DOI:
10.1039/C7AY02232H
(Paper)
Anal. Methods, 2018,
10, 145-150
Plasmonic nanograting enhanced fluorescence for protein microarray analysis of carcinoembryonic antigen (CEA)†
Received
18th September 2017
, Accepted 28th November 2017
First published on 29th November 2017
Abstract
The low sensitivity of protein microarrays has limited the wide application in diagnostics and high-throughput proteomic analysis. We present, for the first time, the exploration of protein microarray analysis on nanostructured plasmonic gold nanograting that showed a 10-fold fluorescence enhancement. The as-fabricated plasmonic protein microarray showed a limit of detection (LOD) of 0.36 ng mL−1 with the dynamic detection range of 1 ng mL−1 to 100 μg mL−1 for detecting the cancer biomarker of carcinoembryonic antigen (CEA). The LOD determined in this study is one order lower than that of the commercial planar glass substrates, which is attributed to the surface plasmon field enhanced fluorescence (SPFS) from the plasmonic coupling. The highly sensitive and wide dynamic detection range of plasmonic microarrays presents new opportunities in proteomic applications and diagnostics research.
1. Introduction
Microarray based analysis is becoming an important research tool for broad applications in biotechnological and biomedical fields because of the advantages of fast and high-throughput assays, the small volume of sample consumption, and the parallel analytical capacity.1–3 It shows significant advantages over the conventional methods of enzyme-linked immunosorbent assays (ELISAs),4,5 electrochemical analyses,6,7 label-free optical methods,8,9 and field-effect transistors10 for rapid and multiplexed analysis.11,12 Despite the wide applications in bioscience,13 disease diagnostics14 and drug discovery,15 the challenge of limited sensitivity persists because of the low available quantity of signal molecules for binding.16 The lack of an efficient magnification method such as polymerase chain reaction (PCR) in gene microarray hinders the important applications of protein microarrays for diagnosis with high sensitivity.17 A series of classic methods including rolling circle amplification (RCA)18,19 enzymatic catalysis,20,21 and gold nanomaterial-assisted scanometric experiment22,23 have been developed to increase the sensitivity of protein microarrays in the past decades. Enzyme-catalyzed deposition or enzyme-linked chemiluminescence both provide a low limit of detection (LOD), but the former strategy shows nonlinearity at high analyte concentrations and the latter strategy requires the use of specific instruments.20 Both strategies suffer from volatility and high-cost of reagents and enzymes.24 The RCA strategy is suitable for protein detection, but it is complicated with a poor target concentration response.18 There is an enormous demand for an alternate, cheap and convenient strategy to increase the sensitivity of protein microarrays.
Fluorescence detection is usually used for protein microarray, showing the advantages of being sensitive, quick, convenient and comparatively cheap.25 The traditional organic dye-labeling suffers from the difficulty of obtaining sufficient low LOD because of the low quantum yields and the limited dye-to-reporter ratio. Researchers have paid increasing attention to explore new nanomaterials with particular optical properties, for instance quantum dots (QD), metallic nanoparticles and fluorescent silica nanomaterials to improve the sensitivity of fluorescence detection.26–29 These new nanomaterials have shown outstanding benefits for the detection of protein molecules with high sensitivity for fluorescence-based protein microarrays.30–34 Plasmonic materials based on noble metallic nanostructures show unique optical properties for fluorescence enhancement and chemically catalytic applications.35–41 Our previous studies showed that silver-based plasmonic nanogratings are effective for fluorescence enhancement detection and cell observation.37,38 Unfortunately, the nanogratings suffered from poor stability of the silver film in a phosphate buffer saline solution environment.42,43 There is a requirement to develop stable plasmonic nanogratings for the application of protein microarray detection with high fluorescence enhancement.
In this study, we developed the gold-based one-dimensional (1D) plasmonic nanograting as a substrate for the fluorescence enhancement of protein microarray analysis. A current read-out method was applied to the biomolecular immunoassay.44 The substrate exhibited a 10-fold fluorescence amplification compared to the bare glass substrates, showing the prominent advantages of suitability for mass production, polyfunctionality and repeatability for various fluorophores. A low detection limit of 0.36 ng mL−1 and the wide dynamic detection range of 1 ng mL−1 to 100 μg mL−1 were successfully achieved for analyzing a typical biomarker, carcinoembryonic antigen (CEA). This research illustrates the potential application of plasmonic nanograting for protein microarray analysis.
2. Experimental section
2.1. Materials and chemicals
Quartz slides with the thickness of 1 mm (Fisher Scientific Corp., China) were used as substrates to manufacture grating structures and gold films. The commercial epoxide activated slides were purchased from CapitalBio Corp. (Beijing, China). (3-Glycidoxypropyl)trimethoxysilane (GPTS), glycerol, Triton® X-100, phosphate buffered saline (PBS, 0.01 M, pH 7.4) and Tris buffered saline (TBS, 0.05 M, pH 8.0) containing Tween® 20 were obtained from Sigma-Aldrich Corp. (USA). Cy5-labeled IgG (Cy5-IgG) were ordered from Biosynthesis Biotechnology Co., Ltd. (Beijing, China). The target biomarker the CEA antigen (CEA-Ag), capture antibody CEA monoclonal antibody (CEA-mAb), recognition antibody CEA polyclonal antibody (CEA-pAb), and Cy5-labelled secondary antibody were obtained from Fitzgerald Industries International (USA). All the reagents were used as received. The other chemicals were of analytical grade. The deionized water (DI water, 18.2 MΩ cm) used in all experiments was produced using a water purification system (Q-Grad® Millipore Corp., USA).
2.2. Preparation of SiO2 1D grating structures
All the flat quartz slides were sonically cleaned in 2% Hellmanex III solution (Sigma-Aldrich, USA) for a span of 20 min and then exhaustively rinsed under DI water and ethanol and then completely dried under air flow. Then, the cleaned slides were constructed under a standard two light beam interference strategy, followed by a dry etching procedure as described in our previous literatures.42,45
2.3. Plasmonic multilayer construction on quartz 1D gratings
The quartz 1D gratings were consequently metalized with multilayers of Cr (2 nm)/Au (150 nm)/Cr (1 nm)/SiO2 (40 nm) using the radio frequency (RF) sputtering machine (JGP450A, China). A layer of Cr (less than 2 nm) was deposited in order to increase the adhesion of gold on the quartz substrate. A gold film with thickness of 150 nm was sputtered, followed by another thinner layer of Cr, and a subsequent 40 nm-thick layer of SiO2. The total thickness of Cr/Au/Cr/SiO2 plasmonic layer is about 193 nm. The quartz slides were also coated with the same layers. Coated substrates were reacted with 5% (v/v) GPTS ethanol solution in a 40 °C oven for a period of 3 h. All the substrates were extensively rinsed with ethanol after carrying out crosslinking reaction in a 110 °C vacuum oven for 3 h.
2.4. Preparation of capture antibody microarrays on the substrates
First, the probe protein dissolved in printing buffer was transferred to a 384-well microplate as the printing source. Then, 250 μg mL−1 of CEA-mAb was used as the capture antibody in printing buffer (0.01 M PBS contains 2.5% glycerol and 0.003% Triton® X-100). Then, the protein microarrays were spotted on the modified plasmonic nanogratings, the Au film, and commercial epoxide modified glass slides using a Smart Arrayer 64 spotter (Capital Bio Ltd., China) equipped with telechem printhead and stealth micrspotting pins. A sample of about 0.5 nL was delivered to each spot on the substrate with a distance of 500 μm between neighboring spots. The spots were delivered on the surface at room temperature and 55% humidity. The exploration of fluorescence enhancement ability of the plasmonic nanograting substrates was performed using 50 μg mL−1 Cy5-IgG in the printing buffer. Then, the as-prepared slides were transferred to the humid chamber and incubated for 8 h at 37 °C. Then, all slides were rinsed thrice for 5 min with 0.05 M TBS to remove the nonspecific adsorption molecules.
2.5. The detection of CEA antigen by microarray
The reacted-slides were incubated with 10 mg mL−1 bovine serum albumin (BSA) (Dingguo Biotechnology Co., Ltd., Beijing, China) solution for 2 h and exhaustively washed in 0.05 M TBS solution to eliminate the unbound molecules. Then, the antibody microarrays were reacted in different concentrations of the CEA antigen sample solutions for another 2 h and rinsed by 0.05 M TBS buffer. The microarrays were sequentially incubated with the recognition antibody CEA-pAb for 60 min and Cy5-labelled secondary antibody for another 30 min. Finally, all the spotted substrates were thoroughly rinsed three times with 0.05 M TBS and DI water. After being dried by centrifugation, the substrates were scanned with the microarray scanner (LuxScan 10K, CapitalBio Ltd., Beijing, China) at an excitation wavelength of 633 nm. The fluorescent images were quantitatively analyzed using a software, LuxScan 3.0. The mean value and the signal standard deviation were determined for the 6 spot replicates of each sample. The fluorescent bleaching is prevented by strictly avoiding light during the experimental process.
2.6. Instruments and characterization
The morphology of the grating nanostructure was obtained using a field emission scanning electron microscope (SEM) (Hitachi SU-8010, Japan) operating at 5 kV. The morphology was also characterized using an atomic force microscope (AFM) (Dimension Icon, Bruker, Germany) under the tapping mode in air. We set the scanning rate at 0.5 Hz. Moreover, the force constant of the commercially available AFM cantilever tips is about 48 N m−1 with the resonance vibration frequency set between 310 and 440 kHz. SPR spectrometry was operated using a TR2005 spectrometer (RES-TEC Corp., Germany). A depolarized He–Ne laser beam (λ = 632.8 nm, diameter = 1 mm) was used for the incident light. The intensity of the laser was controlled by a 10% ND filter. The reflectivity was monitored using a photodiode through a θ–2θ goniometer, through which the light reflected at the substrate was monitored by the photo diode at an incident angle (θ) of 5–40 degrees. X-ray diffraction (XRD) data were determined by a D8 diffraction instrument (Bruker, Germany) with the X-ray source from the Cu-Kα line at 0.15418 nm.
3. Results and discussion
Fig. 1 is a schematic illustration of coating layers of the grating substrate and the antibody microarray assays platform on the different substrates. The substrate consists of the quartz nanograting and a series of plasmonic layers. The layers of Cr/Au/Cr/SiO2 were sputtered on the as-prepared quartz nanograting surface as shown in Fig. 1(a). Instead of silver, used in the previous study, a 150 nm gold film was used to excite the SPR coupling to improve the stability when the coated nanostructure was exposed to the buffer solutions. A layer of SiO2 with the thickness of 40 nm prevents the quenching effect from gold.42,46,47 The sandwich immunoassay was then performed on this nanograting surface using a microarray technique as shown Fig. 1(b). The fluorescence signal on substrates of bare glass, planar Au film, and plasmonic Au nanogratings from protein microarrays was then evaluated by a prevailing read-out technique.12 The gratings were designed with a period of 400 nm and a depth of 30 nm, which was fabricated on quartz substrates by the two light beam laser interference strategy, followed by dry etching procedure as described in our previous literatures.48 The experimental process is shown in Fig. 1(c). The plasmonic nanogratings were chemically functionalized by (3-glycidoxypropyl)trimethoxysilane (GPTS), of which the trimethoxy silane group reacted with SiO2 to form Si–O–Si bond and the epoxy group covalently bound to the amine groups of the protein.12,17 The geometry of the gratings can be varied by changing processing parameters. The morphology of the 1D nanograting was characterized using SEM and AFM (Fig. 2). The data from both SEM and AFM show a period of 400 ± 10 nm and a depth of 30 ± 5 nm of fabricated nanogratings, which are consistent with our design. The roughness of the grating nanostructure increased after the deposition of Cr/Au/Cr/SiO2 layers with the value of root-mean-square (RMS) increasing from 9.8 nm to 14.3 nm from AFM analysis. However, the period and the depth of the groove were contained after the sputtering procedure as shown in Fig. 2(b and d). The XRD pattern of the plasmonic Au nanograting shows a strong narrow peak at 38.4°, corresponding to Au (111) from the Au film coating on the grating (Fig. S1†). There are no distinguishable peaks from Cr because the thickness of Cr layer is ultrathin and the SiO2 layer is of poor crystallinity. The thickness of the Cr and SiO2 layers were analyzed by fitting the prism coupled-SPR curves as described in the ESI (Fig. S2 and S3†).
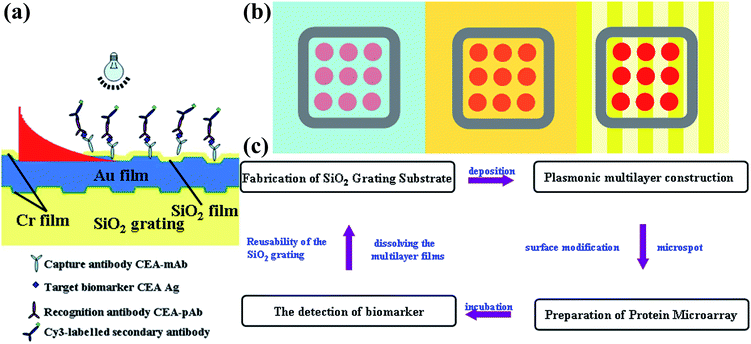 |
| Fig. 1 (a) Scheme of coating layers on the SiO2 grating substrate and schematic illustration of the four-layer antibody microarray assay strategy for the plasmon enhanced fluorescence antibody microarray. (b) Schematic layout of the protein microarrays fabricated on the different substrates of bare glass, planar Au film and planar Au nanograting. (c) The scheme of the whole experimental process. | |
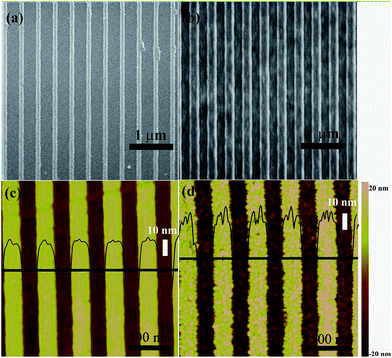 |
| Fig. 2 SEM images of SiO2 gratings (a) before and (b) after coating with multilayer films. AFM images of SiO2 gratings (c) before and (d) after coating with multilayer films. | |
The angle-dependent reflectivity curve was operated to measure the coupling efficiency of SPR. The grating-coupled SPR (GCSPR) is a type of propagated SPR that can provide direct coupling between surface plasmon polaritons and radiation modes; the resonance condition was described using the resonance angle. We constructed the detection environment to the protein microarray analysis on the SPR measurement using a cover glass on the nanograting to form a thin layer of cell for containing the PBS solution as shown in Fig. 3(a). A gap of 0.5 mm between the cover glass and the plasmonic nanograting holds several drops of PBS solution, which is the same as the biological environment for the microarray process. The SPR angle is essential for the microarray read-out system, in which the light is incident and scanned in an angle range of ±5 degrees as illustrated in Fig. 3(a). The angle-dependent reflectivity was then measured to investigate the SPR coupling details for fluorescence enhancement on the as-prepared plasmonic nanograting as shown Fig. 3(b). The SPR angle is at θ = 31 degrees in the air interface and shifts to 5 degrees in the PBS interface, which is favorable for microarrays scanning from the up side of substrates.49 The SPR angle could be calculated based on the dispersion relationship in the following equation.42,48 The angle switch is in accordance with the theoretical results.
where
ω is the angular frequency;
c is the light speed;
ε is the complex dielectric constant of the materials Au and PBS;
θ is the incident angle;
Λ is the grating pitch. The calculated results of grating pitch (
Λ) and the wavelength of the incident light (
λ) varies with the incident angle
θ are shown in Fig. S3.
† The laser of the microarray scanner vertically irradiates the plasmonic nanograting, which captures the incident light and excites the SPR, resulting in the fluorescence enhancement. The grating coupling surface plasmon resonance provides a promising excitation field for enhancing the fluorescence under microarray detection.
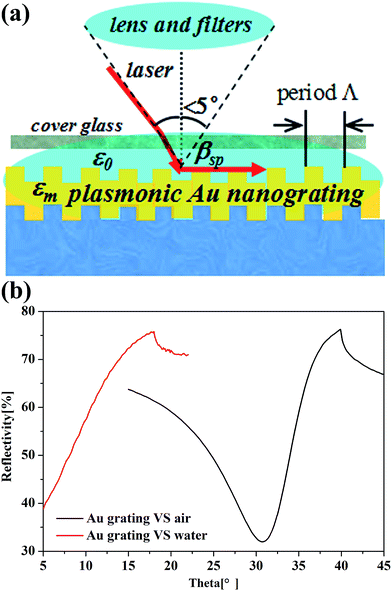 |
| Fig. 3 (a) Scheme of the SPR measurement simulating the environment of protein microarray detection. (b) The experimental angle-dependent reflectivity SPR curves in air and in PBS. | |
The Cy5-IgG microarrays were fabricated on plasmonic Au nanograting, planar Au film and the commercial epoxide modified glass microscope slides to assess the signal amplification ability of the substrates. The fluorescence images and fluorescence intensities are shown in Fig. 4. The fluorescent intensity on the GPTS-modified bare glass reached 1.86 × 103 ± 1.06 × 103 a. u. with quasi rectangular microspots of about 50 μm in diameter. The fluorescent intensity increases slightly (5.68 × 103 ± 1.67 × 103 a. u.) and the dimension of the microspots is around 80 μm on the planar plasmonic Au film. The microspots fabricated on the plasmonic Au nanograting exhibited regular, nearly spherical shapes with the mean diameter of 200 μm as well as a dramatic improvement of the fluorescent intensity of 1.56 × 104 ± 1.73 × 103 a. u., which is a nearly 10-fold amplification compared to that on the glass substrate, thus clearly contributing to the fluorescence enhancement ability of the plasmonic Au nanograting. The microarrays printed on the plasmonic nanograting substrate show homogeneous and circular shapes with excellent reproducibility.
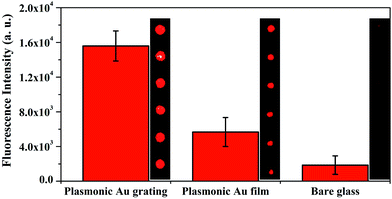 |
| Fig. 4 Fluorescence images and fluorescence intensities observed from nanostructured plasmonic Au grating, planar Au film and glass slide. | |
Furthermore, the advantages of the plasmonic nanograting substrates were evaluated by detecting CEA in 0.01 M PBS utilizing the sandwich formation. CEA detection has been established as crucial for early diagnosis of various human cancers including blood,50,51 breast,52 colorectal,53 lung54 and cervical55 cancers. The calibration curve for diagnosing CEA in PBS on the plasmonic Au nanograting and glass substrate are shown in Fig. 5. Identical CEA sandwich experiments operated on commercial epoxide-functionalized quartz slide are shown for comparison. CEA analysis was operated under identical conditions to that used on glass slide substrates without using plasmonic nanograting substrate for signal amplification. The signal intensity increased with the increase in the CEA concentration. After quantitative analysis, two typical sigmoid dose-dependent curves were plotted with double logarithmic axes. It could be found that the dynamic detection range covers five orders of magnitude from 1 ng mL−1 to 100 μg mL−1 (the whole range gave the favorable fit to y = ax + b). The linear equation of the plasmonic nanograting and the glass substrate is y = 0.0084x + 70.27 (R2 = 0.977) and y = 0.00029x + 43.04 (R2 = 0.986), respectively. The dynamic detection range of glass substrates covers only four orders of magnitude from 10 ng mL−1 to 100 μg mL−1. The LOD of the plasmonic nanograting substrate is 0.36 ng mL−1, which was determined by three standard deviations (SD) above the negative control (NC).12 We showed that the detection limit of our platform is comparable to that of a commercial enzyme linked immunosorbent assay (ELISA) kit. Furthermore, the LOD of our as-fabricated platform is satisfactory for the quantification of diagnosing CEA over the clinically relevant range of 5–100 ng mL−1. The high performance in terms of low LOD with the wide dynamic range contributed to the plasmonic nanograting enabled intrinsic fluorescence amplification. This plasmonic nanograting substrate can be reused without any structural damages by dissolving the multilayer films with aqua regia solution and consequently resputtering the metal layers.
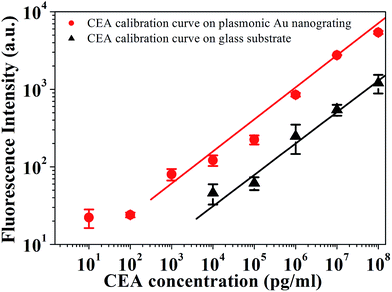 |
| Fig. 5 Calibration curves for CEA detection in 0.01 M PBS on plasmonic Au nanograting and glass substrate. | |
4. Conclusions
The plasmonic nanograting substrate is confirmed to be a superior fluorescence enhancement material for highly sensitive microarray analysis. A low detection limit of 0.36 ng mL−1 with the wide dynamic detection range of 1 ng mL−1 to 100 μg mL−1 was successfully obtained on the plasmonic nanograting substrate for cancer biomarker diagnosis. This developed substrate shows excellent advantages of facile batch manufacturing, adaptability for various fluorophores, reusability, and favorable compatibility with the existent robotic microarrayer. This technique provides great potential for inexpensive, sensitive, and high-throughput cancer biomarker screening in broader clinical and research applications.
Conflicts of interest
The authors declare no competing financial interest.
Acknowledgements
The authors appreciated Prof. Zhenxin Wang (Z. X. Wang) and Dr Tao Li (T. Li) from Changchun Institute of Applied Chemistry (CAS) for the help in the field of protein microarrays preparation. This research was supported by the National Key Research and Development Program of China (2016YFA0200400), Specialized Research Fund for the Doctoral Program of Higher Education (20130061110035), the National Natural Science Foundation of China (No. 21275064 and No. 51571100) and the Fundamental Research Funds for the Central Universities.
References
- D. S. Wilson and S. Nock, Angew. Chem., Int. Ed., 2003, 42, 494–500 CrossRef CAS PubMed.
- M. F. Templin, D. Stoll, M. Schrenk, P. C. Traub, C. F. Vöhringer and T. O. Joos, Trends Biotechnol., 2002, 20, 160–166 CrossRef CAS PubMed.
- Z. Chen, S. M. Tabakman, A. P. Goodwin, M. G. Kattah, D. Daranciang, X. R. Wang, G. Y. Zhang, X. L. Li, Z. Liu, P. J. Utz, K. L. Jiang, S. S. Fan and H. J. Dai, Nat. Biotechnol., 2008, 26, 1285–1292 CrossRef CAS PubMed.
- E. Engvall and P. Perlmann, Immunochemistry, 1971, 8, 871–874 CrossRef CAS PubMed.
- L. H. Tang, Y. Liu, M. M. Ali, D. K. Kang, W. Zhao and A. and J. H. Li, Anal. Chem., 2012, 84, 4711–4717 CrossRef CAS PubMed.
- S. Rauf, L. Zhang, A. Ali, Y. Liu and J. H. Li, ACS Sens., 2017, 2, 227–234 CrossRef CAS PubMed.
- M. J. Wang, L. Y. Wang, G. Wang, X. H. Ji, Y. B. Bai, T. J. Li, S. Y. Gong and J. H. Li, Biosens. Bioelectron., 2004, 19, 575–582 CrossRef CAS PubMed.
- T. Y. Xue, X. Q. Cui, W. M. Guan, Q. Y. Wang, C. Liu, H. T. Wang, K. Qi, D. J. Singh and W. T. Zheng, Biosens. Bioelectron., 2014, 58, 374–379 CrossRef CAS PubMed.
- X. Q. Cui, F. Yang, A. X. Li and X. R. Yang, Anal. Biochem., 2005, 342, 173–175 CrossRef CAS PubMed.
- N. Chartuprayoon, Y. W. Rheem, J. C. K. Ng, J. Nam, W. Chen and N. V. Myung, Anal. Methods, 2013, 5, 3497–3502 RSC.
- B. Zhang, R. B. Kumar, H. J. Dai and B. J. Feldman, Nat. Med., 2014, 20, 948–953 CrossRef CAS PubMed.
- C. Liu, F. L. Meng, W. T. Zheng, T. Y. Xue, Z. Jin, Z. X. Wang and X. Q. Cui, Sens. Actuators, B, 2016, 228, 231–236 CrossRef CAS.
- L. Danckert, S. Hoppe, F. F. Bier and M. von Nickisch-Rosenegk, Microchim. Acta, 2014, 181, 1707–1714 CrossRef CAS PubMed.
- M. Schäferling, M. Riepl, P. Pavlickova, H. Paul, D. Kambhampati and B. Liedberg, Microchim. Acta, 2003, 142, 193–203 CrossRef.
- N. A. Taranova, N. A. Byzova, V. V. Zaiko, T. A. Starovoitova, Y. Y. Vengerov, A. V. Zherdev and B. B. Dzantiev, Microchim. Acta, 2013, 180, 1165–1172 CrossRef CAS.
- T. Li, D. J. Liu and Z. X. Wang, Anal. Chem., 2010, 82, 3067–3072 CrossRef CAS PubMed.
- W. H. Hu, Y. S. Liu, Z. H. Zhu, H. B. Yang and C. M. Li, ACS Appl. Mater. Interfaces, 2010, 2, 1569–1572 CAS.
- B. Schweitzer, S. Roberts, B. Grimwade, W. P. Shao, M. J. Wang, Q. Fu, Q. P. Shu, I. Laroche, Z. M. Zhou, V. T. Tchernev, J. Christiansen, M. Velleca and S. F. Kingsmore, Nat. Biotechnol., 2002, 20, 359–365 CrossRef CAS PubMed.
- J. Yan, C. Y. Hu, P. Wang, R. Liu, X. L. Zuo, X. W. Liu, S. P. Song, C. H. Fan, D. N. He and G. Sun, ACS Appl. Mater. Interfaces, 2014, 6, 20372–20377 CAS.
- Z. X. Wang, R. Levy, D. G. Fernig and M. Brust, J. Am. Chem. Soc., 2006, 128, 2214–2215 CrossRef CAS PubMed.
- A. Wolter, R. Niessner and M. Seidel, Anal. Chem., 2007, 79, 4529–4537 CrossRef CAS PubMed.
- Z. X. Wang, J. Lee, A. R. Cossins and M. Brust, Anal. Chem., 2005, 77, 5770–5774 CrossRef CAS PubMed.
- D. W. Kim, W. L. Daniel and C. A. Mirkin, Anal. Chem., 2009, 81, 9183–9187 CrossRef CAS PubMed.
- H. D. Sikes, R. Jenison and C. N. Bowman, Lab Chip, 2009, 9, 653–656 RSC.
- S. Nagl, M. Schaeferling and O. S. Wolfbeis, Microchim. Acta, 2005, 151, 1–21 CrossRef CAS.
- B. Hötzer, I. L. Medintz and N. Hildebrandt, Small, 2012, 8, 2297–2326 CrossRef PubMed.
- M. Gonzalez-Gonzalez, R. Jara-Acevedo, S. Matarraz, M. Jara-Acevedo, S. Paradinas, J. M. Sayagües, A. Orfao and M. Fuentes, Eur. J. Pharm. Sci., 2012, 45, 499–506 CrossRef CAS PubMed.
- A. A. Ghazani, J. A. Lee, J. Klostranec, Q. Xiang, R. S. Dacosta, B. C. Wilson, M. S. Tsao and W. C. W. Chan, Nano Lett., 2006, 6, 2881–2886 CrossRef CAS PubMed.
- H. Wu, Q. S. Huo, S. Varnum, J. Wang, G. D. Liu, Z. M. Nie, J. Liu and Y. H. Lin, Analyst, 2008, 133, 1550–1555 RSC.
- A. Ressine, S. Ekström, G. Marko-Varga and T. Laurell, Anal. Chem., 2003, 75, 6968–6974 CrossRef CAS PubMed.
- M. K. Dawood, L. Zhou, H. Zheng, H. Cheng, G. Wan, R. Rajagopalan, H. P. Too and W. K. Choi, Lab Chip, 2012, 12, 5016–5024 RSC.
- Y. S. Liu, X. L. Li, S. J. Bao, Z. S. Lu, Q. Li and C. M. Li, Nanotechnology, 2013, 24, 175501 CrossRef PubMed.
- Y. S. Liu, W. H. Hu, Z. S. Lu and C. M. Li, ACS Appl. Mater. Interfaces, 2014, 6, 7728–7734 CAS.
- W. H. Hu, Y. S. Liu, T. Chen, Y. Liu and C. M. Li, Adv. Mater., 2015, 27, 181–185 CrossRef CAS PubMed.
- H. T. Wang, X. Q. Cui, W. M. Guan, X. L. Zheng, H. T. Zhao, Z. Wang, Q. Y. Wang, T. Y. Xue, C. Liu, D. J. Singh and W. T. Zheng, Nanoscale, 2014, 6, 7295–7302 RSC.
- Q. Y. Wang, W. T. Zheng, H. Chen, B. S. Zhang, D. S. Su and X. Q. Cui, J. Power Sources, 2016, 316, 29–36 CrossRef CAS.
- H. Li, W. B. Qiang, C. Z. Wang, M. Vuki and D. K. Xu, Analyst, 2013, 138, 7376–7383 RSC.
- Y. Wang, H. Li and D. K. Xu, Anal. Chim. Acta, 2016, 905, 149–155 CrossRef CAS PubMed.
- H. Li, M. Wang, W. B. Qiang, H. T. Hu, W. Li and D. K. Xu, Analyst, 2014, 139, 1653–1660 RSC.
- L. Malic, B. Cui, T. Veres and M. Tabrizian, Opt. Lett., 2007, 32, 3092–3094 CrossRef CAS PubMed.
- Y. J. Hung, I. I. Smolyaninov, C. C. Davis and H. C. Wu, Opt. Express, 2006, 14, 10825–10830 CrossRef CAS PubMed.
- X. Q. Cui, K. Tawa, H. Hori and J. Nishii, Adv. Funct. Mater., 2010, 20, 546–553 CrossRef CAS.
- Z. Jin, W. M. Guan, C. Liu, T. Y. Xue, Q. Y. Wang, W. T. Zheng and X. Q. Cui, Appl. Surf. Sci., 2016, 377, 207–212 CrossRef CAS.
- Y. S. Liu, C. M. Li, L. Yu and P. Chen, Front. Biosci., 2007, 12, 3768–3773 CrossRef CAS.
- K. Tawa, H. Hori, K. Kintaka, K. Kiyosue, Y. Tatsu and J. Nishii, Opt. Express, 2008, 16, 9781–9790 CrossRef CAS PubMed.
- K. Tawa, X. Q. Cui, K. Kintaka, J. Nishii and K. Morigaki, J. Photochem. Photobiol., A, 2011, 221, 261–267 CrossRef CAS.
- Y. Wang, W. Knoll and J. Dostalek, Anal. Chem., 2012, 84, 8345–8350 CrossRef CAS PubMed.
- X. Q. Cui, K. Tawa, H. Hori and J. Nishii, Appl. Phys. Lett., 2009, 95, 3 Search PubMed.
- L. Ma, Z. J. Zhu, T. Li and Z. X. Wang, Biosens. Bioelectron., 2013, 52, 118–123 CrossRef PubMed.
- M. J. Duffy, Clin. Chem., 2001, 47, 624–630 CAS.
- A. L. Washburn, L. C. Gunn and R. C. Bailey, Anal. Chem., 2009, 81, 9499–9506 CrossRef CAS PubMed.
- G. Sölétormos, D. Nielsen, V. Schiøler, H. Mouridsen and P. Dombernowsky, Eur. J. Cancer, 2004, 40, 481–486 CrossRef PubMed.
- J. Y. Wang, R. P. Tang and J. M. Chiang, Dis. Colon Rectum, 1994, 37, 272–277 CrossRef CAS PubMed.
- H. J. Sung and J. Y. Cho, BMB Rep., 2008, 41, 615–625 CrossRef CAS PubMed.
- G. Borras, R. Molina, J. Xercavins, A. Ballesta and J. R. Iglesias, Gynecol. Oncol., 1995, 57, 205–211 CrossRef CAS PubMed.
Footnote |
† Electronic supplementary information (ESI) available. See DOI: 10.1039/c7ay02232h |
|
This journal is © The Royal Society of Chemistry 2018 |
Click here to see how this site uses Cookies. View our privacy policy here.