to target a specific application.
Over the past several years, a large and growing body of studies has focused on the design of single ionic liquids with “tailor-made” properties. A possible technique to modify the properties of ionic liquids is to add other substances such as molecular solvents, that are broadly accessible and whose properties have been widely investigated. Even though molecular solvents may decrease the viscosity of ionic liquids, they can lead to an increased volatility and consequently to a reduced thermal stability. The combination of single ionic liquids (IL–IL mixtures) may be an interesting alternative. Throughout this paper, the term “ionic liquid mixtures/systems” will refer to the combination of one single ionic liquid with one or more ionic liquids.
So far, there have been relatively few studies dealing with ionic liquid mixtures. Niedermeyer et al.10 performed a comprehensive review of the mixtures of ionic liquids. These authors investigated thermodynamics, physical and chemical properties, and applications of ionic liquid mixtures. To the best of our knowledge, ternary ionic liquid mixtures have received very little attention until now, thus motivating the present study. As pointed out by Plechkova and Seddon,11 ionic liquid ternary mixtures may be worth considering, where the chemistry of the system would be controlled by the first component, the physical properties (such as density and viscosity) of the system would be adjusted by the second component, and the global cost of the system would be lowered (through a dilution of the other two components) by adding a third component, cheap and chemically inert. The present article describes an experimental study and a thermodynamic model for the phase diagrams of the common-cation ternary system [C4MPyrr]Cl–[C4MPyrr]Br–[C4MPyrr]BF4 (where [C4MPyrr] refers to 1-butyl-1-methyl-pyrrolidinium) and of the ternary reciprocal system [C2Py]Cl–[C2Py]Br–[C4Py]Cl–[C4Py]Br (where [CnPy] refers to 1-alkyl-pyridinium) (a ternary reciprocal system is composed of two cations and two anions.). In particular, the minimum liquidus temperature in these two multicomponent ionic liquid systems can be calculated. As part of the present work, a literature review of the phase diagram measurements for ionic liquid systems was conducted (see Tables 1–3). Table 1 gives an overview of the phase diagram measurements for ionic liquid systems with organic cations. These are almost exclusively binary common-ion (common-cation or common-anion) systems, and the main experimental technique used was Differential Scanning Calorimetry (DSC). As seen in Table 1, most of these systems are simple eutectic systems; three systems display a eutectic behavior with two terminal solid solutions ([C3MPyrr][NTf2]–[C3MPyrr][FSI], [C4MPyrr][N(C4F9SO2)2]–[C4MPyrr][C4F9SO3], and [C2MPyrr][N(C4F9SO2)2]–[C2MPyrr][CF3SO3]); two systems exhibit an extensive solid solution over the entire composition range ([C3MPyrr][PF6]–[C3MPip][PF6] and [C2MPyrr][N(C4F9SO2)2]–[C4MPyrr][N(C4F9SO2)2]); and one system involves the formation of an intermediate compound ([N1112(OH)][C4F9SO3]–[C4MPyrr][C4F9SO3]). From the thermodynamic modeling viewpoint, Kick et al.12 modeled the phase diagram of the [C2MIm]Cl–[C4MIm]Cl common-anion binary system using the Schröder–Van Laar equation in its simplest form assuming an ideal liquid. Maximo et al.13 modeled the phase diagrams of nine [PF6]-based binary ionic liquid systems. These authors reported that most of the binary systems investigated are simple eutectic systems with a liquid close to ideal, while one particular binary system ([C3MPyrr][PF6]–[C3MPip][PF6]) displays an extensive solid solution confirmed by powder X-ray diffractometry of the solid phase. Recently, Teles et al.14 measured the phase diagrams of four binary systems of fluorinated ionic liquids, and modeled three of them. They reported a quasi-ideal eutectic behavior with the presence of two terminal solid solutions for the [C4MPyrr][N(C4F9SO2)2]–[C4MPyrr][C4F9SO3] and [C2MPyrr][N(C4F9SO2)2]–[C2MPyrr][CF3SO3] binary systems. Also, these authors observed a continuous solid solution behavior (confirmed by a X-ray diffraction study of the solid phase) for the [C2MPyrr][N(C4F9SO2)2]–[C4MPyrr][N(C4F9SO2)2] binary common-anion system. Robelin15 modeled the thermodynamic properties (including the phase diagram) of a binary system consisting of one ionic liquid ([C2MIm]Cl) and one inorganic compound (AlCl3) using the Modified Quasichemical Model. Using experimental data from ref. 16, the author calculated two binary eutectics (Teu1 = 231 K, Xeu1 = 40.1 mol% AlCl3; Teu2 = 166 K, Xeu2 = 66.2 mol% AlCl3) along with the formation of the intermediate compound [C2MIm]AlCl4. Robelin15 reported that this system exhibits extensive short-range ordering owing to the size and charge differences between the inorganic Al3+ and organic [C2MIm]+ cations.
Table 1 Literature review of the phase diagram measurements for ionic liquid systems with organic cations (Tfusion: temperature of fusion; Tg: glass transition temperature; Teu: eutectic temperature; Xeu: eutectic liquid composition)
System |
Component 1 (Tfusion [K]) |
Component 2 (Tfusion [K]) |
Type of phase diagram |
Ref. |
Ref. 52. Cations: [CnMIm]: 1-alkyl-3-methylimidazolium; [C2Pz]: 1-ethyl-2-methylpyrazolium; [CnMPyrr]: 1-alkyl-1-methylpyrrolidinium; [P6,6,6,14]: trihexyl(tetradecyl)phosphonium; [C3MPy]: 1-propyl-3-methylpyridinium; [C1Py]: 1-methylpyridinium; [C3MPip]: 1-propyl-1-methyl-piperidinium; [N4444]: tetrabutylammonium; [P4444]: tetrabutylphosphonium; [1,3-diMeIm]: 1,3-dimethylimidazolium; [N1112(OH)]: 2-(hydroxyethyl)trimethylammonium; [PPh4]: tetraphenylphosphonium; [HEA]: 2-hydroxyethylammonium. Anions: [NTf2]: bis(trifluoromethylsulfonyl)imide; [4,5-diNO2-Im]: 4,5-dinitroimidazolate; [4-NO2-Tri]: 4-nitro-1,2,3-triazolate; [dCa]: dicyanamide; [FSI]: bis(fluorosulfonyl)imide; [N(C4F9SO2)2]: bis(nonafluorobutylsulfonyl)imide; [C4F9SO3]: nonafluorobutanesulfonate; [CF3SO3]: trifluoromethanesulfonate; [HCOO]: formate; [TFSI]: bis(trifluoromethanesulfonyl)imide. |
Common anion |
[C2MIm][PF6] (334) |
[C2Pz][PF6] (352) |
Simple eutectic (Xeu = 47 mol% [C2MIm][PF6]; Teu = 296.15 K) |
42
|
[C3MPyrr][NTf2] (282) |
[C4MPyrr][NTf2] (256) |
Simple eutectic (Xeu = 50 mol% [C4MPyrr][NTf2]; Teu = 248.15 K) |
43
|
[C3MPyrr][NTf2] (282) |
[C6MPyrr][NTf2] (274) |
Not specified (measurements performed at a few compositions) |
[C3MPyrr][NTf2] (282) |
[C2MIm][NTf2] (274) |
Not specified (measurements performed at a few compositions) |
[C3MPyrr][NTf2] (282) |
[P6,6,6,14][NTf2] (Tg = 198) |
Not specified (measurements performed at a few compositions) |
[C2MIm][Cl] (361) |
[C4MIm][Cl] (342) |
Simple eutectic (Xeu = 50 mol% [C2MIm][Cl]; Teu = 315 K) |
12
|
[C2MIm][Cl] (361) |
[C4MIm][Cl] (338) |
Simple eutectic (Xeu = 51.3 mol% [C2MIm][Cl]; Teu = 319 K) |
44
|
[C3MIm][PF6] (312) |
[C3MPy][PF6] (312) |
Simple eutectic |
13
|
[C3MIm][PF6] (312) |
[C3MPyrr][PF6] (383) |
Simple eutectic |
[C3MIm][PF6] (312) |
[C3MPip][PF6] (368) |
Simple eutectic |
[C3MPy][PF6] (312) |
[C3MPip][PF6] (368) |
Simple eutectic |
[C3MPy][PF6] (312) |
[C3MPyrr][PF6] (383) |
Simple eutectic |
[C3MPyrr][PF6] (383) |
[C3MPip][PF6] (368) |
Complete solid solution |
[C3MIm][PF6] (312) |
[C12MIm][PF6] (326) |
Simple eutectic |
[C3MIm][PF6] (312) |
[N4444][PF6] (520) |
Simple eutectic |
[C3MIm][PF6] (312) |
[P4444][PF6] (497) |
Simple eutectic |
[C1Py][NTf2] (317) |
[C1MPyrr][NTf2] (—) |
Simple eutectic (Xeu = 30 mol% [C1MPyrr][NTf2]; Teu = 306.15 K) |
45
|
[C2MPyrr][N(C4F9SO2)2] (431) |
[C4MPyrr][N(C4F9SO2)2] (374) |
Complete solid solution |
14
|
[N1112(OH)][C4F9SO3] (449) |
[C4MPyrr][C4F9SO3] (361) |
Formation of an intermediate compound at 33 mol% [C4MPyrr][C4F9SO3] |
[PPh4][NTf2] (407) |
Cs[NTf2] (398) |
Simple eutectic (Xeu = 32.0 mol% [PPh4][NTf2]; Teu = 371.75 K) |
46
|
[C3MPyrr][FSI] (264) |
[C4MPyrr][FSI] (255) |
Not specified (measurements performed only at equimolar composition) |
47
|
[C3MPyrr][TFSI] (283) |
[C4MPyrr][TFSI] (266) |
|
Common cation |
[C2MIm][PF6] (335) |
[C2MIm][Cl] (361) |
Simple eutectic (Xeu = 37.6 mol% [C2MIm][Cl]; Teu = 312 K) |
44
|
[C2MIm][PF6] (335) |
[C2MIm][NO3] (316) |
Simple eutectic (Xeu = 60.0 mol% [C2MIm][NO3]; Teu = 292 K) |
[1,3-diMeIm][4,5-diNO2-Im] (372) |
[1,3-diMeIm][4-NO2-Tri] (359) |
Simple eutectic (Xeu = 64 mol% [1,3-diMeIm][4-NO2-Tri]; Teu = 339.65 K) |
48
|
[C3MPyrr][NTf2] (282) |
[C3MPyrr][dCa] (256) |
Simple eutectic (Xeu = 75 mol% [C3MPyrr][dCa]; Teu = 246.15 K) |
43
|
[C3MPyrr][NTf2] (285)a |
[C3MPyrr][FSI] (—) |
Eutectic behavior (Xeu = 70 mol% [C3MPyrr][FSI]; Teu = 247.15 K) with two terminal solid solutions |
49
|
[C4MPyrr][Cl] (474) |
[C4MPyrr][Br] (477) |
Simple eutectic (Xeu = 35.3 mol% [C4MPyrr][Br]; Teu = 464 K) |
50
|
[C4MPyrr][Cl] (474) |
[C4MPyrr][BF4] (425) |
Simple eutectic (Xeu = 47.4 mol% [C4MPyrr][BF4]; Teu = 366 K) |
[C4MPyrr][Br] (477) |
[C4MPyrr][BF4] (425) |
Simple eutectic (Xeu = 55.6 mol% [C4MPyrr][BF4]; Teu = 394 K) |
[C2MPyrr][NTf2] (364) |
[C2MPyrr][BF4] (339) |
Simple eutectic (Xeu = 40.1 mol% [C2MPyrr][BF4]; Teu = 322 K) |
[C4MPyrr][N(C4F9SO2)2] (374) |
[C4MPyrr][C4F9SO3] (361) |
Eutectic behavior (Xeu = 48.0 mol% [C4MPyrr][N(C4F9SO2)2]; Teu = 305 K) with two terminal solid solutions |
14
|
[C2MPyrr][N(C4F9SO2)2] (431) |
[C2MPyrr][CF3SO3] (384) |
Eutectic behavior (Xeu = 38.0 mol% [C2MPyrr][N(C4F9SO2)2]; Teu = 357 K) with two terminal solid solutions |
[C3MPyrr][FSI] (264) |
[C3MPyrr][TFSI] (283) |
Not specified (measurements performed only at equimolar composition) |
47
|
[C4MPyrr][FSI] (255) |
[C4MPyrr][TFSI] (266) |
|
Ternary reciprocal |
[C2MPyrr][NTf2] (364) |
[C4MPyrr][BF4] (425) |
Simple eutectic (Xeu = 50.1 mol% [C4MPyrr][BF4]; Teu = 274 K) |
50
|
[C4MIm][Cl] (338) |
[HEA][HCOO] (185) |
Simple eutectic |
51
|
[C3MPyrr][TFSI] (283) |
[C4MPyrr][FSI] (255) |
Not specified (measurements performed only at equimolar composition) |
47
|
[C3MPyrr][FSI] (264) |
[C4MPyrr][TFSI] (266) |
Table 2 Literature review of the phase diagram measurements for common-anion binary systems of salts with ions commonly used for the preparation of ionic liquids (Tfusion: temperature of fusion; Teu: eutectic temperature; Xeu: eutectic liquid composition)
Component 1 (Tfusion [K]) |
Component 2 (Tfusion [K]) |
Type of phase diagram |
Ref. |
Anions: [NTf2]: bis(trifluoromethylsulfonyl)imide; [FSA]: bis(fluorosulfonyl)amide. |
Li[NTf2] (506) |
Na[NTf2] (530) |
Simple eutectic (Xeu = 67.0 mol% Li[NTf2]; Teu = 453 K) |
53
|
Na[NTf2] (530) |
Rb[NTf2] (450) |
Simple eutectic (Xeu = 25.0 mol% Na[NTf2]; Teu = 431 K) |
K[NTf2] (472) |
Cs[NTf2] (395) |
Simple eutectic (Xeu: not specified; Teu = 395 K) |
Li[NTf2] (506) |
Rb[NTf2] (450) |
Two eutectic reactions (Xeu1 = 25.0 mol% Li[NTf2] and Teu1 = 421 K; Xeu2 = 60.0 mol% Li[NTf2] and Teu2 = 426 K) with formation of the intermediate compound LiRb(NTf2)2 |
Li[NTf2] (506) |
Cs[NTf2] (395) |
Two eutectic reactions (Xeu1 = 7.0 mol% Li[NTf2] and Teu1 = 385 K; Xeu2 = 60.0 mol% Li[NTf2] and Teu2 = 432 K) with formation of the intermediate compound LiCs(NTf2)2 |
Li[NTf2] (506) |
K[NTf2] (472) |
Eutectic reaction (Xeu = 43.0 mol% Li[NTf2]; Teu = 423 K) with formation of the intermediate compound Li3K(NTf2)4 |
Na[NTf2] (530) |
K[NTf2] (472) |
Eutectic reaction (Xeu = 25.0 mol% Na[NTf2]; Teu = 456 K) with formation of the intermediate compound Na3K(NTf2)4 |
Na[NTf2] (530) |
Cs[NTf2] (395) |
Eutectic reaction (Xeu = 7.0 mol% Na[NTf2]; Teu = 383 K) with formation of the intermediate compound Na3Cs(NTf2)4 |
K[NTf2] (472) |
Rb[NTf2] (450) |
Complete solid solution |
Rb[NTf2] (450) |
Cs[NTf2] (395) |
Complete solid solution |
|
Li[FSA] (403) |
K[FSA] (369) |
Simple eutectic (Xeu = 45.0 mol% Li[FSA]; Teu = 338 K) |
17
|
Li[FSA] (403) |
Cs[FSA] (386) |
Simple eutectic (Xeu = 40.0 mol% Li[FSA]; Teu = 331 K) |
K[FSA] (369) |
Cs[FSA] (386) |
Simple eutectic (Xeu = 60.0 mol% K[FSA]; Teu = 333 K) |
Table 3 Literature review of the phase diagram measurements for common-anion ternary systems of salts with ions commonly used for the preparation of ionic liquids (Teu: eutectic temperature; Tp: peritectic temperature)
Ternary system |
Type of phase diagram |
Ref. |
Anions: [NTf2]: bis(trifluoromethylsulfonyl)imide; [FSA]: bis(fluorosulfonyl)amide. |
Li[NTf2]–Na[NTf2]–K[NTf2] |
One ternary eutectic point (Teu = 427 K with XLi[NTf2] = 0.45, XNa[NTf2] = 0.10, XK[NTf2] = 0.45) and one ternary peritectic point (Tp = 431 K with XLi[NTf2] = 0.50, XNa[NTf2] = 0.10, XK[NTf2] = 0.40) |
54
|
Li[NTf2]–Na[NTf2]–Cs[NTf2] |
Two ternary eutectic points (Teu1 = 431 K with XLi[NTf2] = 0.60, XNa[NTf2] = 0.10, XCs[NTf2] = 0.30; Teu2 = 388 K with XLi[NTf2] = 0.05, XNa[NTf2] = 0.05, XCs[NTf2] = 0.90) |
Li[NTf2]–K[NTf2]–Cs[NTf2] |
Two ternary peritectic points (Tp1 = 421 K with XLi[NTf2] = 0.50, XK[NTf2] = 0.25, XCs[NTf2] = 0.25; Tp2 = 417 K with XLi[NTf2] = 0.30, XK[NTf2] = 0.40, XCs[NTf2] = 0.30) |
Na[NTf2]–K[NTf2]–Cs[NTf2] |
No ternary invariant point reported |
|
Li[FSA]–Na[FSA]–K[FSA] |
One ternary eutectic point (Teu = 318 K with XLi[FSA] = 0.30, XNa[FSA] = 0.40, XK[FSA] = 0.30) |
18
|
Li[FSA]–Na[FSA]–Cs[FSA] |
One ternary eutectic point (Teu = 311 K with XLi[FSA] = 0.30, XNa[FSA] = 0.40, XCs[FSA] = 0.30) |
Na[FSA]–K[FSA]–Cs[FSA] |
One ternary eutectic point (Teu = 309 K with XNa[FSA] = 0.40, XK[FSA] = 0.25, XCs[FSA] = 0.35) |
|
Li[FSA]–K[FSA]–Cs[FSA] |
One ternary eutectic point (ref. 18: Teu = 312 K with XLi[FSA] = 0.30, XK[FSA] = 0.35, XCs[FSA] = 0.35; ref. 17: Teu = 309 K with XLi[FSA] = 0.33, XK[FSA] = 0.33, XCs[FSA] = 0.33) |
17 and 18
|
The phase diagrams of binary systems of salts with ions commonly used for the preparation of ionic liquids have also been investigated (see Table 2). These are exclusively common-anion systems. These systems may be simple eutectic systems or may exhibit an intermediate compound or an extensive solid solution over the entire composition range. Robelin15 modeled the phase diagrams of three binary systems consisting of alkali bis(fluorosulfonyl)amides M[FSA] (with M = Li, K, Cs) using the experimental data reported in ref. 17.
Finally, an overview of the phase diagram measurements available in the literature for ternary systems of salts with ions commonly used for the preparation of ionic liquids is given in Table 3. These are common-anion ternary systems with different alkali metals. Studies of ionic liquid ternary systems involving organic cations are still lacking. Robelin15 modeled the phase diagram of the Li[FSA]–K[FSA]–Cs[FSA] system. This author calculated a ternary eutectic (Teu = 315 K with XLi[FSA] = 0.31, XK[FSA] = 0.33, XCs[FSA] = 0.36) very close to the experimental eutectic reported in the literature.17,18 In another study, Gbassi and Robelin19 modeled the phase diagram of the Li[CH3COO]–K[CH3COO]–Cs[CH3COO] system. Although the three pure salts have relatively high melting temperatures, a ternary eutectic was calculated at T = 368 K.
In the present work, the liquid phase of the two multicomponent ionic liquid systems investigated was modeled with the Modified Quasichemical Model for short-range ordering.20–22 This model has been applied successfully to various inorganic salt systems such as the NaF–AlF3–CaF2–Al2O3 base electrolyte23 (used to produce aluminum) and fertilizer systems.24,25 The liquid model considers the distribution of cations and anions on the sites of two different sublattices, and each ion occupies exactly one site. As mentioned previously,15 this model may have to be modified since the cations in ionic liquid systems are large and may involve long alkyl chains. In particular, it was shown15 that the current thermodynamic model would fail to reproduce the experimental [C2MIm]AlCl4–NaAlCl4 section in the NaCl–[C2MIm]Cl–AlCl3 phase diagram due to the large size difference between the inorganic Al3+ and organic [C2MIm]+ cations (where [C2MIm] refers to 1-ethyl-3-methyl-imidazolium). A large ion such as [C2MIm]+ can occupy several sites on the cationic sublattice. Also, the Modified Quasichemical Model does not yet take into account specific short-range interactions such as hydrogen bonding, van der Waals and π–π stacking interactions.15 Despite the fact that coulombic forces are the dominating interaction between the cations and anions of ionic liquids, relatively weak interactions such as hydrogen bonding may affect their physicochemical properties such as melting point, viscosity, etc.26 Previous studies have provided evidence for the existence of hydrogen bonds in ionic liquids (mainly imidazolium-based).27–31 In particular, it has been found that pyrrolidinium- and pyridinium-based ionic liquids exhibit weaker hydrogen bonding interactions than imidazolium-based compounds.32 This is due to the fact that the interaction between an anion and a H atom (C–H) on the imidazolium ring makes a stronger H-bond than that between an anion and a H atom (N–CH3) on a non-imidazolium cation.
As explained in Section 5.1, to model the phase diagram of a common-ion system with no existing intermediate compounds such as [C4MPyrr]Cl–[C4MPyrr]Br–[C4MPyrr]BF4, only the molar Gibbs free energies of fusion and the molar Gibbs free energies of solid–solid transition (if different allotropes exist) of the pure compounds need to be known. On the other hand, for a ternary reciprocal system such as [C2Py], [C4Py]‖Cl, Br, the standard thermodynamic properties (
,
, and Cp(T)) of all four pure compounds must be known or estimated, so that the Gibbs free energy change for the exchange reaction [C2Py]Br (liquid) + [C4Py]Cl (liquid) = [C2Py]Cl (liquid) + [C4Py]Br (liquid) can be assessed. Thermodynamic tables such as JANAF33 and Barin34 provide recommended values of the standard thermodynamic properties of numerous inorganic compounds. However, no such compilation tables are currently available for ionic liquid compounds. Therefore, methods of estimation such as the Volume-based Thermodynamics (VBT) from Glasser and Jenkins35–41 need to be used to estimate the missing thermodynamic data for the pure ionic liquid compounds.
The present paper is organised as follows: Section 2 describes the experimental procedure for the preparation of the various mixtures and for the phase diagram measurements by DSC in the [C4MPyrr]Cl–[C4MPyrr]Br–[C4MPyrr]BF4 ternary system. Sections 3 and 4 are devoted to the thermodynamic models used for the liquid phase and the relevant solid solutions, respectively. Section 5 presents the thermodynamic model developed for the common-cation ternary system [C4MPyrr]Cl–[C4MPyrr]Br–[C4MPyrr]BF4. Section 6 briefly describes the principles of the Volume-based Thermodynamics (VBT) with focus on the pyridinium-based compounds of the ternary reciprocal system [C2Py], [C4Py]‖Cl, Br. Finally, Section 7 is devoted to the thermodynamic model developed for the latter reciprocal system.
2. Materials and methods
2.1 Materials
All used ionic liquids were purchased from Iolitec GmbH, with a nominal purity of 99%, were recrystallized from an acetonitrile/ethyl acetate (1
:
10) mixture and dried under high vacuum (p = 0.001 mbar) in order to eliminate the influence of water on the melting point analysis. The water content of the samples was determined by Karl–Fischer titration using a Metrohm 915 KF Ti-Touch instrument with volumetric titration, and kept below 1000 ppm.
2.2 Preparation of ionic liquid mixtures for DSC analysis
In the case of hygroscopic salts such as pyrrolidinium halides, the procedure for sample preparation for DSC analysis needed to be performed in a glove box, under an argon atmosphere with highly reduced humidity. In order to prepare the ternary mixtures of ionic liquids at the different concentrations of each salt, each sample was prepared by weighing out the appropriate amount of each salt and then mixing it together in a vial. Subsequently, each mixture was heated up until complete melting of the solids, cooled down and ground after solidification. Approximately 6–18 mg of each sample was placed into a tarred aluminium DSC pan, and sealed using a lid of the same material, and submitted for DSC analysis.
2.3 DSC protocol
Thermal transitions were determined by performing DSC experiments using a Mettler-Toledo DSC 1 STARe System differential scanning calorimeter, cooled with a Huber TC100 immersion cooler. The calorimeter was calibrated for temperature and cell constants using high purity indium (melting temperature: 430 K; specific enthalpy of melting: 28.71 J g−1). All data were collected at atmospheric pressure, with nitrogen as a purge gas, and an empty sample pan as the reference. The experiments were conducted in the temperature range from 193.15 to 483.15 K. The samples were initially heated from room temperature, at a rate of 10 K min−1, to the highest temperature. At this temperature, they were held for a 10 min isotherm, prior to two cycles of cooling and heating at rates of 5 K min−1 spaced by 5 min isothermal holding at the lower and upper endpoint temperatures.
2.4 Transition temperature determination based on the peak position
The data from the DSC thermograms were obtained via analysis with the STARe Evaluation Software by Mettler Toledo. All temperatures reported for the glass transition and melting were established as the onset temperatures for the endothermic changes in heat flow. Uncertainties of 1 K in the temperatures and of 1% in the enthalpies are estimated.
3. Thermodynamic model for the liquid phase
The liquid phase of the [C2Py], [C4Py]‖Cl, Br ternary reciprocal system was modeled with the Modified Quasichemical Model in the Quadruplet Approximation (MQMQA)22 that evaluates coupled 1st- and 2nd-nearest-neighbor short-range order. This is a two-sublattice model, where the cations are assumed to distribute on one sublattice, and the anions are assumed to distribute on another sublattice. A quadruplet consists of two second-nearest-neighbor cations and two second-nearest-neighbor anions, which are mutual first-nearest-neighbors (see Fig. 1). Each quadruplet has a Gibbs free energy. Quadruplets mix randomly, constrained by an elemental mass balance. The equilibrium quadruplet composition (configuration) is the one that minimizes the Gibbs free energy of the melt at given temperature, pressure and composition, and thus results in an equilibrium configuration that reflects 1st- and 2nd-nearest-neighbor short-range order. The following second-nearest-neighbor pair exchange reactions are taken into account: | (A–X–A)pair + (B–X–B)pair = 2(A–X–B)pair; ΔgAB/X2 | (1) |
| (X–A–X)pair + (Y–A–Y)pair = 2(X–A–Y)pair; ΔgA2/XY | (2) |
where A and B are two different cations ([C2Py]+ and [C4Py]+ in the present case), and X and Y are two different anions (Cl− and Br− in the present case). As the Gibbs free energy change ΔgAB/X2(respectively ΔgA2/XY) becomes progressively more negative, reaction (1) (respectively (2)) is shifted to the right, (A–X–B) (respectively (X–A–Y)) pairs predominate and second-nearest-neighbor cation–cation (respectively anion–anion) short-range ordering results. The Gibbs free energy changes ΔgAB/X2 and ΔgA2/XY are model parameters that can be expressed as a function of composition by empirical polynomial expressions (see eqn (11) in ref. 21) in order to reproduce the available experimental data (phase diagram in the present case) for the A, B‖X and A‖X, Y binary subsystems, respectively. In order to obtain a quantitative fit for the A, B‖X, Y system, small empirical “ternary reciprocal parameters” may have to be included. As described previously,22 these parameters represent the Gibbs free energies of formation of the ABXY quadruplets from the binary quadruplets according to: |  | (3) |
However, such “ternary reciprocal parameters” were not required for the [C2Py], [C4Py]‖Cl, Br system. The extent of first-nearest-neighbor (cation–anion) short-range ordering is related to the Gibbs free energy change for the following exchange reaction: | AX + BY = AY + BX; ΔgexchangeAB/XY | (4) |
If ΔgexchangeAB/XY is negative, then A–Y and B–X first-nearest-neighbor pairs predominate. For instance, as explained in Section 7.1,
for the exchange reaction [C2Py]Br (liquid) + [C4Py]Cl (liquid) = [C2Py]Cl (liquid) + [C4Py]Br (liquid) can be estimated as −1.6 kJ mol−1 at 25 °C, −1.9 kJ mol−1 at 100 °C, and −2.4 kJ mol−1 at 150 °C (i.e. above the highest melting temperature of the four pure ionic liquids). These are relatively small values and thus the [C2Py], [C4Py]‖Cl, Br liquid displays relatively little first-nearest-neighbor (cation–anion) short-range ordering. As will be shown in Section 7.2, the optimized model parameters ΔgAB/X2 and ΔgA2/XY for the four common-ion binary subsystems are relatively small in amplitude. Therefore, the [C2Py], [C4Py]‖Cl, Br liquid also exhibits relatively little second-nearest-neighbor (cation–cation and anion–anion) short-range ordering. It can be concluded that the investigated ternary reciprocal liquid displays small deviations from ideality, which is due to the fact that the two cations only differ by the length of their alkyl chains (ethyl or butyl) and that the two anions are halides with similar ionic radii.
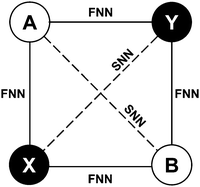 |
| Fig. 1 Example of a quadruplet (A, B: cations; X, Y: anions; FNN: first-nearest-neighbor; SNN: second-nearest-neighbor). | |
Values of the second-nearest-neighbor “coordination numbers” of the various ions in the various quadruplets have to be defined. Reasonable values are chosen in order to best reproduce the available phase diagram data. These should be regarded as model parameters which may differ from the actual physical coordination numbers. They were all set to 6 for the [C2Py], [C4Py]‖Cl, Br system. In the improved version of the MQMQA,55 the parameter ζA/X, which is equal to the number of quadruplets emanating from, or containing, a first-nearest-neighbor A–X pair (where A is a cation and X is an anion), is defined as:
|  | (5) |
where

is the second-nearest-neighbor “coordination number” of the ion i (i = A, X) when all i exist in A
2X
2 quadruplets. The resulting values of
ζA/X (
eqn (5)) for the [C
2Py], [C
4Py]‖Cl, Br system are all equal to 6.
For the liquid phase of the common-cation ternary system [C4MPyrr]Cl–[C4MPyrr]Br–[C4MPyrr]BF4, the Modified Quasichemical Model in the Pair Approximation (MQMPA)20,21 is sufficient. Since the cationic sublattice is occupied only by one type of cation ([C4MPyrr]+), only the second-nearest-neighbor anion–anion pairs on the anionic sublattice need to be taken into account, and ΔgA2/XY (eqn (2)) is the only relevant Gibbs free energy change. For the sake of clarity, this notation will be retained in the present article. (The notation ΔgA/XY was often used in previous publications.) All second-nearest-neighbor anion–anion “coordination numbers” were set to 6. The [C4MPyrr]Cl–[C4MPyrr]Br–[C4MPyrr]BF4 ternary system is considered to be “asymmetric”, with [C4MPyrr]BF4 as the “asymmetric” component. This asymmetry makes the estimation of the thermodynamic properties of the ternary liquid from binary optimized parameters very similar to the Kohler–Toop interpolation method.56 Under these conditions, the composition variables χXY, defined previously,21 that are relevant for the present work become:
| χCl(BF4) = χBr(BF4) = xClCl + xBrBr + xClBr | (6) |
| χ(BF4)Cl = χ(BF4)Br = x(BF4)(BF4) | (7) |
where
xXY is the mole fraction of second-nearest-neighbor (X–[C
4MPyrr]–Y) pairs. Note that, for the binary systems [C
4MPyrr]X–[C
4MPyrr]BF
4 (where X = Cl, Br),
eqn (6) reduces to:
As mentioned before, the parameters Δ
gA2/XY (with A = [C
4MPyrr]) of
reaction (2) for each anion–anion pair are expanded, through optimization with the available phase diagram data, as empirical polynomials in
χXY and
χYX. For the ternary system, terms may be added that give the effect of the third component on the pair-formation energies Δ
gA2/XY.
21 One ternary term was required for the [C
4MPyrr]Cl–[C
4MPyrr]Br–[C
4MPyrr]BF
4 system (see Section 5.3). As will be shown in Section 5.2, the optimized model parameters Δ
gA2/XY for the three binary subsystems are relatively small in amplitude. Therefore, the investigated common-cation ternary liquid displays relatively little second-nearest-neighbor (anion–anion) short-range ordering. Thus, the Bragg–Williams (random-mixing) model could have been used successfully for this liquid. This model is described in detail in
ref. 57 and is briefly presented in
ref. 15. The Modified Quasichemical Model (MQM) was finally preferred since it is suitable for liquids exhibiting either small or extensive short-range ordering, and also for liquids displaying positive deviations from ideality. As a result, the MQM is well adapted for the development of large thermodynamic databases.
58–60
4. Thermodynamic model for the solid solutions
The solid solutions relevant for the present work are: the Cl-rich [C4MPyrr](Cl,[Br]) solid solution, the Br-rich [C4MPyrr](Br,[Cl]) solid solution, the [C2Py]Cl–[C2Py]Br solid solution, and the [C4Py]Cl–[C4Py]Br solid solution. They were all modeled using the Compound Energy Formalism.61,62 Let us consider a solid solution phase such as B(Cl,Br), where B (B = [C4MPyrr]+, [C2Py]+ or [C4Py]+) resides on the cationic sublattice C, and Cl− and Br− reside on the anionic sublattice A. The molar Gibbs free energy of the solution is then given by the following equation: |  | (9) |
The first two terms represent the reference Gibbs free energy of the solution, where
and
are the site fractions of Cl− and Br− on the anionic sublattice A, and
and
are the standard molar Gibbs free energies of the end-member components BCl and BBr, respectively, possibly augmented by a temperature-dependent Gibbs free energy. This latter term is always positive and is a model parameter. For instance, for the Cl-rich [C4MPyrr](Cl,[Br]) high temperature solid solution, the two end-member compositions are [C4MPyrr]Cl(s2) and hypothetical [C4MPyrr]Br having the same crystal structure as [C4MPyrr]Cl(s2). The Gibbs free energy of this hypothetical [C4MPyrr]Br is that of [C4MPyrr]Br(s) augmented by a constant (and positive) Gibbs free energy. On the other hand, for the [C2Py]Cl–[C2Py]Br extensive solid solution, the two end-member compositions are [C2Py]Cl(s) and [C2Py]Br(s) (which have the same crystal structure). The third term in eqn (9) gives the ideal entropy of mixing (Temkin type63), assuming a random distribution of the anions on the anionic sublattice. The final term is the molar excess Gibbs free energy and is expressed as follows: |  | (10) |
The L factor may depend on the temperature and also on the composition, where Redlich–Kister terms as a function of site fractions are generally used.
5. The [C4MPyrr]Cl–[C4MPyrr]Br–[C4MPyrr]BF4 system
This section describes a thermodynamic model for the common-cation ternary system [C4MPyrr]Cl–[C4MPyrr]Br–[C4MPyrr]BF4. Fig. 2 shows the chemical structure of the three pure ionic liquids. The phase diagrams of the three binary subsystems were measured previously by DSC,50 and these data are used in the present work. As described in Section 5.3, two isoplethal sections were measured in the ternary system, and these new data are compared to the phase diagrams predicted by using solely the optimized model parameters for the three binary subsystems along with a standard asymmetric interpolation method.21 Then, one small ternary parameter is included for the liquid phase in order to best reproduce the ternary data.
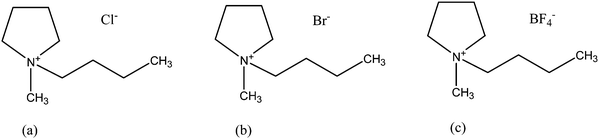 |
| Fig. 2 Chemical structure of the pyrrolidinium-based ionic liquids: (a) [C4MPyrr]Cl; (b) [C4MPyrr]Br; (c) [C4MPyrr]BF4. | |
5.1 Pure ionic liquids
To model the phase diagram of a common-ion (common-cation or common-anion) system for which measurements are available, only the molar Gibbs free energies of fusion and the molar Gibbs free energies of solid–solid transition (if different allotropes exist) of the pure compounds need to be known. The molar Gibbs free energy of fusion of the pure compound m may be written as: |  | (11) |
where the enthalpy of fusion
is independent of the temperature to a first approximation. Similarly, the molar Gibbs free energy of solid–solid transition for a compound displaying two different allotropes (s1, s2) may be written as: |  | (12) |
To our knowledge, few thermodynamic data are available in the literature for the three pure compounds [C4MPyrr]Cl, [C4MPyrr]Br and [C4MPyrr]BF4 (see Table 4). The enthalpy of fusion of [C4MPyrr]BF4 has not been measured, and it was assessed in the present work by using the limiting liquidus slope eqn (13), which assumes no solid solubility: |  | (13) |
where R is the gas constant, and Tfusion(m) and
are respectively the temperature of fusion and the molar enthalpy of fusion of the pure salt m. Eqn (13) was applied to the [C4MPyrr]Cl–[C4MPyrr]BF4, [C4MPyrr]Br–[C4MPyrr]BF4 and [C2MPyrr]NTf2–[C4MPyrr]BF4 experimental phase diagrams of Stolarska et al.50 (where [C2MPyrr] and NTf2 refer to 1-ethyl-1-methyl-pyrrolidinium and bis(trifluoromethylsulfonyl)imide, respectively). An average value of 13
725 J mol−1 was obtained for the enthalpy of fusion of [C4MPyrr]BF4. As shown in Table 4, experimental values of the enthalpy of fusion of [C4MPyrr]Cl and [C4MPyrr]Br were available and they are used directly in the present work. Finally, for the sake of consistency, the temperatures of fusion of the three pure compounds measured by Stolarska et al.50 were selected in the present study.
Table 4 Enthalpies of fusion and of solid–solid transition measured by DSC in the literature for the pure compounds [C4MPyrr]Cl, [C4MPyrr]Br and [C4MPyrr]BF4
Cation |
Anion |
Water content (ppm) |
Purity (%) |
Melting temperature (K) |
Enthalpy of fusion (J mol−1) |
Solid–solid transition |
Ref. |
[C4MPyrr]+ |
Cl− |
— |
>98 |
474 |
— |
— |
50
|
7–8 |
High |
476 |
13 037 |
T
trans = 466.45 K
ΔHtrans = 1493 J mol−1
|
64
|
Br− |
— |
>98 |
477 |
— |
— |
50
|
3540 |
— |
487 |
13 120 |
— |
65
|
BF4− |
— |
>98 |
425 |
— |
— |
50
|
5.2 Binary subsystems
5.2.1 The [C4MPyrr]Br–[C4MPyrr]BF4 binary system.
The phase diagram has been measured by DSC.50 No solid solubility was reported, and there are no reported intermediate compounds. The optimized Gibbs free energy of reaction (2) is: | Δg[C4MPyrr]2/Br(BF4)/(J mol−1) = 209.2 + 836.8χBr(BF4) + 1740.5χ(BF4)Br | (14) |
The calculated phase diagram is shown along with the measurements in Fig. 3. This is a simple eutectic system. The reported characteristics of the experimental eutectic are {T = 394 K (i.e. 120.85 °C), 55.6 mol% [C4MPyrr]BF4}.50 The characteristics of the calculated eutectic are {121 °C, 55.5 mol% [C4MPyrr]BF4}.
 |
| Fig. 3 Calculated [C4MPyrr]Br–[C4MPyrr]BF4 phase diagram, temperature versus mole fraction of [C4MPyrr]BF4. Experimental data are from Stolarska et al.50 (●) and from the present work (▲). | |
As shown in Fig. 3, a DSC signal for the eutectic reaction was observed only for three binary mixtures. As emphasized by Stolarska et al.,50 owing to the dynamic nature of the experimental technique, thermal transitions that should exist thermodynamically may be kinetically hindered. Therefore, measuring and interpreting the phase diagrams of ionic liquid systems is expected to be more difficult than for inorganic salt systems. The measured liquidus temperatures in Fig. 3 are somewhat scattered: for instance, at 10 mol% [C4MPyrr]BF4, the shift between the calculated and experimental liquidus temperatures is almost 10 °C. The scattering of the data is more visible in the eutectic temperatures measured for the seven binary mixtures in the [C4MPyrr]Cl–[C4MPyrr]BF4 binary system (see Fig. 4).
 |
| Fig. 4 Calculated [C4MPyrr]Cl–[C4MPyrr]BF4 phase diagram, temperature versus mole fraction of [C4MPyrr]BF4. Experimental data are from Stolarska et al.50 (●) and from the present work (▲). | |
5.2.2 The [C4MPyrr]Cl–[C4MPyrr]BF4 binary system.
The phase diagram has been measured by DSC.50 No solid solubility was reported and the measured limiting slope of the [C4MPyrr]Cl liquidus curve agrees with eqn (13). There are no reported intermediate compounds. The optimized Gibbs free energy of reaction (2) is: | Δg[C4MPyrr]2/Cl(BF4)/(J mol−1) = −669.4 − 836.8χCl(BF4) + 836.8χ(BF4)Cl | (15) |
The calculated phase diagram is compared with the measurements in Fig. 4. Again, this is a simple eutectic system. The reported characteristics of the experimental eutectic are {T = 366 K (i.e. 92.85 °C), 47.4 mol% [C4MPyrr]BF4}.50 The composition of the eutectic liquid was derived from a Tammann plot, where the specific heat of fusion of the eutectic peak was plotted against the composition.50 The characteristics of the calculated eutectic are {92 °C, 50.4 mol% [C4MPyrr]BF4}. As reported in Table 4, Babushkina64 measured by DSC a solid–solid transition at 193.3 °C for [C4MPyrr]Cl. Therefore, two different allotropes (s1, s2) were introduced for the latter compound in the present work.
5.2.3 The [C4MPyrr]Cl–[C4MPyrr]Br binary system.
The phase diagram has been measured by DSC.50 No solid solubility was reported, and there are no reported intermediate compounds. As shown in Fig. 5(a), the measured limiting slopes of the [C4MPyrr]Cl and [C4MPyrr]Br liquidus curves substantially disagree with eqn (13), and therefore this system exhibits at least some terminal solid solubility. Two thermal transitions were observed only for four binary mixtures. The low-temperature transition at about 190 °C was attributed to a binary eutectic.50 For pure [C4MPyrr]Cl and most of the [C4MPyrr]Cl–[C4MPyrr]Br binary mixtures investigated, another thermal transition was observed in the range 370–390 K (i.e. 96.85–116.85 °C)50 (see Fig. 5(b)). As suggested by Stolarska et al., this transition might be associated with [C4MPyrr]Cl polymorphism, with the existence of two preferred conformations of the pyrrolidinium ions. However, no such thermal transition was observed for the [C4MPyrr]Cl–[C4MPyrr]BF4 system.50 Finally, the low-temperature thermal transition reported above was not considered in the present work. Two terminal solid solutions were introduced: a Cl-rich [C4MPyrr](Cl,[Br]) solid solution and a Br-rich [C4MPyrr](Br,[Cl]) solid solution. The reported characteristics of the experimental eutectic are {T = 464 K (i.e. 190.85 °C), 35.3 mol% [C4MPyrr]Br}. The characteristics of the calculated eutectic are {192 °C, 35.3 mol% [C4MPyrr]Br}. The low-temperature transitions at about 190 °C measured for the binary mixtures containing 60.0 or 80.0 mol% [C4MPyrr]Br are not well reproduced by the model, but this shift may be due to the scattering of the data. Since the Cl− and Br− anions have similar ionic radii,66 the binary liquid was assumed to be ideal. That is: | Δg[C4MPyrr]2/ClBr = 0 | (16) |
The two terminal solid solutions were modeled with the Compound Energy Formalism (CEF). Their optimized Gibbs free energies are given by: |  | (17) |
|  | (18) |
Recently, the [C4MPyrr][C4F9SO3]–[C4MPyrr][N(C4F9SO2)2] phase diagram has been measured by DSC, and it has been interpreted as displaying two terminal solid solutions.14 A similar assumption was made in the present work for the [C4MPyrr]Cl–[C4MPyrr]Br system, thus implying that [C4MPyrr]Cl(s2) and [C4MPyrr]Br do not have the same crystal structure. To our knowledge, no crystal structure information is available. If there was convincing evidence that these two solid compounds have the same crystal structure, then a single solid solution would have to be introduced and the experimental low-temperature transition at about 190 °C would be due to a very flat minimum (azeotrope) or a solid–solid miscibility gap. The introduction of two terminal solid solutions allowed us to best reproduce the experimental phase diagram, in particular the liquidus temperatures which are of primary interest in the present study.
 |
| Fig. 5 (a) Calculated [C4MPyrr]Cl–[C4MPyrr]Br phase diagram, temperature versus mole fraction of [C4MPyrr]Br. Experimental data are from Stolarska et al.50 (●) and from the present work (▲). Notations: A: [C4MPyrr](Cl,[Br])(ss), B: [C4MPyrr](Br,[Cl])(ss). The limiting liquidus slopes calculated from eqn (13) are shown as thin red lines. (b) Calculated [C4MPyrr]Cl–[C4MPyrr]Br phase diagram over a wider temperature range. Experimental data are from Stolarska et al.50 (●, ■) and from the present work (▲). | |
5.3 The [C4MPyrr]Cl–[C4MPyrr]Br–[C4MPyrr]BF4 ternary system
The calculated liquidus projection of the [C4MPyrr]Cl–[C4MPyrr]Br–[C4MPyrr]BF4 system is shown in Fig. 6. As explained in Section 3, the thermodynamic properties of the ternary liquid are calculated from the optimized model parameters for the three binary subsystems (eqn (14)–(16)) using an asymmetric interpolation method. As justified below, a small ternary excess parameter was included for the liquid phase: |  | (19) |
In Fig. 6, the univariant lines (along which the liquid coexists with two solid phases) are shown as bold lines. By convention, the arrows indicate the directions of decreasing temperature along these lines. The constant temperature lines are called liquidus isotherms, and they are displayed as thin lines. Two ternary invariant reactions are calculated. The ternary eutectic reaction liquid = [C4MPyrr]Cl(s1) + [C4MPyrr]BF4 + [C4MPyrr](Cl,[Br])(ss) occurs at 91 °C with a liquid composition of (49.5 mol% [C4MPyrr]BF4 + 49.0 mol% [C4MPyrr]Cl + 1.5 mol% [C4MPyrr]Br). This corresponds to the minimum liquidus temperature in the ternary system. The ternary quasi-peritectic reaction liquid + [C4MPyrr](Br,[Cl])(ss) = [C4MPyrr](Cl,[Br])(ss) + [C4MPyrr]BF4 occurs at 98 °C with a liquid composition of (52.2 mol% [C4MPyrr]BF4 + 41.9 mol% [C4MPyrr]Cl + 5.9 mol% [C4MPyrr]Br).
 |
| Fig. 6 Calculated liquidus projection of the [C4MPyrr]Cl–[C4MPyrr]Br–[C4MPyrr]BF4 system. | |
In order to test the accuracy of the developed thermodynamic model, two isoplethal sections were measured by DSC: the isoplethal section at constant 50 mol% [C4MPyrr]BF4 and the isoplethal section at a constant molar ratio [C4MPyrr]Cl/([C4MPyrr]Cl + [C4MPyrr]Br) of 0.85. As an example, DSC thermograms for the latter isoplethal section are shown in Fig. 7. The data and the calculations are compared in Fig. 8 and 9. A small ternary excess parameter (eqn (19)) was introduced for the liquid in order to best reproduce the measurements. The calculations in the presence and absence of the ternary excess parameter are compared for both isoplethal sections in Fig. 10 and 11. As explained previously, thermal transitions that should exist thermodynamically may not be observed by DSC. Therefore, for a given sample, the number of calculated transitions should be equal to or higher than the number of experimental transitions. Both situations occur in the isoplethal section shown in Fig. 8. For both of the investigated isoplethal sections, agreement between the calculations and the measurements is satisfactory (Fig. 8 and 9). The observed temperature shift is always less than 12 °C.
 |
| Fig. 7 DSC thermograms of the common-cation ternary system [C4MPyrr]Cl–[C4MPyrr]Br–[C4MPyrr]BF4, for the isoplethal section at a constant molar ratio [C4MPyrr]Cl/([C4MPyrr]Cl + [C4MPyrr]Br) of 0.85. Mole fraction of [C4MPyrr]BF4 in the ternary mixture: (1) 0.00; (2) 0.25; (3) 0.50; (4) 0.75; (5) 0.90; (6) 1.00. | |
 |
| Fig. 8 Calculated section of the [C4MPyrr]Cl–[C4MPyrr]Br–[C4MPyrr]BF4 phase diagram at constant 50.0 mol% [C4MPyrr]BF4. New DSC measurements (●); Stolarska et al.50 (▲). Notations: A: [C4MPyrr]BF4(s), B: [C4MPyrr]Cl(s1), C: [C4MPyrr](Cl,[Br])(ss), D: [C4MPyrr](Br,[Cl])(ss). | |
 |
| Fig. 9 Calculated section of the [C4MPyrr]Cl–[C4MPyrr]Br–[C4MPyrr]BF4 phase diagram at a constant molar ratio [C4MPyrr]Cl/([C4MPyrr]Cl + [C4MPyrr]Br) of 0.85. New DSC measurements (●); Stolarska et al.50 (▲). Notations: A: [C4MPyrr]BF4(s), B: [C4MPyrr]Cl(s1), C: [C4MPyrr](Cl,[Br])(ss), D: [C4MPyrr](Br,[Cl])(ss). | |
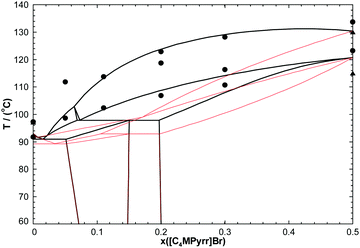 |
| Fig. 10 Calculated section of the [C4MPyrr]Cl–[C4MPyrr]Br–[C4MPyrr]BF4 phase diagram at constant 50.0 mol% [C4MPyrr]BF4, in the presence (thick lines) or in the absence (thin red lines) of the ternary excess parameter for the liquid phase (eqn (19)). | |
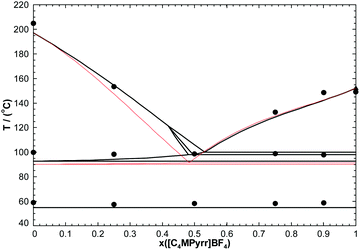 |
| Fig. 11 Calculated section of the [C4MPyrr]Cl–[C4MPyrr]Br–[C4MPyrr]BF4 phase diagram at a constant molar ratio [C4MPyrr]Cl/([C4MPyrr]Cl + [C4MPyrr]Br) of 0.85, in the presence (thick lines) or in the absence (thin red lines) of the ternary excess parameter for the liquid phase (eqn (19)). | |
6. Volume-based Thermodynamics (VBT)
Since no compilation tables exist currently for the thermodynamic properties (Cp(T),
,
) of pure ionic liquids, estimation techniques such as the Volume-Based Thermodynamics (VBT) from Glasser and Jenkins35–41 are needed to predict the missing thermodynamic data. (
is the standard molar enthalpy at 298.15 K, relative to the elements in their stable standard states at 298.15 K.) Recently, Robelin15 tested the applicability of the VBT to predict the thermodynamic properties of various methyl-imidazolium-based ILs. The VBT only takes into account long-range Coulombic interactions, and short-range interactions such as van der Waals and hydrogen bonding are not yet considered.41 The VBT requires a knowledge of the molecular volume νm (expressed in nm3). The latter can be derived from crystallographic data for the pure solid phases, and from density measurements (using a densitometer) for the pure liquid phases. The estimation of the relevant thermodynamic properties of a pure ionic liquid is described below.
6.1 Heat capacity Cp(T)
By analogy with Strechan et al.,67 who correlated the heat capacity (Cp) with the molecular volume (νm) at 298.15 K for various methyl-imidazolium-based ionic liquids, the experimental data (Cp and density) available for twenty one pyridinium-based ILs were collected from the literature (see Table 5). Only five of the ionic liquids (highlighted in Table 5) are 1-alkyl-pyridinium-based compounds and have a chemical structure very similar to those of the pyridinium-based ionic liquids studied in the present work. The experimental data available for these five compounds were used to derive the following linear regression equation: | Cp(298.15 K)/(J mol−1 K−1) = 1195.2νm + 16.8 | (20) |
where νm is the molecular volume (in nm3). The standard error of a prediction is ±18.4 J mol−1 K−1. Fig. 12 displays the corresponding linear regression line along with the linear regression line obtained from nineteen pyridinium-based compounds. For the latter, the [C2(4)MPy][NTf2] and [C8(3)MPy][BF4] compounds (shown as empty circles) were discarded.
Table 5 Calculated (eqn (20)) and experimental Cp values at T = 298.15 K for various pyridinium-based ionic liquids
Compound |
Measured Cp [J mol−1 K−1] at 298.15 K |
Measured density ρ [g cm−3] at 298.15 K |
ν
m [nm3] at 298.15 K |
Calculated Cp [J mol−1 K−1] at 298.15 K |
Relative shift for Cp (%) |
Ref. |
Cations: [C4(3)MPy]: 1-butyl-3-methylpyridinium; [C4(4)MPy]: 1-butyl-4-methylpyridinium; [C2Py]: 1-ethylpyridinium; [C3Py]: 1-propylpyridinium; [C2(2)C2Py]: 1,2-diethylpyridinium; [C1Py]: 1-methylpyridinium; [C4(3)CNPy]: 1-butyl-3-cyanopyridinium; [C6(3)CNPy]: 1-hexyl-3-cyanopyridinium; [C6(4)CNPy]: 1-hexyl-4-cyanopyridinium; [C8(3)CNPy]: 1-octyl-3-cyanopyridinium; [C6(3)MPy]: 1-hexyl-3-methylpyridinium; [C6Py]: 1-hexylpyridinium; [C4Py]: 1-butylpyridinium; [C2(4)MPy]: 1-ethyl-4-methylpyridinium; [C8(3)MPy]: 1-octyl-3-methylpyridinium. Anions: [C(CN)3]: tricyanomethanide; [NTf2]: bis(trifluoromethylsulfonyl)imide; [dCa]: dicyanamide; [EtSO4]: ethylsulfate; [MeSO4]: methylsulfate; [CF3SO3]: triflate. |
[C4(3)MPy][C(CN)3] |
468.0 |
1.042 |
0.383 |
474.6 |
1.4 |
69
|
[C4(4)MPy][NTf2] |
636.4 |
1.412 |
0.506 |
621.6 |
−2.3 |
70
|
[C4(3)MPy][BF4] |
412.3 |
1.183 |
0.333 |
414.8 |
0.6 |
71
|
[C4(4)MPy][BF4] |
413.7 |
1.183 |
0.333 |
414.8 |
0.3 |
71
|
[C
2
Py][NTf
2
]
|
522.4
|
1.536
|
0.420
|
518.8
|
−0.7
|
72
|
[C4(3)MPy][dCa] |
367.7 |
1.056 |
0.340 |
423.2 |
15.1 |
73
|
[C
3
Py][BF
4
]
|
363.0
|
1.253
|
0.277
|
347.9
|
−4.2
|
74
|
[C2(2)C2Py][EtSO4] |
412.0 |
1.219 |
0.356 |
442.3 |
7.4 |
75
|
[C
1
Py][MeSO
4
]
|
299.0
|
1.346
|
0.253
|
319.2
|
6.8
|
75
|
[C4(3)CNPy][NTf2] |
586.0 |
1.479 |
0.495 |
608.4 |
3.8 |
76
|
[C6(3)CNPy][NTf2] |
658.0 |
1.409 |
0.553 |
677.7 |
3.0 |
76
|
[C6(4)CNPy][NTf2] |
633.0 |
1.407 |
0.554 |
678.9 |
7.3 |
76
|
[C8(3)CNPy][NTf2] |
709.0 |
1.355 |
0.609 |
744.7 |
5.0 |
76
|
[C6(3)MPy][NTf2] |
634.0 |
1.362 |
0.559 |
684.9 |
8.0 |
77 and 78
|
[C6Py][NTf2] |
612.0 |
1.388 |
0.532 |
652.6 |
6.6 |
78
|
[C
4
Py][BF
4
]
|
395.2
|
1.213
|
0.305
|
381.3
|
−3.5
|
79
|
[C4(3)MPy][NTf2] |
622.0 |
1.415 |
0.505 |
620.4 |
−0.3 |
80 and 81
|
[C4(3)MPy][BF4] |
405.0 |
1.183 |
0.333 |
414.8 |
2.4 |
80 and 82
|
[C
4
Py][CF
3
SO
3
]
|
470.4
|
1.214
|
0.390
|
482.9
|
2.7
|
83
|
[C2(4)MPy][NTf2] |
630.5 |
1.487 |
0.449 |
553.4 |
−12.2 |
84 and 85
|
[C8(3)MPy][BF4] |
450.5 |
1.095 |
0.445 |
548.7 |
21.8 |
86
|
 |
| Fig. 12 Heat capacity (Cp) at 298.15 K as a function of molecular volume (νm) for various pyridinium-based ionic liquids. Experimental data for 1-alkyl-pyridinium-based compounds (▲), experimental data for other types of pyridinium-based compounds (●), discarded experimental data (○). Linear regression equation for compounds shown as ▲: Cp/(J mol−1 K−1) = 1195.2νm + 16.8 (full line). Linear regression equation for compounds shown as ▲ and ●: Cp/(J mol−1 K−1) = 1109.5νm + 37.1 (dashed line). | |
6.2 Standard absolute entropy (
)
Glasser38 proposed the following expression for the standard absolute entropy (
) of ionic liquids, which is an average of two linear regression equations (one valid for anhydrous ionic solids36 and another one valid for organic liquids37): |  | (21) |
where νm represents the molecular volume (in nm3).
6.3 Standard enthalpy 
The standard enthalpy of formation (
) is the most important thermodynamic quantity for pure ionic liquids. As an example, let us consider solid 1-ethyl-pyridinium chloride (referred to as [C2Py]Cl). One can derive the following equation from the thermodynamic cycle shown in Fig. 13: |  | (22) |
where ΔHL is the lattice enthalpy. The values of
and
can be assessed from ab initio calculations. The lattice enthalpy is given by the following expression:40 |  | (23) |
where UPOT represents the lattice potential energy, n is the number of ion types in the formula unit, ni is the number of ions of type i, and ci depends on the type of ion (3 for a monoatomic ion i, 5 for a linear polyatomic ion i, 6 for a nonlinear polyatomic ion i).
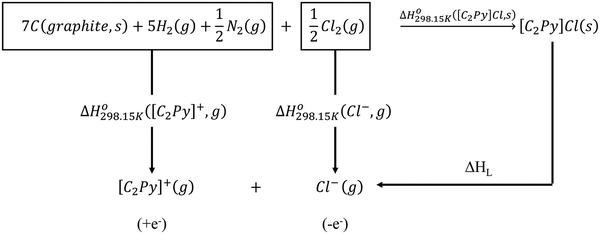 |
| Fig. 13 Thermodynamic cycle to calculate the standard enthalpy of solid [C2Py]Cl. | |
The lattice potential energy is calculated as follows:40
| 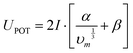 | (24) |
where
α and
β are fitted constants obtained by Gutowski
et al.68 for a series of salt compounds containing the organic cations NH
4+, N
2H
5+, CH
3NH
3+, (CH
3)
2NH
2+, (CH
3)
3NH
+, (C
2H
5)
2NH
2+ and (C
2H
5)
3NH
+:
| α = 83 262 J mol−1 nm | (25) |
| β = 157 318 J mol−1 | (26) |
and
|  | (27) |
In
eqn (27),
I is the ionic strength factor,
n is again the number of ion types in the formula unit,
ni is again the number of ions of type
i, and
zi is the valence of ion
i.
Eqn (24) is only valid for lattice potential energy values lower than 5000 kJ mol
−1.
40
The VBT is applied to the solid and liquid phases of [C2Py]Cl, [C2Py]Br, [C4Py]Cl and [C4Py]Br in the Appendix. In particular, all details are given for [C2Py]Cl and [C2Py]Br.
7. The [C2Py], [C4Py]‖Cl, Br system
This section describes a thermodynamic model for the ternary reciprocal system [C2Py]Cl–[C2Py]Br–[C4Py]Cl–[C4Py]Br. As mentioned previously, the thermodynamic properties of all four pure compounds must be known or estimated, so that the Gibbs free energy change for the exchange reaction [C2Py]Br (liquid) + [C4Py]Cl (liquid) = [C2Py]Cl (liquid) + [C4Py]Br (liquid) can be estimated. First of all, the thermodynamic properties (
,
and Cp(T)) of all four pure ionic liquids are assessed by using all available data from the literature and by applying the VBT whenever relevant. Then, the phase diagrams of the four common-ion binary subsystems are modeled. Finally, the liquidus projection of the ternary reciprocal system and the two diagonal sections ([C4Py]Br–[C2Py]Cl and [C4Py]Cl–[C2Py]Br) are calculated solely from the optimized model parameters for the four common-ion binary subsystems.
7.1 Pure ionic liquids
Fig. 14 presents the chemical structures of the four pure compounds investigated. Table 6 gathers all thermodynamic data collected from the literature for the four pyridinium-based pure ionic liquids. All phase diagram measurements in the [C2Py], [C4Py]‖Cl, Br ternary reciprocal system were conducted recently by Stolarska et al. (unpublished results). For the sake of consistency, the temperatures of fusion of the four pure compounds measured by these authors were selected in the present study. The selection of the enthalpies of fusion is described at the beginning of the Appendix. The thermodynamic properties (
,
and Cp(T)) of the four pure compounds were then assessed using the VBT, and are displayed in Table 7. These various values are discussed in detail in the Appendix. In particular, owing to the lack of relevant density measurements, the densities at 298.15 K of each pure solid and liquid were assessed using the method proposed by Krossing et al.87 and the group contribution method of Paduszynski and Domanska,88 respectively. As explained in the Appendix, Verevkin et al.89 measured by DSC
for the liquid phases of [C2Py]Cl, [C2Py]Br, [C4Py]Cl and [C4Py]Br. These experimental values were favoured in the present work. The thermodynamic properties finally selected for the four pyridinium-based compounds are displayed in Table 8. Note that Tables 7 and 8 only differ by the
values for the solid and liquid phases. In Table 7,
was estimated by the VBT and
was then derived from it using other thermodynamic properties (Cp and properties of fusion). In Table 8,
was taken directly from Verevkin et al.89 and
was then derived from it using other thermodynamic properties (Cp and properties of fusion). The shifts between the two values of
are about −27.4 kJ mol−1 for [C2Py]Cl, −43.8 kJ mol−1 for [C2Py]Br, 1.7 kJ mol−1 for [C4Py]Cl, and −16.0 kJ mol−1 for [C4Py]Br.
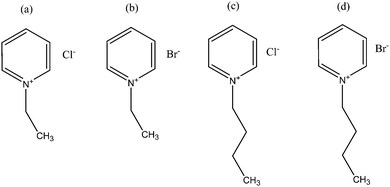 |
| Fig. 14 Chemical structure of the pyridinium-based ionic liquids: (a) [C2Py]Cl; (b) [C2Py]Br; (c) [C4Py]Cl; (d) [C4Py]Br. | |
Table 6 Thermodynamic data collected from the literature for the pure compounds [C2Py]Cl, [C2Py]Br, [C4Py]Cl and [C4Py]Br
Cation |
Anion |
Water content [ppm] |
Purity [%] |
Experimental technique |
 [kJ mol−1] |
C
p [J mol−1 K−1] |
Temperature of fusion (K) |
Enthalpy of fusion (kJ mol−1) |
Ref. |
s and l refer to the solid and liquid phases, respectively. AC: adiabatic calorimetry. |
[C2Py]+ |
Cl− |
<100 |
99 |
DSC |
s: −125.1
l: −113.3
|
— |
435 |
23.5 |
89
|
— |
— |
DSC |
— |
— |
390 |
— |
Stolarska et al. (unpublished results) |
Br− |
<100 |
99 |
DSC |
s: −82.0
l: −77.1
|
— |
391.3 |
12.8 |
89
|
— |
— |
DSC |
— |
— |
395 |
— |
Stolarska et al. (unpublished results) |
— |
>99.5 |
AC |
— |
76.4–300.3 J mol−1 K−1 between 77.7 and 409.7 K |
391.31 |
12.77 |
90
|
|
[C4Py]+ |
Cl− |
<100 |
99 |
DSC |
s: −177.7
l: −164.7
|
— |
393.3 |
20.7 |
91
|
— |
— |
DSC |
— |
— |
408 |
— |
Stolarska et al. (unpublished results) |
Br− |
<100 |
99 |
DSC |
s: −144.1
l: −130.5
|
— |
378 |
20.4 |
89
|
— |
— |
DSC |
— |
— |
380 |
— |
Stolarska et al. (unpublished results) |
Table 7 Standard thermodynamic properties of the pyridinium-based pure compounds estimated by the VBT
Table 8 Selected thermodynamic properties for the pyridinium-based pure compounds
Discussion: using the selected thermodynamic data in Table 8, the thermodynamic stability of the [C2Py]Cl, [C2Py]Br, [C4Py]Cl and [C4Py]Br pure compounds was examined both in the solid (at 25 °C) and liquid (slightly above the melting temperature) states. Each compound is calculated to decompose into C(graphite, s) and gaseous products. These reactions of decomposition are highly thermodynamically favoured since they have a very negative Gibbs free energy change. However, they are kinetically hindered owing to the necessity to break several bonds. A similar situation occurs for some common organic compounds such as benzene and acetone (both liquid at 25 °C) as well as naphthalene and phenol (both solid at 25 °C). Although these various compounds are stable at room temperature, they are all calculated to decompose thermodynamically using the FactSage databases.92
The thermodynamic properties of pure ionic liquids are useful to assess their relative stability. More importantly, they are required to estimate the exchange Gibbs free energy (eqn (4)), which is an important parameter to model the phase diagrams of ternary reciprocal systems such as [C2Py], [C4Py]‖Cl, Br. As mentioned previously, using the selected thermodynamic data in Table 8,
for the exchange reaction [C2Py]Br (liquid) + [C4Py]Cl (liquid) = [C2Py]Cl (liquid) + [C4Py]Br (liquid) is estimated as −1.6 kJ mol−1 at 25 °C, −1.9 kJ mol−1 at 100 °C, and −2.4 kJ mol−1 at 150 °C. Using the thermodynamic data in Table 7 (mainly derived from the VBT),
is calculated as −2.9 kJ mol−1 at 25 °C, −3.3 kJ mol−1 at 100 °C, and −3.7 kJ mol−1 at 150 °C. Although these two series of values are very close to each other, this is fortuitous since the shift between the two values of
in Tables 7 and 8 is as high as −43.8 kJ mol−1 for [C2Py]Br. As reported by Robelin,15 the
value estimated from the VBT for solid [C2MIm]NO3 was about 46 kJ mol−1 lower than the experimental value of Emel’yanenko et al.93 obtained by combustion calorimetry. These shifts are at least partly due to the uncertainty on the value of the standard enthalpy of formation at 298.15 K of the organic cation in the gas state, derived from ab initio calculations. It can thus be concluded that, in the general case, the
values assessed from the VBT are not accurate enough to model the phase diagram of reciprocal systems; experimental values of
(such as the data of Verevkin et al.89 obtained by DSC for the [C2Py]Cl, [C2Py]Br, [C4Py]Cl and [C4Py]Br pure liquids) are required.
7.2 Common-ion binary subsystems
7.2.1 The [C2Py]Br–[C4Py]Br binary system.
The phase diagram has been measured by DSC (unpublished results). No solid solubility was reported, and the measured limiting slopes of the [C2Py]Br and [C4Py]Br liquidus curves agree with eqn (13). No intermediate compound has been observed. The optimized Gibbs free energy of reaction (1) is: | Δg[C2Py][C4Py]/Br2/(J mol−1) = 159.0 − 29.3x[C4Py][C4Py] | (28) |
where x[C4Py][C4Py] is the mole fraction of second-nearest-neighbor ([C4Py]–Br–[C4Py]) pairs. The calculated phase diagram is shown along with the measurements in Fig. 15. This is a simple eutectic system. The reported characteristics of the experimental eutectic are {T = 342 K (i.e. 68.85 °C), 48.0 mol% [C4Py]Br} (unpublished results). The characteristics of the calculated eutectic are {69 °C, 48.0 mol% [C4Py]Br}.
 |
| Fig. 15 Calculated [C2Py]Br–[C4Py]Br phase diagram, temperature versus mole fraction of [C4Py]Br. Experimental data are from Stolarska et al. (unpublished results) (●). | |
7.2.2 The [C2Py]Cl–[C4Py]Cl binary system.
The phase diagram has been measured by DSC (unpublished results). No solid solubility was reported, and the measured limiting slopes of the [C2Py]Cl and [C4Py]Cl liquidus curves agree with eqn (13). No intermediate compound has been observed. The optimized Gibbs free energy of reaction (1) is: | Δg[C2py][C4Py]/Cl2/(J mol−1) = 627.6 − 1464.4x[C2py][C2py]+ 251.0x[C4Py][C4Py] | (29) |
where xii is the mole fraction of second-nearest-neighbor (i–Cl–i) pairs. The calculated phase diagram is compared to the measurements in Fig. 16. Again, this is a simple eutectic system. It was not possible to reproduce the experimental liquidus temperature of 76.1 °C at 20.0 mol% [C4Py]Cl. If this data point had been favoured, then the calculated eutectic temperature would be significantly lower than the experimental one. The reported characteristics of the experimental eutectic are {T = 349 K (i.e. 75.85 °C), 20.5 mol% [C4Py]Cl} (unpublished results). As seen in Fig. 16, the eutectic temperatures measured for the [C2Py]Cl–[C4Py]Cl binary system are relatively scattered: 75 ± 8 °C. The characteristics of the calculated eutectic are {76 °C, 27.3 mol% [C4Py]Cl}.
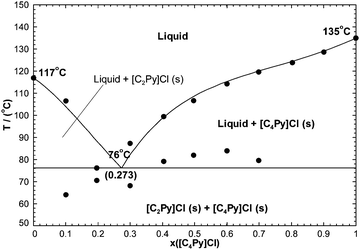 |
| Fig. 16 Calculated [C2Py]Cl–[C4Py]Cl phase diagram, temperature versus mole fraction of [C4Py]Cl. Experimental data are from Stolarska et al. (unpublished results) (●). | |
7.2.3 The [C2Py]Cl–[C2Py]Br binary system.
The phase diagram has been measured by DSC (unpublished results). A full solid solution was reported, and no intermediate compound has been observed. As explained previously, the Cl− and Br− anions have similar ionic radii and therefore the binary liquid was assumed to be ideal. That is:The [C2Py]Cl–[C2Py]Br extensive solid solution was modeled with the Compound Energy Formalism (CEF). Its optimized Gibbs free energy is given by eqn (9), and the corresponding excess Gibbs free energy is: |  | (31) |
The calculated phase diagram is shown along with the measurements in Fig. 17. Agreement is excellent.
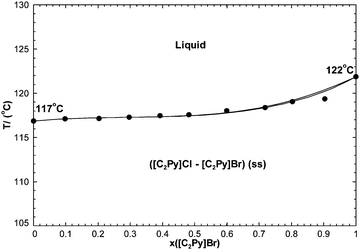 |
| Fig. 17 Calculated [C2Py]Cl–[C2Py]Br phase diagram, temperature versus mole fraction of [C2Py]Br. Experimental data are from Stolarska et al. (unpublished results) (●). | |
7.2.4 The [C4Py]Cl–[C4Py]Br binary system.
The phase diagram has been measured by DSC (unpublished results). Again, a full solid solution was reported, and no intermediate compound has been observed. By analogy with the [C2Py]Cl–[C2Py]Br system, the binary liquid was assumed to be ideal. That is:The [C4Py]Cl–[C4Py]Br extensive solid solution was modeled with the CEF. Its optimized Gibbs free energy is given by eqn (9), with: |  | (33) |
The calculated phase diagram is compared to the measurements in Fig. 18. Agreement is excellent.
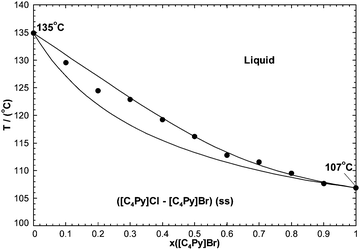 |
| Fig. 18 Calculated [C4Py]Cl–[C4Py]Br phase diagram, temperature versus mole fraction of [C4Py]Br. Experimental data are from Stolarska et al. (unpublished results) (●). | |
7.3 The [C2Py]Cl–[C2Py]Br–[C4Py]Cl–[C4Py]Br ternary reciprocal system
The [C2Py]Cl–[C2Py]Br–[C4Py]Cl–[C4Py]Br ternary reciprocal system has been studied by DSC (unpublished results). Stolarska et al. measured the [C4Py]Br–[C2Py]Cl and [C4Py]Cl–[C2Py]Br sections. The corresponding calculated sections are shown along with the measurements in Fig. 19 and 20, respectively. (Glass transitions were measured below 250 K (i.e. −23 °C) in both sections and are not shown in the figures.) No additional parameters were introduced for the liquid phase. The thermodynamic properties of the latter are calculated solely from the optimized parameters for the four common-ion binary subsystems (eqn (28)–(30) and (32)). As seen in Fig. 19 and 20, the measurements are somewhat scattered but agree very satisfactorily with the calculations. The calculated liquidus projection of the [C2Py]Cl–[C2Py]Br–[C4Py]Cl–[C4Py]Br system is shown in Fig. 21. As mentioned previously, the ternary reciprocal liquid exhibits small deviations from ideality, owing to the fact that the two cations only differ by the length of their alkyl chains and that the two anions are halides with similar ionic radii. In the reciprocal square in Fig. 21, the four apices correspond to the pure salts: [C2Py]Cl, [C4Py]Cl, [C2Py]Br and [C4Py]Br. Note that there is a degree of freedom in expressing the compositions in such a system. For example, a solution of one mole of [C2Py]Cl and one mole of [C4Py]Br could equally well be described as a mixture of one mole of [C4Py]Cl and one mole of [C2Py]Br. Therefore, the compositions are expressed in terms of cationic and anionic equivalent fractions. The X-axis corresponds to the cationic molar ratio
, and the Y-axis corresponds to the anionic molar ratio
(where ni is the number of moles of ion i).
 |
| Fig. 19 Calculated (predicted) [C4Py]Br–[C2Py]Cl section of the [C2Py]Cl–[C2Py]Br–[C4Py]Cl–[C4Py]Br phase diagram along with measurements from Stolarska et al. (unpublished results) (●). Notations: A: ([C2Py]Cl–[C2Py]Br)(ss), B: ([C4Py]Cl–[C4Py]Br)(ss). | |
 |
| Fig. 20 Calculated (predicted) [C4Py]Cl–[C2Py]Br section of the [C2Py]Cl–[C2Py]Br–[C4Py]Cl–[C4Py]Br phase diagram along with measurements from Stolarska et al. (unpublished results) (●). Notations: A: ([C2Py]Cl–[C2Py]Br)(ss), B: ([C4Py]Cl–[C4Py]Br)(ss). | |
 |
| Fig. 21 Calculated (predicted) liquidus projection of the [C2Py]Cl–[C2Py]Br–[C4Py]Cl–[C4Py]Br system. | |
The [C2Py]Cl–[C2Py]Br–[C4Py]Cl–[C4Py]Br system is indeed a “ternary” system since it consists of four ionic species and the following condition of electroneutrality is respected at any composition inside the reciprocal square:
| n[C2Py]+ + n[C4Py]+ = nCl− + nBr− | (34) |
In
Fig. 21, the univariant line (along which the liquid coexists with the [C
2Py]Cl–[C
2Py]Br and [C
4Py]Cl–[C
4Py]Br extensive solid solutions) is shown as a bold line. By convention, the arrow indicates the direction of decreasing temperature along this line. The liquidus isotherms are displayed as thin lines. There are no calculated ternary invariant reactions, and the minimum liquidus temperature in the ternary reciprocal system corresponds to the binary eutectic reaction liquid = [C
2Py]Br + [C
4Py]Br at 69 °C.
8. Conclusions
For the first time, the phase diagrams of a common-cation ternary system ([C4MPyrr]Cl–[C4MPyrr]Br–[C4MPyrr]BF4) and of a ternary reciprocal system (i.e. a system with two cations and two anions: [C2Py], [C4Py]‖Cl, Br), both consisting only of pyrrolidinium-based and pyridinium-based ionic liquids, respectively, were modeled successfully. The Modified Quasichemical Model20–22 was used for the liquid phase, whereas the Compound Energy Formalism (CEF)61,62 was used for the relevant solid solutions. The two multicomponent liquids investigated display relatively small deviations from ideality. The Volume-based Thermodynamics (VBT) from Glasser and Jenkins35–41 was used in conjunction with the available data from the literature to assess the thermodynamic properties of the [C2Py]Cl, [C2Py]Br, [C4Py]Cl and [C4Py]Br pure compounds, thus making it possible to estimate the Gibbs free energy change for the exchange reaction [C2Py]Br (liquid) + [C4Py]Cl (liquid) = [C2Py]Cl (liquid) + [C4Py]Br (liquid). The optimized parameters form a database for use with the FactSage thermochemical software92 and may be used, along with the Gibbs free energy minimization software, to calculate phase equilibria in the [C4MPyrr]{Cl,Br,BF4} and [C2Py], [C4Py]‖Cl, Br systems.
The phase diagrams of the three binary subsystems of the [C4MPyrr]{Cl,Br,BF4} common-cation system were modeled based on the available DSC measurements.50 Whereas [C4MPyrr]{Cl,BF4} and [C4MPyrr]{Br,BF4} are simple eutectic systems, [C4MPyrr]{Cl,Br} exhibits a eutectic behavior with two terminal solid solutions. The thermodynamic properties of the ternary liquid were calculated from the optimized model parameters for the three binary subsystems using a standard asymmetric interpolation method with [C4MPyrr]BF4 as the asymmetric component. A small ternary excess parameter was included for the liquid phase in order to best reproduce the two isoplethal sections at constant 50 mol% [C4MPyrr]BF4 and at a constant molar ratio [C4MPyrr]Cl/([C4MPyrr]Cl + [C4MPyrr]Br) of 0.85, which were measured by DSC in the present work. The calculated minimum liquidus temperature in the ternary system corresponds to a ternary eutectic at 91 °C, very close to the binary eutectic in the [C4MPyrr]{Cl,BF4} system.
The phase diagrams of the four common-ion binary subsystems of the [C2Py], [C4Py]‖Cl, Br system were modeled based on the available DSC measurements (unpublished results). Whereas {[C2Py], [C4Py]}Cl and {[C2Py], [C4Py]}Br are simple eutectic systems, [C2Py]{Cl,Br} and [C4Py]{Cl,Br} both display an extensive solid solution over the entire composition range. The thermodynamic properties of the ternary reciprocal liquid were calculated solely from the optimized parameters for the four common-ion binary subsystems. The experimental diagonal sections [C4Py]Br–[C2Py]Cl and [C4Py]Cl–[C2Py]Br are very satisfactorily reproduced by the model. The calculated minimum liquidus temperature in the [C2Py], [C4Py]‖Cl, Br system corresponds to the binary eutectic at 69 °C in the {[C2Py], [C4Py]}Br system.
Conflicts of interest
There are no conflicts of interest to declare.
Appendix: pure ionic liquids [C2Py]Cl, [C2Py]Br, [C4Py]Cl and [C4Py]Br
The properties of fusion proposed by Verevkin et al.89 for [C2Py]Cl were discarded since these are estimated values. The temperature of fusion was assessed from general trends of ionic liquids as 435 K, which is 45 K higher than the experimental value of Stolarska et al. (unpublished results). The enthalpy of fusion was estimated as 23
500 J mol−1 using the modified Walden's rule.89 The properties of fusion of [C2Py]Br and [C4Py]Br were measured by Verevkin et al.,89 and the ones of [C4Py]Cl were measured by Verevkin et al.91 The corresponding values of the entropy of fusion are 32.4, 54.0 and 52.6 J mol−1 K−1, respectively. The entropies of fusion of [C4Py]Br and [C4Py]Cl are very close to each other. Similarly, the entropy of fusion of [C2Py]Cl was assumed to be identical to that of [C2Py]Br, and the enthalpy of fusion of [C2Py]Cl was then estimated as 12
638 J mol−1. The thermodynamic properties (
,
and Cp(T)) of the four pure compounds were assessed using the VBT. The obtained values are discussed in detail below.
As mentioned previously, the VBT requires a knowledge of the molecular volume νm (expressed in nm3). Verevkin et al.91 measured the density of pure liquid [C4Py]Cl as 1.010 g cm−3 at 418.2 K using a pycnometer. To our knowledge, no other experimental density values are available for the solid or liquid phases of [C2Py]Cl, [C4Py]Cl, [C2Py]Br and [C4Py]Br. The molecular volume of each pure solid at 298.15 K was finally assessed using the method proposed by Krossing et al.87 Based on the cell parameters derived from X-ray diffraction data, these authors determined the molecular volume νm of various ionic liquids in the solid state. Assuming the additivity of the ion volumes (i.e. νm = νcation + νanion), Krossing et al. reported volume values for various ions, which are supposed to be independent of the crystallographic structure. The following values were proposed:87 0.047 nm3 for Cl−, 0.056 nm3 for Br−, and 0.198 nm3 for [C4Py]+. Although no volume value was reported for [C2Py]+, an estimation can be made from the volume value reported for [C4Py]+. Krossing et al.87 proposed the following ion volume values for various methyl-imidazolium-based cations differing from each other by one –CH2– group: 0.156 nm3 for [C2MIm]+, 0.178 nm3 for [C3MIm]+, 0.196 nm3 for [C4MIm]+ and 0.219 nm3 for [C5MIm]+. Krossing et al. concluded that the addition of one –CH2– group in the alkyl chain of these various ions corresponds to an average volume increase of 0.021 nm3. The volume of [C2Py]+ may thus be estimated as 0.156 nm3 (i.e. 0.198 − 2 × 0.021). The derived molar volumes and densities of the four pyridinium-based ionic liquids in the solid state are presented in Table 9.
Table 9 Molar volume (Vm) and density (ρ) of pyridinium-based ionic liquids in the solid state estimated using the method proposed by Krossing et al.87
Compound |
V
m (cm3 mol−1) |
ρ (g cm−3) |
[C2Py]Cl |
122.3 |
1.174 |
[C2Py]Br |
127.7 |
1.473 |
[C4Py]Cl |
147.9 |
1.161 |
[C4Py]Br |
153.0 |
1.412 |
In order to assess the density of ionic liquids in the liquid state, Paduszynski and Domanska88 developed a new method based on generalized empirical correlations and group contributions obtained from a comprehensive database of experimental data for a variety of ionic liquids. These authors considered the organic cations to consist of a core along with alkyl chains or functional groups attached to it, whereas the anions were treated as individual groups. The molar volume of a given ionic liquid in the liquid state is then estimated by adding the molar volumes of its constitutive groups. The molar volumes of the constitutive groups of the four pyridinium-based ionic liquids in the liquid state and the derived densities of the latter are given in Table 10. The density of pure liquid [C4Py]Cl was calculated to be 1.107 g cm−3 at 298.15 K using the group contribution method developed by Paduszynski and Domanska, and then 1.028 g cm−3 at 418.2 K using eqn (3) from ref. 88. The latter compares reasonably well with the experimental value of 1.010 g cm−3 obtained by Verevkin et al.91 (relative shift of 1.74%). The same relative shift of 1.74% was finally applied to the original density values estimated at 298.15 K (and displayed in Table 10) for the [C2Py]Cl, [C2Py]Br, [C4Py]Cl and [C4Py]Br pure liquids.
Table 10 Molar volume (Vm) of constitutive groups and density (ρ) of pyridinium-based ionic liquids in the liquid state estimated using the method proposed by Paduszynski and Domanska88
Compound |
Constitutive groups |
Number |
V
m (cm3 mol−1) |
ρ (g cm−3) |
[C2Py]Cl |
Pyridinium |
1 |
65.95 |
1.181 |
N–CH2 |
1 |
2.88 |
CH3 |
1 |
26.16 |
Cl |
1 |
26.66 |
|
[C2Py]Br |
Pyridinium |
1 |
65.95 |
1.419 |
N–CH2 |
1 |
2.88 |
CH3 |
1 |
26.16 |
Br |
1 |
37.54 |
|
[C4Py]Cl |
Pyridinium |
1 |
65.95 |
1.107 |
N–CH2 |
1 |
2.88 |
CH2 |
2 |
16.73 |
CH3 |
1 |
26.16 |
Cl |
1 |
26.66 |
|
[C4Py]Br |
Pyridinium |
1 |
65.95 |
1.302 |
N–CH2 |
1 |
2.88 |
CH2 |
2 |
16.73 |
CH3 |
1 |
26.16 |
Br |
1 |
37.54 |
To our knowledge, no heat capacity measurements are available for the [C2Py]Cl, [C4Py]Cl and [C4Py]Br compounds (solid and liquid). The Cp values at 298.15 K were assessed using eqn (20) (valid for 1-alkyl-pyridinium-based compounds) along with our estimated molecular volumes of the solid and liquid phases. Then, an identical procedure (described below) was used to assess the standard absolute entropies
of the solid and liquid phases. As an example, let us consider the [C2Py]Cl compound. As a starting point, using eqn (21) the
values of the solid and liquid phases were estimated as 282.5 and 285.6 J mol−1 K−1, respectively. As explained previously, the entropy of fusion of [C2Py]Cl is about 32.4 J mol−1 K−1. Assuming constant Cp values, one can derive the following expression:
|  | (35) |
It follows that

. Since this term was initially only about 3.1 J mol
−1 K
−1, the shift of (31.6–3.1) = 28.5 = 2 × 14.2 was equally distributed on the initial values of

for the solid and liquid phases. That is, the

values finally selected for the solid and liquid phases of [C
2Py]Cl are about 268.3 and 299.9 J mol
−1 K
−1, respectively.
Tong et al.90 measured the heat capacity of [C2Py]Br by adiabatic calorimetry over the temperature range 77–410 K. These Cp data were fitted with the following equations:
| Cp(T)/(J mol−1 K−1) = A + BT + CT2 = 43.0120 + 0.3308T + 0.0008T2 (T in K) for the solid phase | (36) |
| Cp(T)/(J mol−1 K−1) = a + bT = −23.4290 + 0.7903T (T in K) for the liquid phase | (37) |
The calculated
Cp values of [C
2Py]Br are compared to the measurements of Tong
et al.90 in
Fig. 22. One can derive the following expression for [C
2Py]Br:
| 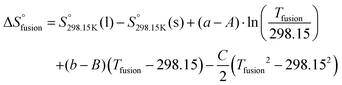 | (38) |
As a starting point, using
eqn (21) the

values of the solid and liquid phases of [C
2Py]Br were estimated as 293.8 and 308.5 J mol
−1 K
−1, respectively. Using a procedure similar to that for [C
2Py]Cl, the

values finally selected for the solid and liquid phases of [C
2Py]Br are about 284.4 and 317.9 J mol
−1 K
−1, respectively.
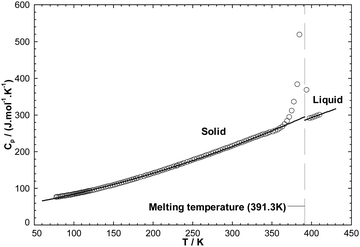 |
| Fig. 22 Calculated heat capacity of [C2Py]Br versus temperature. Experimental data are from Tong et al.90 (○). Cp = 43.012 + 0.3308T + 0.0008T2 (solid), Cp = −23.429 + 0.7903T (liquid). | |
Using an equation similar to eqn (22) along with eqn (23)–(27), values of
for the solid phases of [C2Py]Cl, [C2Py]Br, [C4Py]Cl and [C4Py]Br were estimated, based on the values
and
reported by Verevkin et al.89 and the values
and
taken from the FactSage thermodynamic databases.92 The standard thermodynamic properties of the four pyridinium-based compounds estimated by the VBT are gathered in Table 7.
Verevkin et al.89 measured by DSC
for the liquid phases of [C2Py]Cl, [C2Py]Br, [C4Py]Cl and [C4Py]Br. For each of these four pure liquids, these authors derived
from the experimental reaction enthalpy ΔrH° related to the formation of the pyridinium-based ionic liquid from precursors (liquid pyridine and liquid C4H9Cl in the case of liquid [C4Py]Cl). A layer of ionic liquid solvent ([C4MIm][NTf2]) was used to separate the precursors and to avoid beginning of the chemical reaction outside the DSC apparatus. The reaction enthalpy ΔrH°, derived by integration of the DSC peak, corresponds to the temperature of the peak maximum (about 503 K for [C4Py]Cl). Verevkin et al.89 showed that the correction (ΔCp) required to adjust the reaction enthalpy to the reference temperature 298.15 K is within the DSC experimental uncertainties of about 1–3 kJ mol−1. Therefore, the experimental value of ΔrH° was considered to be valid at 298.15 K and, using experimental values from the literature for
of the precursors, Verevkin et al.89 estimated
for the liquid pyridinium-based compound. These experimental values of
from Verevkin et al.89 were finally favoured in the present work. The thermodynamic properties finally selected for the four pyridinium-based compounds are displayed in Table 8.
Kabo et al.94 proposed a group contribution method for the assessment of
of various liquid 1-alkyl-3-methylimidazolium nitrates [CnMIm]NO3 (with n = 1, 2, 4). The substitution procedure was described by Emel’yanenko et al.,95 and the group contribution values were determined by Domalski and Hearing.96 Kabo et al.94 selected liquid 3-methylimidazolium nitrate [HMIm]NO3 as the starting compound with a known (experimental)
value. [C1MIm]NO3 is obtained from [HMIm]NO3 by substituting the H atom connected to a N atom for a –CH3 group, which corresponds to an enthalpy increment
.96 Then, [CnMIm]NO3 (with n = 2, 4) is obtained from [C1MIm]NO3 by substituting one time or three times a H atom connected to a C atom for a –CH3 group, which corresponds to an enthalpy increment
.96 In the present work, a similar approach was used for the [C2Py]Cl, [C2Py]Br, [C4Py]Cl and [C4Py]Br pure liquids. The liquids [C1Py]Cl and [C1Py]Br were selected as the starting compounds, since their
values were measured by Verevkin et al.89 using DSC.
In Table 11, for each of the four pyridinium-based compounds, the
value derived using the group contribution method from Kabo et al.94 (series 3) is given along with the experimental value of Verevkin et al.89 (series 1) displayed in Table 8, and the value derived from the VBT value of
(series 2) displayed in Table 7. As mentioned previously, the shifts between series 1 and 2 lie between −43.8 and +1.7 kJ mol−1, whereas the shifts between series 1 and 3 lie between 5.0 and 8.7 kJ mol−1. The calculated shifts for series 3 are much smaller than those for series 2. This is not surprising since series 3 was obtained with the group contribution method from Kabo et al.,94 which requires the knowledge of
for a starting compound similar to the targeted compound. On the other hand, series 2 only required a knowledge of the molecular volumes (for application of the VBT) along with the properties of fusion.
Table 11 Comparison between experimental and estimated values of
for the pyridinium-based pure compounds
Compound |
From Verevkin et al. (series 1) |
From VBT (series 2) |
From group contribution method of Kabo et al.94 (series 3) |
[C2Py]Cl |
−113 30089 |
−140 704 |
−104 630 |
[C2Py]Br |
−77 10089 |
−120 880 |
−72 130 |
[C4Py]Cl |
−164 70091 |
−162 987 |
−156 090 |
[C4Py]Br |
−130 50089 |
−146 505 |
−123 590 |
Kabo et al.94 also proposed two linear regression equations to assess
for the solid and liquid phases of various 1-butyl-3-methyl-imidazolium compounds with different anionic groups ([C4MIm]X) as a function of the known value of
for the corresponding potassium salt KX, based on the available data for X = Br, I, and NO3. In the present work, a similar approach was used for the liquid phase of 1-butylpyridinium compounds ([C4Py]X), using the experimental
values available for X = Cl, Br and BF4 (see Table 12).
Table 12 Experimental values of
from the literature used to derive eqn (39)
Anion X |
 [kJ mol−1] |
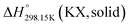 [kJ mol−1] |
Cl− |
−164.791 |
−436.733 |
Br− |
−130.589 |
−393.833 |
BF4− |
−1356.397 |
−1887.033 |
The following linear regression equation was obtained:
|  | (39) |
where X is an anion. The standard error of a prediction is ±0.73 kJ mol
−1.
Eqn (39) can be used to estimate
for a given 1-butylpyridinium compound from the known value of
for the corresponding potassium salt. Owing to the lack of experimental data, no linear regression equation was derived for 1-ethylpyridinium compounds ([C2Py]X).
Acknowledgements
The modeling part of this project was supported by the Natural Sciences and Engineering Research Council of Canada (Discovery Grant RGPIN 435893-2013). The new DSC measurements in the ternary system [C4MPyrr]{Cl,Br,BF4} were supported by the National Science Centre (Poland), project SONATA (No. 2011/03/D/ST5/06200). Constructive discussions with Prof. Nick Virgilio were much appreciated.
References
- H. Olivier, J. Mol. Catal. A: Chem., 1999, 146, 285–289 CrossRef CAS
.
-
P. Wasserscheid and T. Welton, Ionic liquids in synthesis, John Wiley & Sons, 2008 Search PubMed
.
- J. J. Raj, C. D. Wilfred, S. N. Shah, M. Pranesh, M. A. Mutalib and K. C. Lethesh, J. Mol. Liq., 2017, 225, 281–289 CrossRef CAS
.
-
M. Koel, Ionic liquids in chemical analysis, CRC Press, 2008 Search PubMed
.
- J. F. Brennecke and E. J. Maginn, AIChE J., 2001, 47, 2384–2389 CrossRef CAS
.
-
C. Hardacre and V. Parvulescu, Catalysis in Ionic Liquids: From Catalyst Synthesis to Application, Royal Society of Chemistry, 2014 Search PubMed
.
-
H. Ohno, Electrochemical aspects of ionic liquids, John Wiley & Sons, 2011 Search PubMed
.
-
Z. Fang, Production of biofuels and chemicals with ionic liquids, Diss. Tohoku University, Japan, 2014 Search PubMed
.
-
A. Stark and K. R. Seddon, Kirk-Othmer encyclopedia of chemical technology, 2007 Search PubMed
.
- H. Niedermeyer, J. P. Hallett, I. J. Villar-Garcia, P. A. Hunt and T. Welton, Chem. Soc. Rev., 2012, 41, 7780–7802 RSC
.
- N. V. Plechkova and K. R. Seddon, Chem. Soc. Rev., 2008, 37, 123–150 RSC
.
- M. Kick, P. Keil and A. König, Fluid Phase Equilib., 2013, 338, 172–178 CrossRef CAS
.
- G. J. Maximo, R. J. Santos, P. Brandao, J. M. Esperança, M. C. Costa, A. J. Meirelles, M. G. Freire and J. A. Coutinho, Cryst. Growth Des., 2014, 14, 4270–4277 CAS
.
- A. R. R. Teles, H. Correia, G. J. Maximo, L. P. Rebelo, M. G. Freire, A. B. Pereiro and J. A. Coutinho, Phys. Chem. Chem. Phys., 2016, 18, 25741–25750 RSC
.
- C. Robelin, Fluid Phase Equilib., 2016, 409, 482–494 CrossRef CAS
.
- A. A. Fannin Jr, D. A. Floreani, L. A. King, J. S. Landers, B. J. Piersma, D. J. Stech, R. L. Vaughn, J. S. Wilkes and J. L. Williams, J. Phys. Chem., 1984, 88, 2614–2621 CrossRef
.
- K. Kubota, T. Nohira, G. Takuya and R. Hagiwara, ECS Trans., 2009, 16, 91–98 CAS
.
- K. Kubota, T. Nohira and R. Hagiwara, Electrochim. Acta, 2012, 66, 320–324 CrossRef CAS
.
- G. K. Gbassi and C. Robelin, Fluid Phase Equilib., 2015, 406, 134–141 CrossRef CAS
.
- A. Pelton, S. Degterov, G. Eriksson, C. Robelin and Y. Dessureault, Metall. Mater. Trans. B, 2000, 31, 651–659 CrossRef
.
- A. D. Pelton and P. Chartrand, Metall. Mater. Trans. A, 2001, 32, 1355–1360 CrossRef
.
- A. D. Pelton, P. Chartrand and G. Eriksson, Metall. Mater. Trans. A, 2001, 32, 1409–1416 CrossRef
.
-
P. Chartrand and A. D. Pelton, Light Metals, Warrendale, Proceedings, 2002, 245–252.
- P. Chartrand, F. Gemme and C. Robelin, Procedia Eng., 2014, 83, 250–258 CrossRef CAS
.
- C. Robelin, P. Chartrand and A. D. Pelton, J. Chem. Thermodyn., 2015, 83, 12–26 CrossRef CAS
.
- K. Fumino, T. Peppel, M. Geppert-Rybczyńska, D. H. Zaitsau, J. K. Lehmann, S. P. Verevkin, M. Köckerling and R. Ludwig, Phys. Chem. Chem. Phys., 2011, 13, 14064–14075 RSC
.
- K. Ala'a, A. M. Greenway, P. B. Hitchcock, T. J. Mohammed, K. R. Seddon and J. A. Zora, J. Chem. Soc., Chem. Commun., 1986, 1753–1754 Search PubMed
.
- K. Dong, S. Zhang, D. Wang and X. Yao, J. Phys. Chem. A, 2006, 110, 9775–9782 CrossRef CAS PubMed
.
- P. A. Hunt, C. R. Ashworth and R. P. Matthews, Chem. Soc. Rev., 2015, 44, 1257–1288 RSC
.
- A. Elaiwi, P. B. Hitchcock, K. R. Seddon, N. Srinivasan, Y.-M. Tan, T. Welton and J. A. Zora, J. Chem. Soc., Dalton Trans., 1995, 3467–3472 RSC
.
- W. M. Reichert, J. D. Holbrey, R. P. Swatloski, K. E. Gutowski, A. E. Visser, M. Nieuwenhuyzen, K. R. Seddon and R. D. Rogers, Cryst. Growth Des., 2007, 7, 1106–1114 CAS
.
- K. Dong, S. Zhang and J. Wang, Chem. Commun., 2016, 52, 6744–6764 RSC
.
-
M. Chase, NIST—JANAF Thermochemical Tables, Monograph No9, Parts I and II, 4th edn, J. Phys. Chem. Data, American Chemical Society & American Institute of Physics, Woodbury, New York, USA, 1998, 1963 pages.
-
I. Barin, Thermochemical Data of Pure Substances, Wiley-VCH, 1997 Search PubMed
.
- H. D. B. Jenkins, H. K. Roobottom, J. Passmore and L. Glasser, Inorg. Chem., 1999, 38, 3609–3620 CrossRef CAS PubMed
.
- H. D. B. Jenkins and L. Glasser, Inorg. Chem., 2003, 42, 8702–8708 CrossRef CAS PubMed
.
- L. Glasser and H. D. B. Jenkins, Thermochim. Acta, 2004, 414, 125–130 CrossRef CAS
.
- L. Glasser, Thermochim. Acta, 2004, 421, 87–93 CrossRef CAS
.
- L. Glasser and H. D. B. Jenkins, Inorg. Chem., 2011, 50, 8565–8569 CrossRef CAS PubMed
.
- L. Glasser and H. D. B. Jenkins, J. Chem. Eng. Data, 2010, 56, 874–880 CrossRef
.
- H. D. B. Jenkins, Sci. Prog., 2011, 94, 265–297 CrossRef CAS PubMed
.
- T. D. J. Dunstan and J. Caja, ECS Trans., 2007, 3, 21–32 CAS
.
- G. Annat, M. Forsyth and D. R. MacFarlane, J. Phys. Chem. B, 2012, 116, 8251–8258 CrossRef CAS PubMed
.
- O. Stolarska, A. Soto, H. Rodríguez and M. Smiglak, RSC Adv., 2015, 5, 22178–22187 RSC
.
- A. S. Ivanova, T. Brinzer, E. A. Roth, V. A. Kusuma, J. D. Watkins, X. Zhou, D. Luebke, D. Hopkinson, N. R. Washburn and S. Garrett-Roe, RSC Adv., 2015, 5, 51407–51412 RSC
.
- M. Scheuermeyer, M. Kusche, F. Agel, P. Schreiber, F. Maier, H.-P. Steinrueck, J. H. Davis, F. Heym, A. Jess and P. Wasserscheid, New J. Chem., 2016, 40, 7157–7161 RSC
.
- M. Kunze, S. Jeong, E. Paillard, M. Winter and S. Passerini, J. Phys. Chem. C, 2010, 114, 12364–12369 CAS
.
- M. Smiglak, N. J. Bridges, M. Dilip and R. D. Rogers, Chem. – Eur. J., 2008, 14, 11314–11319 CrossRef CAS PubMed
.
- P. M. Bayley, A. S. Best, D. R. MacFarlane and M. Forsyth, ChemPhysChem, 2011, 12, 823–827 CrossRef CAS PubMed
.
- O. Stolarska, H. Rodríguez and M. Smiglak, Fluid Phase Equilib., 2016, 408, 1–9 CrossRef CAS
.
- P. Bharmoria, K. Damarla, T. J. Trivedi, N. I. Malek and A. Kumar, RSC Adv., 2015, 5, 99245–99252 RSC
.
- G. B. Appetecchi, M. Montanino, M. Carewska, M. Moreno, F. Alessandrini and S. Passerini, Electrochim. Acta, 2011, 56, 1300–1307 CrossRef CAS
.
- R. Hagiwara, K. Tamaki, K. Kubota, T. Goto and T. Nohira, J. Chem. Eng. Data, 2008, 53, 355–358 CrossRef CAS
.
- K. Kubota, T. Nohira, T. Goto and R. Hagiwara, J. Chem. Eng. Data, 2008, 53, 2144–2147 CrossRef CAS
.
- G. Lambotte and P. Chartrand, J. Chem. Thermodyn., 2011, 43, 1678–1699 CrossRef CAS
.
- G. Toop, Trans. Metall. Soc. AIME, 1965, 233, 850–854 CAS
.
- A. D. Pelton and Y.-B. Kang, Int. J. Mater. Res., 2007, 98, 907–917 CrossRef CAS
.
- A. D. Pelton and P. Chartrand, Metall. Mater. Trans. A, 2001, 32, 1361–1383 CrossRef
.
- P. Chartrand and A. D. Pelton, Can. Metall. Q., 2000, 39, 405–420 CrossRef CAS
.
- E. Renaud, C. Robelin, A. E. Gheribi and P. Chartrand, J. Chem. Thermodyn., 2011, 43, 1286–1298 CrossRef CAS
.
- B. Sundman and J. Ågren, J. Phys. Chem. Solids, 1981, 42, 297–301 CrossRef CAS
.
- M. Hillert, J. Alloys Compd., 2001, 320, 161–176 CrossRef CAS
.
- M. Temkin, Acta Physicochim. URSS, 1945, 20, 411–420 CAS
.
- O. B. Babushkina, Z. Naturforsch., A: Phys. Sci., 2008, 63, 66–72 CAS
.
- M. Zawadzki, M. Krolikowska and P. Lipinski, Thermochim. Acta, 2014, 589, 148–157 CrossRef CAS
.
- R. Shannon, Acta Crystallogr., Sect. A: Cryst. Phys., Diffr., Theor. Gen. Crystallogr., 1976, 32, 751–767 CrossRef
.
- A. A. Strechan, A. G. Kabo, Y. U. Paulechka, A. V. Blokhin, G. J. Kabo, A. S. Shaplov and E. I. Lozinskaya, Thermochim. Acta, 2008, 474, 25–31 CrossRef CAS
.
- K. E. Gutowski, R. D. Rogers and D. A. Dixon, J. Phys. Chem. B, 2007, 111, 4788–4800 CrossRef CAS PubMed
.
- W. G. Meindersma, T. van Acker and A. B. de Haan, Fluid Phase Equilib., 2011, 307, 30–38 CrossRef CAS
.
- M. Larriba, P. Navarro, J.-B. Beigbeder, J. García and F. Rodríguez, J. Chem. Thermodyn., 2015, 82, 58–75 CrossRef CAS
.
- I. Bandrés, B. Giner, H. Artigas, F. M. Royo and C. Lafuente, J. Phys. Chem. B, 2008, 112, 3077–3084 CrossRef PubMed
.
- J. Benito, M. García-Mardones, V. Pérez-Gregorio, I. Gascón and C. Lafuente, J. Solution Chem., 2014, 43, 696–710 CrossRef CAS
.
- V. N. Emel’yanenko, S. P. Verevkin and A. Heintz, Thermochim. Acta, 2011, 514, 28–31 CrossRef
.
- I. Bandrés, M. C. López, M. Castro, J. Barberá and C. Lafuente, J. Chem. Thermodyn., 2012, 44, 148–153 CrossRef
.
- E. J. González, Á. Domínguez and E. A. Macedo, J. Chem. Eng. Data, 2012, 57, 2165–2176 CrossRef
.
- U. Domańska, K. Skiba, M. Zawadzki, K. Paduszyński and M. Królikowski, J. Chem. Thermodyn., 2013, 56, 153–161 CrossRef
.
- C. Cadena, Q. Zhao, R. Q. Snurr and E. J. Maginn, J. Phys. Chem. B, 2006, 110, 2821–2832 CrossRef CAS PubMed
.
- Q.-S. Liu, P.-P. Li, U. Welz-Biermann, J. Chen and X.-X. Liu, J. Chem. Thermodyn., 2013, 66, 88–94 CrossRef CAS
.
- B. Mokhtarani, A. Sharifi, H. R. Mortaheb, M. Mirzaei, M. Mafi and F. Sadeghian, J. Chem. Thermodyn., 2009, 41, 323–329 CrossRef CAS
.
- J. M. Crosthwaite, M. J. Muldoon, J. K. Dixon, J. L. Anderson and J. F. Brennecke, J. Chem. Thermodyn., 2005, 37, 559–568 CrossRef CAS
.
- S. Corderí and B. González, J. Chem. Thermodyn., 2012, 55, 138–143 CrossRef
.
- H. Guerrero, M. García-Mardones, P. Cea, C. Lafuente and I. Bandrés, Thermochim. Acta, 2012, 531, 21–27 CrossRef CAS
.
- I. Bandrés, F. M. Royo, I. Gascón, M. Castro and C. Lafuente, J. Phys. Chem. B, 2010, 114, 3601–3607 CrossRef PubMed
.
- P. Navarro, M. Larriba, J. García and F. Rodríguez, J. Chem. Thermodyn., 2014, 76, 152–160 CrossRef CAS
.
- M. Larriba, P. Navarro, J. García and F. Rodríguez, Sep. Purif. Technol., 2013, 120, 392–401 CrossRef CAS
.
- I. Bandrés, B. Giner, H. Artigas, C. Lafuente and F. M. Royo, J. Chem. Eng. Data, 2009, 54, 236–240 CrossRef
.
- I. Krossing, J. M. Slattery, C. Daguenet, P. J. Dyson, A. Oleinikova and H. Weingaertner, J. Am. Chem. Soc., 2006, 128, 13427–13434 Search PubMed
.
- K. Paduszynski and U. Domanska, Ind. Eng. Chem. Res., 2012, 51, 591–604 CrossRef CAS
.
- S. P. Verevkin, D. H. Zaitsau, V. N. Emel'yanenko, R. V. Ralys, A. V. Yermalayeu and C. Schick, Thermochim. Acta, 2013, 562, 84–95 CrossRef CAS
.
- B. Tong, Q.-S. Liu, Z.-C. Tan and U. Welz-Biermann, J. Phys. Chem. A, 2010, 114, 3782–3787 CrossRef CAS PubMed
.
- S. P. Verevkin, D. H. Zaitsau, V. N. Emel'yanenko, R. V. Ralys, C. Schick, M. Geppert-Rybczynska, S. Jayaraman and E. J. Maginn, Aust. J. Chem., 2012, 65, 1487–1490 CrossRef CAS
.
- C. W. Bale, E. Bélisle, P. Chartrand, S. A. Decterov, G. Eriksson, A. E. Gheribi, K. Hack, I. H. Jung, Y. B. Kang, J. Melançon, A. D. Pelton, S. Petersen, C. Robelin, J. Sangster, P. Spencer and M. A. Van Ende, Calphad, 2016, 54, 35–53 CrossRef CAS
.
- V. N. Emel'yanenko, S. P. Verevkin, A. Heintz and C. Schick, J. Phys. Chem. B, 2008, 112, 8095–8098 CrossRef PubMed
.
- G. J. Kabo, Y. U. Paulechka, A. G. Kabo and A. V. Blokhin, J. Chem. Thermodyn., 2010, 42, 1292–1297 CrossRef CAS
.
- V. N. Emel'yanenko, G. J. Kabo and S. P. Verevkin, J. Chem. Eng. Data, 2006, 51, 79–87 CrossRef
.
- E. S. Domalski and E. D. Hearing, J. Phys. Chem. Ref. Data, 1993, 22, 805–1159 Search PubMed
.
- Z. H. Zhang, L. X. Sun, Z. C. Tan, F. Xu, X. C. Lv, J. L. Zeng and Y. Sawada, J. Therm. Anal. Calorim., 2007, 89, 289–294 CrossRef CAS
.
|
This journal is © the Owner Societies 2018 |
Click here to see how this site uses Cookies. View our privacy policy here.