DOI:
10.1039/C7AY02283B
(Paper)
Anal. Methods, 2018,
10, 101-108
Development of molecularly imprinted photonic polymers for sensing of sulfonamides in egg white
Received
25th September 2017
, Accepted 26th November 2017
First published on 27th November 2017
Abstract
We developed a simple method based on molecularly imprinted photonic polymers (MIPPs) for sensing of sulfonamides in egg white samples. The fabrication of MIPPs mainly involved a photonic crystal template method integrated with a molecular imprinting technique. Firstly, an opal photonic crystal template was self-assembled from monodisperse polystyrene colloids. And then the template was embedded with molecularly imprinted polymers, which was synthesized with acrylic acid and acrylamide as monomers, N,N′-methylenebisacrylamide as a cross-linker and sulfamerazine (SM1) or sulfamethazine (SM2) as imprinting molecules. After removal of the photonic crystal template and the imprinted molecules, the resultant MIPP sensor accommodated an inverse opal photonic crystal structure embedded in hydrogels. By using the as-prepared MIPP sensor, sulfonamides were sensitively and specifically recognized via a molecular imprinting technique and the recognition was detected through the readable Bragg diffraction shift from the photonic crystal structure. A 70 nm maximum Bragg diffraction blue-shift of the MIPP was observed with the concentrations of the sulfamerazine or sulfamethazine varying from 3.8 μM to 22.8 μM and function relationships were found between blue-shifts and the concentrations of sulfonamides in pH 5 acetic acid–sodium acetate buffer solution. The developed method has been applied successfully to detect sulfonamides in egg white samples without using labelling techniques and expensive instruments.
1 Introduction
Molecular imprinting technology is a powerful tool in the development of highly selective analytical methods, which allows the synthesis of polymers with selectivity towards a predetermined target molecule.1,2 The common approach for synthesis of molecularly imprinted polymers (MIPs) mainly involves polymerization of functional monomers with cross-linkers around target molecules. After the target molecules are removed, specific cavities with both morphological and stereochemical (such as size, shape and chemical functionality) recognition sites are formed through the molecular imprinting process.3 MIPs possess several advantages including low cost, ease of preparation, storage stability, repeated operations without loss of activity, high mechanical strength, durability to heat and pressure, and applicability in harsh chemical media.4 Because of such advantages, molecular imprinting techniques have drawn much attention of researchers in various fields, and to date, extensive studies on MIPs have been reported such as artificial receptors in sample preparation,5–13 and drug analysis,5,7,9 as well as chemical sensors and biosensors.4,11–13 Especially in the sensor field, MIP-based sensors have made considerable progress and become a well-established procedure for preparing artificial recognition units instead of natural receptors.4
The main advantage of a MIP-based sensor is that it can not only specifically bind target analytes as a recognition element, but also can generate output signals for detection as a transduction element in the sensor system. In traditional MIP assays, analytes should contain chromophores or fluorophores, or be electroactive to generate readable optical or electronic signals. Otherwise, the target analytes need to be modified or tagged. In the past few years, substantial efforts have been made to explore new, multi-functional and more easily operated MIP-based sensors.14 Among them, molecularly imprinted photonic polymers (MIPPs), which involve the formation of complexes between the imprinting molecule and hydrogel functional monomers on photonic crystal templates, have emerged as a sensing platform to achieve direct detection and quantification of analytes through readable optical signals to target rebinding events in the molecular imprinting process.15–28 MIPPs were first introduced and then have been further developed by Li's group.15–19 Their achievements including recognitions of L-dopa,15 proteins,16 cholic acid,17 alkaloids18 and atrazine19 have demonstrated that MIPPs are practicable in sensor applications. In the previous work, MIPPs are commonly prepared by using closest-packing photonic crystals as templates. Pre-polymerization solutions including hydrogel monomers and imprinting molecules are infused and polymerized in the voids of the photonic crystal templates during the preparation process. After removal of photonic crystal templates and imprinting molecules, the prepared MIPPs accommodate a highly-ordered three-dimensional (3D) inverse opal photonic crystal structure and an interconnected macropore array with a thin hydrogel wall. Due to their special structure, MIPP sensors are endowed with specific recognition properties derived from imprinted materials, as well as exhibiting rapid swelling or shrinkage in volume responding to chemical stimuli arising from the analyte-sensitive hydrogel polymer. Meanwhile, the volume change of the 3D ordered photonic polymer film can give rise to interesting optical properties such as the Bragg diffraction shift, which can be used to optically determine analytes. Therefore, MIPPs can exhibit a specific, sensitive and quick response to target analytes, and simultaneously achieve direct detection without using any labelling techniques and expensive instruments.
In our previous work, we developed a label-free spectral sensor based on MIPPs for sensing tetracyclines in a food matrix.20 A linear relationship was found between the Δλ and the concentration of tetracyclines in the range of 0.04 μM to 0.24 μM. Using the developed method, direct and selective detection of tetracyclines has been achieved and successfully applied to detect tetracycline in milk and honey samples. In general, the performances of a MIPP are dependent on the initial choice of polymerizable ingredients and the reaction conditions that are used to synthesize the polymer. Thus it is required to find a suitable system for every template molecule to make acceptable cavities yet easily cleavable to achieve fast and easy extraction of the template from the network. In this study, we have focused on developing a MIPP sensor for detecting sulfonamides in some food samples such as egg white. To the best of our knowledge, there is no article focused on the analysis of sulfonamides using methods based on photonic crystal sensor or MIPP sensor technology. Sulfonamides are antibiotic agents which play important roles in both human and veterinary medicines to fight common bacterial diseases. But the presence of sulfonamides in food products becomes a continuing health issue because studies have shown that one or more members of this family are suspected carcinogens.29–32 Due to the potential hazard of these compounds, many countries have adopted a maximum acceptable limit of residual sulfonamides in animal origin foods. For example, the European Union (EU) has set a maximum residue limit at 100 ng g−1 for total sulfonamides in edible animal tissues, including poultry.33 In egg, where no corresponding residue limits were set, sulfonamides are “zero tolerance” substances. Hence, it is important to establish effective methods for the determination of sulfa drug residues in edible animal tissue. Here, sulfonamide imprinted MIPPs were prepared and their application for the determination of sulfonamide residues in egg white was investigated. We carefully optimized the conditions of polymerization and template removal for preparing the MIPP, and proposed the possible mechanism of the imprinting process of MIPPs to sulfonamides, which were different from our previous work and the work of other groups. The developed method has been applied to the analysis of sulfonamide residues in egg white samples.
2 Materials and methods
2.1 Materials
Sulfamerazine (SM1) and sulfamethazine (SM2) were purchased from Alfa Aesar. Acetic acid, sodium acetate, styrene, sodium dodecyl sulfonate (SDS), ammonium persulfate (APS), acrylamide (AMD), acrylic acid (AA), N,N′-methylenebisacrylamide (BIS), methanol and other affiliated chemicals were all purchased from local suppliers. Styrene was vacuum distilled to remove inhibitors prior to being used, and other solvents and chemicals were of reagent quality and were used without further purification unless otherwise specified. Common glass slides (50 × 20 mm) were immersed in a H2SO4–H2O2 mixture for 12 hours, followed by rinsing with deionized water in an ultrasonic bath three times and then dried for use. All containers for photonic crystal growth were cleaned by rinsing with a H2SO4–H2O2 mixture and deionized water.
Buffer solution was prepared using 0.1 M acetic acid and 0.1 M sodium acetate. When used, the pH was adjusted by adding additional acetic acid or sodium acetate solution. The molecular structures of sulfamerazine and sulfamethazine are shown in Fig. 1. Standard solutions of sulfonamides (10 mg L−1) were prepared in acetonitrile and maintained at 4 °C in a refrigerator. These standard solutions were then further diluted with the acetic acid–sodium acetate buffer solution to yield appropriate working solutions.
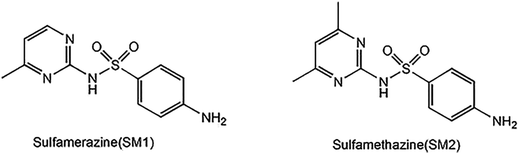 |
| Fig. 1 Molecular structures of the two investigated sulfonamides. | |
2.2 Preparation of monodisperse polystyrene colloids
The monodisperse polystyrene colloids with different diameters were synthesized by emulsion polymerization in the same way as ref. 20, 34 and 35. In brief, 0.25 g APS, 0.125 g SDS, 0.0125 g sodium hydroxide and 100 mL deionized water were mixed in a 500 mL flask, under intensive stirring with nitrogen to degas, and 25 mL styrene were slowly added with a constant pressure dropping funnel. Monodisperse polystyrene particles of about 200 nm were obtained after overnight reaction.
2.3 Preparation of polystyrene photonic crystal templates
Templates of photonic crystals were prepared from the as-obtained monodisperse polystyrene colloids by using the vertical deposition method.20,34,35 Polystyrene colloids particles in aqueous media with a concentration of about 5% (v/v) were placed into treated containers. Treated glass slides were put into containers vertically for photonic crystal growth. Containers were laid in a 43 °C water bath for 2–3 days. After the solvent was evaporated completely, templates of the close-packed face-centered-cubic (fcc) photonic crystal were obtained.
2.4 Preparation of sulfonamide MIPPs
To prepare photonic hydrogel films, acrylamide and acrylic acid were mixed with sulfonamides to form interaction. A SM1 imprinted photonic polymer (MIPP1) and SM2 imprinted photonic polymer (MIPP2) were prepared by the following steps. First, 0.017 g sulfonamides, 3.0 g AMD, 1.4 mL AA and 0.09 g BIS were mixed fully in 4 mL deionized water by ultrasonic vibration for 10 minutes to ensure sufficient complexation and 0.08 g APS was added as an initiator. Then the homogeneous monomer mixture was dropped onto the polystyrene photonic crystal template by using a capillary-attraction-induced method until the template became transparent, indicating successful infiltration. Polymerization was performed in an oven at 60 °C for 3 hours. Afterwards, the obtained polymer film was immersed in xylene to etch the polystyrene spheres from photonic crystal templates, and then rinsed with anhydrous ethanol and deionized water three times. Finally, the embedded sulfonamide molecules were removed by immersing the polymer film in an acetic acid/methanol (1
:
9 v/v) mixture containing 5% SDS (w/v) in a bath oscillator at 60 °C until no further diffraction changes were observed, which indicates full removal of sulfonamides from the imprinted films. Thus the MIPP macroporous materials with an inverse opal structure imprinted by sulfonamides were obtained. For control experiments, a non-imprinted photonic hydrogel (NIPP) film was also prepared using the same procedure and conditions only without sulfonamide molecules.
Surface morphologies of the used templates and the imprinted films were observed by using a field-emission scanning electron microscope (JEOL JSM-6700, Japan) operating at 25 kV.
2.5 Detecting responses of MIPPs to sulfonamides
The optical responses of the MIPP film to sulfonamides were measured in sulfonamide aqueous solutions using a 380–1050 nm fiber optic spectrometer (JKHQ-D1, Tianjin, China) in the vertical direction. In our experiments, always a maximum diffraction peak was observed and recorded. Before each spectral scan, the pH of all solutions was checked and the final value was controlled at about pH 5 using acetic acid–sodium acetate buffer. In all cases, the swelling equilibrium of the MIPP film would be reached after being immersed in 10 mL buffer solutions for 5 minutes. After one detection, the film was immersed in an acetic acid/methanol (1
:
9 v/v) mixture containing 5% SDS (w/v) for another 30 minutes and thoroughly rinsed with deionized water to recover the blank state for the next detection. For a series of sulfonamide concentrations, the detection followed the sequence from low to high concentrations to eliminate interference.
2.6 Sample detection
1 g egg white dispersed in 100 mL buffer solution was prepared as a blank sample without further treatment but adjusting the pH value to 5. The SM1 mixed egg white sample was prepared by mixing 7.6 μM SM1 standard solution with the blank sample and the total volume was 10 mL. The diffraction spectrum of MIPP1 in pure buffer solution was first measured as a control experiment. Then the diffraction spectrums of MIPP1 in the blank and mixed samples were measured by immersing MIPP1 in the blank and mixed samples at room temperature for 5 minutes, respectively. To demonstrate the reproducibility of the sample detection, the SM1 mixed egg white sample was analyzed seven times and the RSD was calculated from the results.
3 Results and discussion
3.1 Fabrication of a sulfonamide imprinted photonic polymer film
Sulfamerazine (SM1) and sulfamethazine (SM2), which are structurally similar (Fig. 1), were chosen as the target molecules since they are the two common members of sulfonamides. MIPP1 and MIPP2 were prepared using SM1 and SM2 as the imprinted template, respectively. The sulfonamide imprinted photonic polymers were prepared by the following steps. First, photonic crystal templates were prepared using the vertical deposition method followed by infiltrating the liquid precursors and the complex of sulfonamides with monomers into the voids of photonic crystal templates by the capillary-attraction-induced method. Then, polymerization was performed before removing the used photonic crystal templates and imprinted molecule template. Backfilling the template materials at room temperature and removal without pattern collapse were critical because it was possible to introduce additional defects during each of the processing steps. In this work, all the parameters involving the preparation of monodisperse polystyrene particles and polystyrene photonic crystal templates, infiltration of precursors, polymerization and the subsequent templates removal process were simultaneously considered and investigated in detail to get the optimal conditions.
Photonic crystal template methods have been extensively studied as routes to prepare photonic band gap materials.36,37 Herein, monodisperse polystyrene particles with a diameter of about 200 nm (Fig. 2a) were used to form photonic crystal templates with a highly ordered closed packing structure. Fig. 2b shows the SEM of the polystyrene photonic crystal template filled with the polymer without etching the two templates, which showed a rather perfect face-centered cubic (fcc) close-packed structure with the (111) plane parallel to the substrate surface. To produce a well-defined optical signal of Bragg diffraction of the photonic crystal structure hydrogel film, a three-dimensional, ordered and interconnected macroporous structure is the precondition and photonic crystal templates and imprinted molecules should be etched completely. Fig. 2c shows the 3D-ordered macroporous structure of the obtained MIPP film after removing the photonic crystal template and the imprinted template. As expected, it could be seen from the SEM images that the MIPP film maintains an inverse opal structure with 3D ordered and interconnected macropores after eluting polystyrene spheres and sulfonamide template molecules.
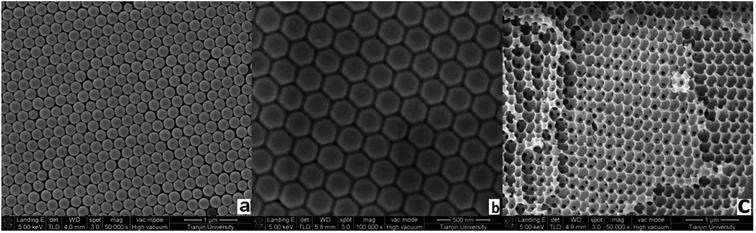 |
| Fig. 2 Typical SEM images of (a) the used monodisperse polystyrene particles, (b) the polystyrene photonic crystal template filled with the polymer without etching the two templates, and (c) the resultant MIPP film with a 3D ordered macroporous structure. | |
3.2 Sensing properties of MIPPs to sulfonamides
For the purpose of application, it is generally necessary to consider the main parameters of MIP-based sensors such as the response time, linear response range and selectivity.
In our present work, the increased surface-to-volume ratio due to the 3D ordered macroporous structure endowed the MIPP film with a rapid response to external stimuli, which would also be directly transferred into readable optical signals because of the Bragg diffraction shift. In every experiment, an immersion time of 5 minutes for any concentration of sulfonamides was sufficient for diffusion and swelling equilibrium to occur.
The sensing behaviors of MIPP1 and MIPP2 immersed in SM1 and SM2 solutions of a series of concentrations at pH 5 were investigated respectively. The trend of the diffraction peak shift of the SM1 imprinted photonic polymer MIPP1 responding to a series of concentrations of SM1 is shown in Fig. 3. The original Bragg diffraction wavelength of MIPP1 in pure acetic acid–sodium acetate buffer solution is at 645 nm, which is used as control in the experiment. Then, after being immersed into 3.8 μM SM1 solution for 5 minutes, MIPP1 displays a diffraction blue-shift of more than 10 nm, which indicates that MIPP1 is sensitive to the rebinding of SM1 molecules. With the increase of the concentration of SM1, the diffraction peak of MIPP1 has a gradual blue-shift with the concentration of SM1 solution increasing from 3.8 μM to 22.8 μM, and the total blue-shift reaches about 70 nm when the SM1 concentration is 22.8 μM (Fig. 3a). The linear relationship between the diffraction peak blue-shift of a MIPP (Δλ) and the concentration of SM1 has not been obtained throughout the investigated concentration range. For SM1, a linear relationship between Δλ and the concentration of SM1 was found in the range from 3.8 μM to 15.2 μM, and a linear equation Δλ = 4.94c − 5.47 with an R2 of 0.93 was obtained (Fig. 3b).
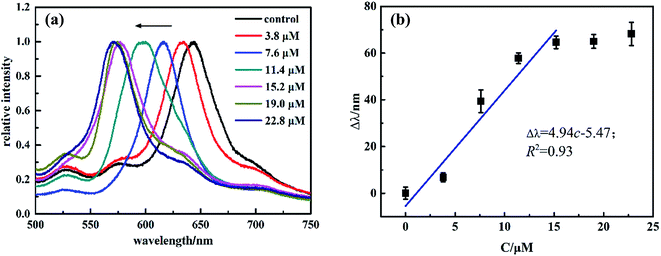 |
| Fig. 3 (a) Diffraction response of MIPP1 to SM1 of varying concentrations and (b) relationship between the blue-shift of the diffraction peak (Δλ) and the concentrations of SM1. | |
The sensing behaviors of MIPP2 to SM2 were also studied in the same way as MIPP1. It has also been found that the blue-shift of MIPP2 increases gradually with the concentration of SM2. In theory, the diffraction shift of the MIPP2 film should be a function of the concentration of SM2 because the hydrogel volume change usually is a function of analyte concentration. The diffraction response of MIPP2 to SM2 of varying concentrations is shown in Fig. 4. As expected, the Bragg diffraction peak of MIPP2 exhibits regular blue-shift in SM2 solution with the concentration of SM2 solution increasing from 3.6 μM to 21.6 μM (Fig. 4a). A linear equation Δλ = 3.92c − 0.09 with an R2 of 0.99 has been obtained in the range from 3.6 μM to 18.0 μM (Fig. 4b).
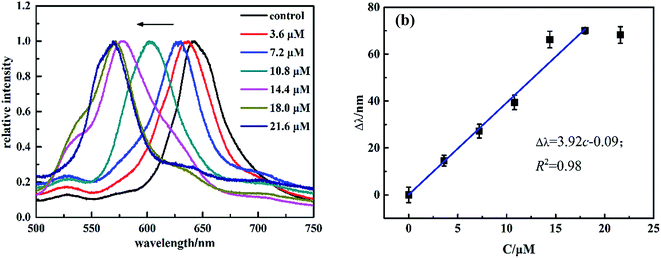 |
| Fig. 4 (a) Diffraction response of MIPP2 to SM2 of varying concentrations, (b) relationship between the blue-shift of the diffraction peak (Δλ) and the concentrations of SM2. | |
Molecularly imprinted polymers based on acrylic type polymers exhibit high selectivity and affinity towards the target compound. To elucidate the molecular recognition properties of the imprinted materials, the optical response of the non-molecularly imprinted photonic polymer (NIPP) film without sulfonamides added was investigated in various concentrations of sulfonamides. As a control experiment, experiments using the NIPP are performed in the same way as those using the MIPP. The optical responses of the NIPP film to a series of concentrations of SM1 are shown in Fig. 5. It is observed that there are only slight and irregular shifts of the Bragg diffraction peak with the increasing concentration of SM1 in the case of the NIPP.
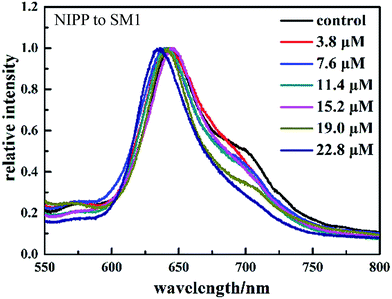 |
| Fig. 5 Diffraction response of the NIPP to SM1 of varying concentrations. | |
In order to further reveal the sensing specificity of the molecular imprinting process, the responses of MIPP1 and MIPP2 to the non-imprinted molecular polymer were examined. Under the same conditions, when MIPP1 was immersed into SM2 solution for 5 minutes, no obvious diffraction shift can be observed compared to the original Bragg diffraction wavelength of MIPP1 in pure acetic acid–sodium acetate buffer solution (Fig. 6a). Similarly, the diffraction peak of MIPP2 exhibits no significant shift to SM1 (Fig. 6b), which demonstrates that MIPP2 is not sensitive to the rebinding of SM1 molecules.
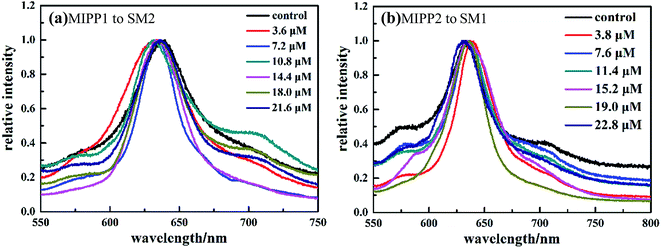 |
| Fig. 6 Diffraction response of (a) MIPP1 to SM2 and (b) MIPP2 to SM1 of varying concentrations. | |
So far, our results demonstrated that the microenvironments created by molecular imprinting were responsible for the observed responses of MIPP1 to SM1 as well as MIPP2 to SM2, which elucidated that molecular imprinting played a key role in the specific recognition of the MIPP film by the target molecule. By using the as-prepared MIPP sensor, sulfonamides could be sensitively and specifically recognized via a molecular imprinting technique and the recognition could be detected through the readable Bragg diffraction shift from the photonic crystal structure.
3.3 Recoverability
In our present work, the above-prepared MIPP sensor film was robust enough to go through a series of tests and a single MIPP film was used throughout the investigation to minimize variations and errors. Nevertheless, the recoverability of the MIPP sensor was still an important concern of the measurement accuracy because the MIPP sensor film was required to be immersed in the test solution, followed by washing with the acetic acid/methanol/SDS mixture and deionized water for several cycles. To examine the recoverability, the MIPP1 sensor film was firstly immersed in the pH 5 acetic acid–sodium acetate buffer solution to reach a stable blank state, and then immersed in 22.8 μM SM1 solution to show responses to analytes. It was experimentally demonstrated that the diffraction peak had a blue-shift from 645 to 577 nm in the presence of SM1. After recording, the film was taken out and immersed in an acetic acid/methanol (1
:
9 v/v) mixture containing 5% SDS (w/v) for 30 minutes and rinsed thoroughly with deionized water for the next cycle. We have experimentally examined the diffraction peak variations of the MIPP1 sensor over five cycles. As presented in Fig. 7, the diffraction wavelength of the MIPP1 sensor recovered back near to the original value after it was washed with the acetic acid/methanol/SDS mixture and deionized water, which indicated that the diffraction response of the MIPP1 sensor was not affected over five cycles. Moreover, we have also demonstrated that the MIPP sensor film was allowed to dry and rehydrated while retaining its diffraction and sensing properties. The MIPP sensor had such a good recoverability which resulted from the good reversible swelling and shrinking characteristics of hydrogel polymers.
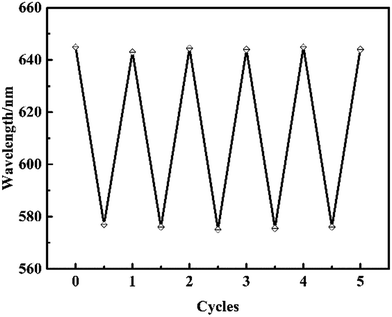 |
| Fig. 7 Diffraction response of the MIPP1 film from pH 5 acetic acid–sodium acetate buffer solution to 22.8 μM SM1 solution over five cycles. | |
3.4 Mechanism of the imprinting process of the MIPP
Our former research has proven that the functional monomer acrylic acid in the polymerized MIP and the template tetracycline molecule can form hydrogen bonds, which proves the selective recognition structure for tetracycline after removing the template tetracycline molecule.20 In this work, it also was deduced that non-covalent hydrogen bonding-based molecular imprinting of sulfonamide template molecules with acrylamide and acrylic functional monomers occurs in the pre-polymerization stage. Fig. 8 illustrates the probable formation of the imprinted sites in the MIPP for sulfonamides. The formation of hydrogen bonds between the monomers and sulfonamide template molecule provided the prerequisite for producing a larger number of specific binding sites by using the MIPP imprinting technique. In the rebinding process, binding sites formed in the MIPP not only involved reestablishment hydrogen bonds among amine, amido, carboxyl and hydroxyl groups, but also included a formation of hydrogen bonds between sulfonyl oxygen with hydroxyl and amine groups.38 The number of complementary template-functional monomer interactions was large, which made the binding and fidelity of recognition of the MIPP stronger. In addition, because there was a rather high degree of cross-linking in the recognition cavity structures, the volume of the hydrogel film shrank and induced a blue-shift of the diffraction wavelength.
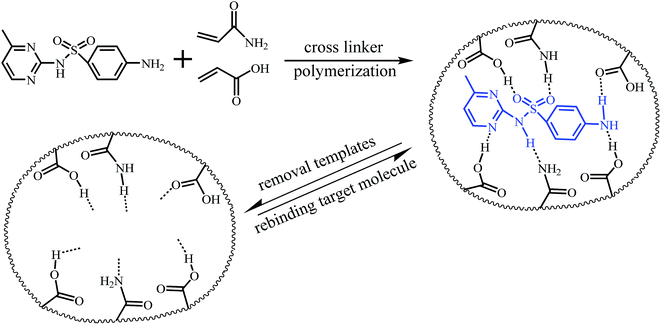 |
| Fig. 8 Schematic illustration of molecular imprinting binding sites formation in MIPPs for recognition of sulfonamides. | |
3.5 Applications
The developed method was validated by determining egg white samples which were mixed with 7.6 μM SM1. After MIPP1 was immersed in the sample for 5 minutes, no obvious shift of its diffraction peak was observed in the blank sample compared to that in pure buffer solution. However, there was a significant diffraction peak blue-shift of MIPP1 in SM1 mixed samples (as shown in Fig. 9). According to the linear equation of MIPP1 to SM1 in the range from 3.8 μM to 15.2 μM, it could be calculated that the content of SM1 in the mixed samples was about 7.3 μM. The relative standard deviation (RSD) of the diffraction peak shift for seven successive tests of MIPP1 in SM1 mixed egg white was 3%. The application of the present method makes the detection of significant amounts of persistent sulfonamide residuals in liquid food samples possible.
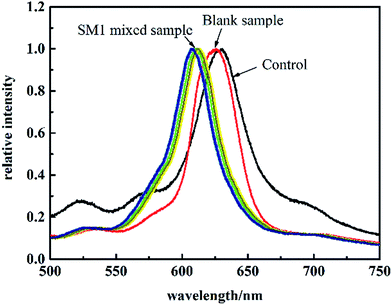 |
| Fig. 9 Diffraction response of MIPP1 in the blank sample and SM1 mixed egg white sample. | |
4 Conclusions
A method for the determination of residual sulfonamides in egg white was established using MIPPs as a sensor. Our results showed that the diffraction peak shift of the photonic crystal material was pretty large (about 70 nm at maximum), which means there is a great possibility that a visual detection method can be developed for sulfonamides in egg white by color change based on the present method. Compared with conventional screening methods in food and many other analytical fields, photonic crystal materials open new possibilities towards the development of smart optical sensing devices.
Conflicts of interest
There are no conflicts to declare.
References
- L. X. Chen, X. Y. Wang, W. H. Lu, X. Q. Wu and J. H. Li, Chem. Soc. Rev., 2016, 45, 2137–2211 RSC.
- P. L. Wang, X. H. Sun, X. O. Su and T. Wang, Analyst, 2016, 141, 3540–3553 RSC.
- L. Ye, Anal. Bioanal. Chem., 2016, 408, 1727–1733 CrossRef CAS PubMed.
- L. Uzun and A. P. F. Turner, Biosens. Bioelectron., 2016, 76, 131–144 CrossRef CAS PubMed.
- H. Zhang, L. Ye and K. Mosbach, J. Mol. Recognit., 2016, 19, 248–259 CrossRef PubMed.
- T. Kubo and K. Otsuk, Trends Anal. Chem., 2016, 81, 102–109 CrossRef CAS.
- S. Ansari and M. Karimi, Talanta, 2017, 164, 612–625 CrossRef CAS PubMed.
- A. Sarafraz-Yazdi and N. Razavi, Trends Anal. Chem., 2015, 73, 81–90 CrossRef CAS.
- S. Ansari and M. Karimi, Trends Anal. Chem., 2017, 89, 146–162 CrossRef CAS.
- Y. L. Hu, J. L. Pan, K. Zhang, H. X. Lian and G. K. Li, Trends Anal. Chem., 2013, 43, 37–52 CrossRef CAS.
- R. I. Boysen, L. J. Schwarz, D. V. Nicolau and M. T. W. Hearn, J. Sep. Sci., 2017, 40, 314–335 CrossRef CAS PubMed.
- J. Ashley, M. A. Shahbazi, K. Kant, V. A. Chidambara, A. Wolff, D. D. Bang and Y. Sun, Biosens. Bioelectron., 2017, 91, 606–615 CrossRef CAS PubMed.
- G. Ertürk and B. Mattiasson, Sensors, 2017, 17, 288–305 CrossRef PubMed.
- M. J. Whitcombe, N. Kirsch and I. A. Nicholls, J. Mol. Recognit., 2014, 27, 106–180 CrossRef PubMed.
- X. B. Hu, Q. An, G. T. Li, S. Y. Tao and B. Liu, Angew. Chem., Int. Ed., 2006, 45, 8145–8148 CrossRef CAS PubMed.
- X. B. Hu, G. T. Li, J. Huang, D. Zhang and Y. Qiu, Adv. Mater., 2007, 19, 4327–4332 CrossRef CAS.
- Z. Wu, X. B. Hu, C. A. Tao, Y. Li, J. Liu, C. D. Yang, D. Z. Shen and G. T. Li, J. Mater. Chem., 2008, 18, 5452–5458 RSC.
- X. B. Hu, G. T. Li, M. H. Li, J. Huang, Y. Li, Y. B. Gao and Y. H. Zhang, Adv. Funct. Mater., 2008, 18, 575–583 CrossRef CAS.
- Z. Wu, C. A. Tao, C. X. Lin, D. Z. Shen and G. T. Li, Chem.–Eur. J., 2008, 14, 11358–11368 CrossRef CAS PubMed.
- L. Q. Wang, F. Y. Lin and L. P. Yu, Analyst, 2012, 137, 3502–3509 RSC.
- J. H. Li, Z. Zhang, S. F. Xu, L. Q. Chen, N. Zhou, H. Xiong and H. L. Peng, J. Mater. Chem., 2011, 21, 19267–19274 RSC.
- C. H. Zhou, T. T. Wang, J. Q. Li, C. Guo, Y. Peng, J. L. Bai, M. Liu, J. W. Dong, N. Gao, B. A. Ning and Z. X. Gao, Analyst, 2012, 137, 4469–4474 RSC.
- F. Xue, Z. H. Meng, Y. F. Wang, S. Y. Huang, Q. H. Wang, W. Lu and M. Xue, Anal. Methods, 2014, 6, 831–837 RSC.
- Z. K. Yang, D. J. Shi, M. Q. Chen and S. R. Liu, Anal. Methods, 2015, 7, 8352–8359 RSC.
- J. Hou, H. C. Zhang, Q. Yang, M. Z. Li, L. Jiang and Y. L. Song, Small, 2015, 11, 2738–2742 CrossRef CAS PubMed.
- Y. N. Zhang, S. M. Huang, C. T. Qian, Q. Z. Wu and J. F. He, J. Appl. Polym. Sci., 2016, 133, 43191–43199 Search PubMed.
- A. M. You, Y. H. Cao and G. Q. Cao, RSC Adv., 2016, 6, 83663–83667 RSC.
- W. Lu, S. A. Asher, Z. H. Meng, Z. Q. Yan, M. Xue, L. L. Qiu and D. Yi, J. Hazard. Mater., 2016, 316, 87–93 CrossRef CAS PubMed.
- S. L. Qin, L. Q. Su, P. Wang and Y. Gao, Anal. Methods, 2015, 7, 8704–8713 RSC.
- L. J. Qiao, X. H. Zhou, Y. H. Zhang, J. Yu, S. S. Zhang and Y. J. Wu, Anal. Methods, 2016, 8, 6099–6106 RSC.
- E. Peris-García, M. T. Ubeda-Torres, M. J. Ruiz-Angel and M. C. García-Alvarez-Coque, Anal. Methods, 2016, 8, 3941–3952 RSC.
- V. Gaudin, Biosens. Bioelectron., 2017, 90, 363–377 CrossRef CAS PubMed.
- S. G. Dmitrienko, E. V. Kochuk, V. V. Tolmacheva, V. V. Apyari and Y. A. Zolotov, Food Chem., 2015, 188, 51–56 CrossRef CAS PubMed.
- F. Y. Lin and L. P. Yu, Anal. Methods, 2012, 4, 2838–2845 RSC.
- Y. X. Zhang, P. Y. Zhao and L. P. Yu, Sens. Actuators, B, 2013, 181, 850–857 CrossRef CAS.
- D. Men, D. Liu and Y. Li, Sci. Bull., 2016, 61, 1358–1371 CrossRef CAS.
- G. von Freymann, V. Kitaev, B. V. Lotsch and G. A. Ozin, Chem. Soc. Rev., 2013, 42, 2528–2554 RSC.
- D. A. Adsmond and D. J. W. Grant, J. Pharm. Sci., 2001, 90, 2058–2077 CrossRef CAS PubMed.
|
This journal is © The Royal Society of Chemistry 2018 |