DOI:
10.1039/C7AY02631E
(Paper)
Anal. Methods, 2018,
10, 151-157
Synthesis of water-soluble fluorescent carbon dots from Setcreasea purpurea boom and its application for Br2 detection†
Received
11th November 2017
, Accepted 24th November 2017
First published on 27th November 2017
Abstract
Fluorescent carbon dots (CDs) were successfully synthesized via pyrolysis of Setcreasea purpurea boom, providing a simple and convenient method for the preparation of CDs without using toxic or expensive materials. The as-synthesized CDs exhibit excellent water-soluble property, good photostability, and higher fluorescence quantum yield (18%), which makes the as-obtained CDs serve as fluorescent inks for printing luminescent patterns for anti-counterfeiting. In addition, the fabricated CDs have sensitive and selective response to molecular bromine (Br2) and have been successfully used for the detection of Br2 in brine, which is based on the Br2-induced fluorescence quenching of CDs. According to UV-visible and transient state fluorescence spectroscopy, the fluorescence quenching of as-prepared CDs was due to the dynamic collision between the CDs and Br2.
Introduction
For the past few years, carbon dots (CDs) have received much attention for their alluring advantages, which are quite different from traditional heavy-metal-based quantum dots, for example, small dimensions, preferable chemical stability, low toxicity, high aqueous solubility, low photobleaching rate, non-blinking and excellent biocompatibility.1 Therefore, CDs can be widely used in numerous fields, such as sensors,2–4 bioimaging,5,6 patterning,7,8 diagnostics,9 and catalysis.10,11 Till date, numerous methods, including hydrothermal synthesis,12–14 pyrolysis,9,15–17 microwave method18–21 and chemical oxidation treatment22 have been developed for the synthesis of CDs; the CDs prepared by all these methods usually exhibit good dispersion in water. By choosing different carbon precursors and controlling synthetic conditions, the physical and chemical properties of CDs could be changed. For example, Zheng et al.22,23 obtained amphiphilic and hydrophobic CDs using cetylpyridinium chloride (CPC) as a carbon source by controlling the concentration of NaOH and the reaction time. Jiang et al.24 acquired CDs, which emitted red, green and blue colors from three different phenylenediamine isomers (i.e., oPD, mPD and pPD) through a hydrothermal approach. Zheng et al.25 obtained CDs via hydrothermal method using folic acid as a precursor, which emitted green and yellow-green photoluminescence on the addition of H3PO4 and H2C2O4, respectively. All the aforementioned CDs were prepared with chemical reagents as carbon sources, which involved stringent reaction conditions, toxic precursors, unpleasant by-products or post-synthetic steps for surface passivation.26 In recent years, the carbon precursors have been overwhelmingly preferred to be eco-friendly and economical as far as possible.
Biomasses are promising candidates for synthesis of CDs because they are widespread, easily available, low-cost, and green. Numerous bio-resources have been used to prepare CDs, such as cotton,27 plant leaves,8,28 flowers,7,29 garlic,30 and Siraitia grosvenorii.31Setcreasea purpurea boom, comprising large amounts of –OH, –COOH groups in its leaf, could also be used as carbon source for preparing CDs.32–34 The surface of as-fabricated CDs possess some active groups, such as –OH and –COOH after pyrolysis, and can act as a chemical sensor for practical applications.
Bromine is one of the halogen elements, which arises from its widespread usage in industrial materials, including fire retardants, agricultural pesticides and pharmaceuticals.35 Moreover, Br2 is a corrosive and highly toxic chemical that is widely used in research labs and in pharmaceutical industry. The by-products obtained from such laboratories/industries could have low levels of reagents, which contain the final active pharmaceutical ingredients (APIs) as residual impurities.36 Therefore, it is of vital significance to detect trace bromine in samples. At present, there are numerous methods used for Br2 detection, such as absorption spectroscopy,36 inductively coupled plasma mass spectrometry (ICP-MS),37–39 inductively coupled plasma optical emission spectrometry (ICP-OES),37 and ICP-oa-ToF-MS.35 However, these approaches always involve expensive instrumentation, complex operations or tedious sample pretreatment in comparison to the fluorescence method.
In this study, we have developed a one-step strategy for the synthesis of blue fluorescent CDs from Setcreasea purpurea boom under argon atmosphere at 300 °C. The as-prepared CDs possess excellent water dispersibility, strong blue fluorescence emission and a high fluorescence quantum yield of 18%. In addition, the CDs exhibit a selective and sensitive response to Br2, and the fluorescence of the CDs can be quenched due to the dynamic collision between CDs and Br2 after the addition of Br2 in CDs aqueous solution. Hence, the synthesized CDs can be used as fluorescence sensors for the detection of bromine in real samples.
Experimental
Materials
Bromine, MgCl2, CoCl2, BaCl2, NiCl2, CaCl2, PbCl2, CdCl2, AlCl3, FeCl3, AgNO3, Hg(NO3)2, CrCl3, CuCl2, ZnCl2, MnCl2, Na2SO3, NaBr, NaF, Na2MoO4, KSCN, KI, KIO3, K2Cr2O7, NaBrO3, KClO3, Na2S, NaClO, Na2SO4, Na3PO4 and I2 were purchased from Chengdu Kelong Chemical Co., Ltd (China). Phosphate buffer solution (PBS) was prepared by mixing NaH2PO4 and Na2HPO4. Quinine sulphate in 0.1 M H2SO4 was chosen as a standard to measure the quantum yield (QY) of synthesized CDs. The leaves of setcreasea purpurea boom were obtained from the campus of Sichuan University, washed with ultrapure water and then dried in the oven at 35 °C before use. Ultrapure water was obtained from a Millipore water purification system (18.2 MΩ cm at 25 °C) and used in all experiments. All reagents of analytical grade were used without further purification.
Synthesis of CDs
CDs were prepared by a one-step pyrolysis strategy with Setcreasea purpurea boom as the carbon source. Typically, 1.0 g of clean and dried Setcreasea purpurea boom was first cut into fractions, and then placed in a quartz boat in a tube furnace to conduct pyrolysis at 300 °C for 2 h with a gradient heating rate of 5 °C min−1 under argon atmosphere. After cooling down to room temperature, the acquired dark products were mechanically ground. Following this, 0.1 g of the ground powder was dispersed in 30 mL ultrapure water with ultrasound to form a dark brown solution. The granular solid was removed via centrifugation at 10
000 rpm for 10 min. Finally, the pure CDs in aqueous solution were obtained by dialysis with a 3500 Da cutoff membrane. The dialysis was carried out for 10 h to remove the water soluble molecules or ions present in the CDs. The CDs solution was stored at 4 °C and diluted with the ultrapure water before use.
Characterization
Transmission electron microscopy (TEM) was performed on a JEM-2100F field-emission scanning electron microscopy (Japan) with an accelerating voltage of 200 kV. Fourier transform infrared spectroscopy (FTIR) was recorded on a Bruker TJ270-30 spectrometer. Powder X-ray diffraction patterns (XRD) were obtained on a DX-2700 X-ray diffractometer with a Cu Kα source. X-ray photoelectron spectroscopy (XPS) was measured using a Kratos AXIS Ultra DLD X-ray Photoelectron Spectrometer equipped with Mg as the source of excitation (Kratos, UK). Elemental C, H, and N analysis was performed using Elementar vario El cube (Germany). Absorption spectroscopy was conducted on Hitachi U-3010 ultraviolet-visible spectrophotometer (Japan). Fluorescence lifetime measurements were carried out using a Horiba Tempro-01 transient state fluorescence spectrometer (England). All fluorescence measurements were collected on a Hitachi F-4600 fluorescence spectrophotometer (Japan).
Measurement of fluorescence quantum yield
Quinine sulphate dispersed in 0.1 M H2SO4, with quantum yield of 0.54, was adopted as the standard to determine the fluorescence quantum yield of as-synthesized CDs. Using the same excitation wavelength of 340 nm, the fluorescent integral area and relevant absorbance of the dilute solution of the CDs and quinine sulphate were measured. Ultimately, the quantum yield of the CDs was calculated according to the following formula:
where Y is the quantum yield, I is the fluorescent integral area, A refers to the corresponding absorbance, n represents the refractive index of solvent and R is the standard value of quinine sulfate.40,41
Detection of bromine
Typically, 100 μL CDs solution (0.24 mg mL−1) and 0.50 mL PBS buffer solution (pH 6.0) were added to a 10 mL colorimetric cylinder. Then, different concentrations of bromine were added and the final volume of the mixture was adjusted to 5.0 mL with ultrapure water. After incubation for 5 min at room temperature, the fluorescence spectra were recorded under 340 nm excitation.
Analysis of bromine in real samples
To confirm bromine detection function of the as-synthesized CDs in real samples, 20 mL each of brine, dechlorinated dilute brine and primary refined brine were pipetted and filtered through 0.45 μm membrane filter paper. Then, 1.0 mL H2SO4 (0.10 M) and 3.0 mL KBrO3 (5.0 mM) were added into the filtered brine, in sequence, and sample 1 was obtained after mixing in the brown glass volumetric flask. Similarly, 1.5 mL H2SO4 (0.10 M) and 3.0 mL KBrO3 (5.0 mM) were added, in sequence, into dechlorination dilute brine and primary refined brine and mixed several times to obtain sample 2 and 3. A certain volume of the real samples was transferred into a colorimetric cylinder and spiked by adding moderate volumes of bromine standard solution. The resultant solution was analyzed by the above-mentioned method. The calculation of relative standard deviation (RSD) was performed using the formula:
where S is the standard deviation and X is the average of three measurements. The spiked recovery was calculated by the following formula:
where Xmeasured is the marked value and Xtheoretical is the theoretical value.
Results and discussion
Synthesis of CDs
Setcreasea purpurea boom, containing abundant carbohydrates, was used as the carbon source to synthesize carbon dots. For exploring the influence of different preparation methods on the properties of CDs, three methods, i.e., hydrothermal method, pyrolysis in the muffle furnace and pyrolysis in the vacuum tube furnace, were adopted. The fluorescence spectra were measured and the results (Fig. S1†) indicate that the fluorescence intensity of CDs fabricated by the first two methods (hydrothermal method, pyrolysis in the muffle furnace) are relatively weak, which should be attributed to the incomplete carbonization in the hydrothermal process and the oxidation reaction in the muffle furnace. Consequently, the CDs were obtained by pyrolysis in the vacuum tube furnace using Setcreasea purpurea boom as the precursor, which can avoid over-oxidation of CDs during the vacuum pyrolysis process. To obtain excellent CDs, the influence of pyrolysis temperatures and time on fluorescence properties of CDs was also investigated. Fig. S2a† shows the fluorescence spectra of CDs prepared at 200 °C, 250 °C, 300 °C and 350 °C for 2 h in an argon atmosphere. From the results, it can be observed that the sample prepared at 300 °C has the strongest fluorescence emission. In addition, the pyrolysis time at 300 °C was also investigated. The data obtained from Fig. S2b† demonstrates that the optimal pyrolysis time is 2 h. Thus, the subsequent CDs preparation was all carried out in a vacuum tube furnace at 300 °C for 2 h, and blue fluorescent CDs with high quantum yield was successfully synthesized under these optimal conditions as shown in Scheme 1.
 |
| Scheme 1 Schematic illustrations for the synthesis and application of CDs. | |
The characterizations of CDs
Fig. 1 shows the typical transmission electron microscopy (TEM) image of CDs. It can be observed that the as-synthesized CDs are almost spherical in morphology (Fig. 1a). The high-resolution (HR) TEM image reveals that the CDs possess no noticeable lattice fringes (the inset in Fig. 1a), which demonstrates the amorphous nature of the as-prepared CDs. The size distribution of CDs is in the diameter range of 1.7–6.1 nm and with an average diameter of 3.9 nm (Fig. 1b). Fig. 2a shows the XRD pattern of the as-prepared CDs; the broad single peak centered near ca. 21° (2θ) with a relatively low intensity is further accounted for highly disordered carbon with a predominantly amorphous structure of the as-prepared CDs, which is in agreement with the results obtained from TEM.
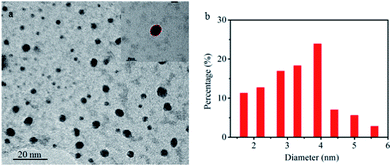 |
| Fig. 1 (a) The TEM image of CDs (inset shows the HRTEM image of one nanoparticle of CDs) and (b) the corresponding particle size distribution histogram. | |
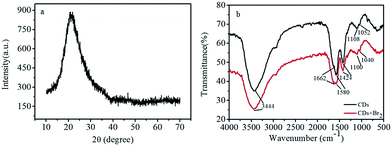 |
| Fig. 2 (a) XRD pattern of CDs. (b) FTIR spectra of CDs and CDs-Br2. | |
FTIR spectra (Fig. 2b) display a broad peak around 3444 cm−1, which can be attributed to the –OH/–NH stretching vibration.27 The peaks around 1662 and 1580 cm−1 arise from the stretching mode of C
O.28 The peak at 1424 cm−1 is ascribed to the stretching vibrations of C–N.42 Furthermore, the peaks at 1100 and 1050 cm−1 can be assigned to the radical group of –C–O.43 This demonstrates that the as-prepared CDs have excellent hydrophilicity and can be well dispersed in water due to the presence of –OH and –COOH groups on their surface. Furthermore, the FTIR spectra of CDs and CDs-Br2 are almost unchanged, further confirming that there was no new substance formed after the addition of Br2 (Fig. 2b). The wide-scan XPS spectrum of CDs (Fig. S3a†) indicate the existence of C, O, and N on the surface of CDs, and the percentage composition for C, O and N is 53.91%, 37.13% and 8.97%, respectively. The CHN analysis results show that the percentage of C, H and N is 34.42%, 4.436% and 3.28%, respectively, further suggesting the presence of numerous active groups on the surface of CDs. The C 1s spectrum (Fig. S3b†) exhibits three peaks at 284.7, 286.3, and 287.8 eV, which belong to C–C/C
C, C–N/C–O, and C
O/C–O–C, respectively.1 The O 1s spectrum (Fig. S3c†) is deconvoluted into two peaks at 531.7 and 533.2 eV, which are attributed to C
O and C–OH/C–O–C, respectively.1 The N 1s spectrum (Fig. S3d†) also displays two peaks at 399.75 and 407.3 eV, which are attributed to C–N and –NO2, respectively.44 The above-mentioned results approximately agree with the results obtained from FTIR measurements, which further manifests that the synthesized CDs possess preferable dispersibility in water and have potential applications in construction of sensors.
The UV-vis absorption spectra of CDs (Fig. 3a) show two weak peaks near 260 and 340 nm, which corresponding to the π–π* and n–π* transition in the CDs, respectively. With a maximum excitation at 340 nm, the CDs exhibit strong photoluminescence in the range of 380–480 nm with a maximum emission wavelength at 415 nm. To further investigate the optical properties of CDs, the fluorescence spectra of CDs under diverse excitation wavelengths (320–500 nm) were carried out (Fig. 3b); the obtained results indicate a red-shift in the emission as the excitation wavelength is changed from 320–500 nm, indicating the tunable emission properties of CDs.45 This excitation-dependence emission behavior of CDs can be attributed to their broad particle size distribution and their different surface states.46,47 In addition, the dilute CDs solution presents strong fluorescence when excited by a 365 nm UV light. The fluorescence quantum yield of the as-fabricated CDs was calculated to be approximately 18% using quinine sulfate as reference. Moreover, the CDs can be used as a fluorescent ink to write on the filter membrane. It can be observed that the written words emit visible blue fluorescence, which can be clearly recognized with naked eyes under 365 nm UV light irradiation (Fig. 4). Hence, the as-prepared CDs could be used for anti-counterfeit technology.
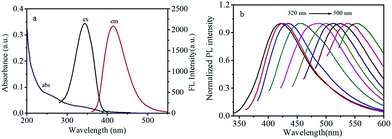 |
| Fig. 3 (a) Absorption, fluorescence excitation spectra and emission spectra of CDs. (b) The corresponding emission spectra by varying the excitation wavelengths from 320 to 500 nm with 20 nm increment. | |
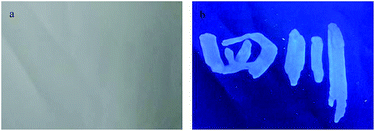 |
| Fig. 4 Symbols written on paper by using CD ink under (a) natural light and (b) UV lamp (365 nm) irradiation. | |
The influence of pH on CDs, their stability and selectivity
The influence of pH from 2 to 12 on fluorescence of the CDs was studied. Fig. S4† shows the fluorescence intensity changes ((I0 − I)/I0) of CDs at different pH values before and after the addition of Br2 in the solution. It can be observed that this change is large and does not change visibly between pH = 2–7. The larger changes of CDs at these pH can be attributed to the strong protonation of CDs because the carboxyl groups could be easily protonated in acidic solutions, which could lead to the aggregation of CDs and result in the fluorescence quenching of CDs.48 When the pH of the solution is higher than 7, the fluorescence clearly decreases due to the weaker protonation of CDs in alkaline conditions. Therefore, in order to obtain a higher and stable sensitivity for Br2 detection, a solution with pH = 6.0 is selected for the subsequent experiments.
The photostability of the as-prepared CDs was investigated. The fluorescence intensity does not change even after continuous excitation at 340 nm for one hour, which demonstrates the preferable photostability of CDs (Fig. S5a†). Moreover, the effects of ionic strength on the stability of CDs were surveyed. As shown in Fig. S5b,† the quenching efficiency of the CDs does not changed significantly by varying the NaCl concentrations, indicating the high stability of the CDs under highly saline conditions.
For the selectivity of as-synthesized CDs, the fluorescence response to various familiar metal cations (including Mg2+, Co2+, Ba2+, Ni2+, Ca2+, Pb2+, Cd2+, Al3+, Fe3+, Ag+ Hg2+, Cr3+, Cu2+, Zn2+ and Mn2+), anions (including SO32−, Br−, F−, MoO42−, SCN−, I−, IO3−, Cr2O72−, BrO3−, ClO3−, S2−, ClO−, SO42−, PO43− and I3−) and Br2 were investigated. The results indicate that all the investigated ions except Br2 have no influence on the fluorescence intensity of CDs (Fig. 5). Therefore, the as-synthesized CDs can be used to construct a fluorescent sensor for the detection of Br2 with excellent selectivity.
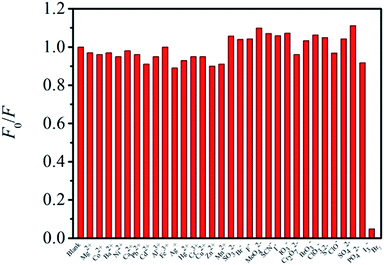 |
| Fig. 5 The response of metal ions, anion and Br2 to CDs. | |
The practical application of CDs as a fluorescence sensor
The selective response of as-fabricated CDs to Br2 indicates that the CDs can be used as a fluorescent sensor to determine bromine in real samples. Therefore, under optimum experimental conditions, the fluorescence intensity changes with different concentrations of Br2 were measured (Fig. 6). The results show that the variation in fluorescence intensity with the concentration of Br2 exhibits a linear relationship in the range of 0.95–13.3 μM (R = 0.9923), and the detection limit for Br2 is calculated to be 4.90 nM based on the formula 3S/k (S denotes the standard deviation and k is the slope of the linear regression equation), which is at least one order of magnitude lower than that measured by microvolume fluorospectrometry,49 microstrip plasma optical emission spectrometry (MSP-OES)50 and high-resolution continuum source molecular absorption spectrometry (HR-CS-MAS).51
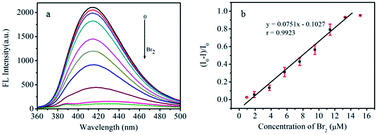 |
| Fig. 6 (a) Fluorescence spectra of CDs in the presence of diverse concentration of Br2. (b) The linear relationship between (I0 − I)/I0 and the concentration of Br2 in the range of 0.95–13.3 μM (Error bars, SD, n = 3). | |
It is well known that brine water generally contains bromide ions, which cannot be directly detected by the constructed CDs-based fluorescent sensor because CDs have no response to Br− according to the above-obtained results (Fig. 5). Therefore, Br− in the samples should be converted into Br2 by the chemical reaction before detection. According to the reaction, 5Br− + BrO3− + 6H+ = 3Br2 + 3H2O, BrO3− reacts with Br− to generate Br2 under acidic conditions, which causes the fluorescence quenching of CDs and the concentration of Br− can be quantitively detected. Therefore, a certain volume of NaBrO3 and hydrochloric acid was added into sample solutions containing Br− before the detection. To validate the feasibility of the sensor based on CDs for the detection of bromine, several real samples including brine water, dechlorinated dilute brine and refined brine water were collected and examined, and the results are shown in Table 1. In addition, the recovery experiments were also carried out by the standard addition method. The experimental results indicate that the CDs as a Br2 sensor could be successfully applied to detect bromine content in high-salinity water samples.
Table 1 Analysis results of bromine in practical samples
Samples |
Content (mg L−1) |
Added (μM) |
Found (μM) |
RSD (%) |
Recovery (%) |
Brine |
91.96 |
2.28 |
2.44 |
2.7 |
107.0 |
Dechlorination dilute brine |
49.65 |
2.47 |
2.38 |
3.4 |
96.4 |
Primary refined brine |
86.89 |
2.09 |
2.19 |
4.9 |
104.8 |
Possible detection mechanism for the response of CDs to Br2
In order to investigate the CDs fluorescence quenching mechanism caused by Br2, the absorption spectra and fluorescence lifetime of CDs aqueous solution before and after the addition of Br2 were measured. It can be observed from Fig. S6a† that the absorption spectra of CDs do not change significantly after the addition of Br2, suggesting that the reaction between CDs and Br2 does not occur. Moreover, the average lifetime of CDs decreases from 6.17 ns to 0.40 ns after adding 19 μM Br2 into the CDs aqueous solution (Fig. S6b†). Therefore, we speculate that this decrease in the lifetime of the CDs should be attributed to the inter-collision between the excited state of the CDs and Br2. The excitation energy of the CDs is transferred to Br2 during this process, resulting in the remarkable fluorescence quenching of CDs, which can be concluded to be dynamic quenching,52 which accounts for the nonradiative electron-transfer from carboxyl groups of CDs to Br2.53–55 It is well known that the electrons of the CDs under the light irradiation can excite electrons from the highest occupied molecular orbital (HOMO) to the lowest unoccupied molecular (LUMO) energy levels. Then, the excited electrons return to the ground state with a radiative transition and emit fluorescence. After the addition of Br2 into the solution of CDs, an inter-collision between the excited state of the CDs and Br2 occurred and the excited electrons of the CDs directly jump to the σ* orbital of Br2 because the ultraviolet absorption of Br2 is at about 270 and 390 nm (inset of Fig. S6a†), which is very close to the LUMO energy levels of CDs.53 Therefore, the electron transfer from CDs to Br2 decreases the irradiative transition and results in the fluorescence quenching of CDs.
Conclusions
In summary, a green, accessible and one-step pyrolytic method was developed to prepare blue fluorescent CDs with high quantum yield (18%). The as-prepared CDs are amorphous, and exhibit water-soluble properties with good photostability. It is interesting to observe that the fluorescence of CDs could be selectively and sensitively quenched by Br2. Therefore, it has been used to construct fluorescent sensor for the detection of Br2, exhibiting higher sensitivity and selectivity with lower limit of detection. The mechanism of fluorescence quenching of CDs by Br2 was also investigated in detail in this study. The constructed fluorescent sensor based on CDs was successfully used for the detection of Br2 in real samples, including brine water, dechlorinated dilute brine, and refined brine water and the desirable results demonstrate its feasible application in high salinity waters.
Conflicts of interest
There are no conflicts to declare.
Acknowledgements
This work was supported by the National Natural Science Foundation of China (21506103), and the Key Research Projects of Sichuan Provincial Department of Education (No. 15ZA0289).
Notes and references
- L. H. Shi, Y. Y. Li, X. F. Li, B. Zhao, X. P. Wen, G. M. Zhang, C. Dong and S. M. Shuang, Biosens. Bioelectron., 2016, 77, 598–602 CrossRef CAS PubMed.
- A. Barati, M. Shamsipur and H. Abdollahi, Biosens. Bioelectron., 2015, 71, 470–475 CrossRef CAS PubMed.
- J. Li, L. P. Lu, T. F. Kang and S. Y. Cheng, Biosens. Bioelectron., 2016, 77, 740–745 CrossRef CAS PubMed.
- T. Q. Han, T. Yan, Y. Y. Li, W. Cao, X. H. Pang, Q. J. Huang and Q. Wei, Carbon, 2015, 91, 144–152 CrossRef CAS.
- P. C. Hsu, Z. Y. Shih, C. H. Lee and H. T. Chang, Green Chem., 2012, 14, 917–920 RSC.
- W. J. Lu, X. J. Gong, M. Nan, Y. Liu, S. M. Shuang and C. Dong, Anal. Chim. Acta, 2015, 898, 116–127 CrossRef CAS PubMed.
- L. H. Shi, Y. Y. Li, X. F. Li, X. P. Wen, G. M. Zhang, J. Yang, C. Dong and S. M. Shuang, Nanoscale, 2015, 7, 7394–7401 RSC.
- L. L. Zhu, Y. J. Yin, C. F. Wang and S. Chen, J. Mater. Chem. C, 2013, 1, 4925–4932 RSC.
- M. Zheng, S. B. Ruan, S. Liu, T. T. Sun, D. Qu, H. F. Zhao, Z. G. Xie, H. L. Gao, X. B. Jing and Z. C. Sun, ACS Nano, 2015, 9, 11455–11461 CrossRef CAS PubMed.
- J. Liu, H. C. Zhang, D. Tang, X. Zhang, L. K. Yan, Y. Z. Han, H. Huang, Y. Liu and Z. H. Kang, ChemCatChem, 2014, 6, 2634–2641 CrossRef CAS.
- Z. Y. Liu, G. X. Zhang, Z. Y. Lu, X. Y. Jin, Z. Chang and X. M. Sun, Nano Res., 2013, 6, 293–301 CrossRef CAS.
- Y. P. Hu, J. Yang, L. Jia and J. S. Yu, Carbon, 2015, 93, 999–1007 CrossRef CAS.
- C. X. Wang, Z. Z. Xu, H. Cheng, H. H. Lin, M. G. Humphrey and C. Zhang, Carbon, 2015, 82, 87–95 CrossRef CAS.
- A. Sachdev and P. Gopinath, Analyst, 2015, 140, 4260–4269 RSC.
- Z. Gao, L. B. Wang, R. X. Su, R. L. Huang, W. Qi and Z. M. He, Biosens. Bioelectron., 2015, 70, 232–238 CrossRef CAS PubMed.
- L. H. Shi, X. F. Li, Y. Y. Li, X. P. Wen, J. F. Li, M. M. F. Choi, C. Dong and S. M. Shuang, Sens. Actuators, B, 2015, 210, 533–541 CrossRef CAS.
- C. E. Machado, L. G. Tartuci, H. F. Gorgulho, L. F. C. de Oliveira, J. Bettini, D. P. dos Santos, J. L. Ferrari and M. A. Schiavon, Chem.–Eur. J., 2016, 22, 4556–4563 CrossRef CAS PubMed.
- X. C. Sun, C. Brückner and Y. Lei, Nanoscale, 2015, 7, 17278–17282 RSC.
- R. M. Shereema, V. Sankar, K. G. Raghu, T. P. Rao and S. S. Shankar, Electrochim. Acta, 2015, 182, 588–595 CrossRef CAS.
- Y. Song, W. Shi, W. Chen, X. H. Li and H. M. Ma, J. Anal. Chem., 2012, 22, 12568–12573 CAS.
- W. W. Guan, W. Gu, L. Ye, C. Y. Guo, S. Su, P. X. Xu and M. Xue, Int. J. Nanomed., 2014, 9, 5071–5078 Search PubMed.
- B. Z. Zheng, T. Liu, M. C. Paau, M. N. Wang, Y. Liu, L. Z. Liu, C. F. Wu, J. Du, D. Xiao and M. M. F. Choi, RSC Adv., 2015, 5, 11667–11675 RSC.
- M. N. Wang, B. Z. Zheng, F. Yang, J. Du, Y. Guo, J. Y. Dai, L. Yan and D. Xiao, Analyst, 2016, 141, 2508–2514 RSC.
- K. Jiang, S. Sun, L. Zhang, Y. Lu, A. G. Wu, C. Z. Cai and H. W. Lin, Angew. Chem., Int. Ed., 2015, 54, 5360–5363 CrossRef PubMed.
- Y. W. Zheng, D. Yang, X. Wu, H. R. Yan, Y. C. Zhao, B. Feng, K. Duan, J. Weng and J. X. Wang, RSC Adv., 2015, 5, 90245–90254 RSC.
- A. Sachdev and P. Gopinath, Analyst, 2015, 140, 4260–4269 RSC.
- X. P. Wen, L. H. Shi, G. M. Wen, Y. Y. Li, C. Dong, J. Yang and S. M. Shuang, Sens. Actuators, B, 2015, 221, 769–776 CrossRef CAS.
- Y. S. Liu, Y. A. Zhao and Y. Y. Zhang, Sens. Actuators, B, 2014, 196, 647–652 CrossRef CAS.
- Y. J. Feng, D. Zhong, H. Miao and X. M. Yang, Talanta, 2015, 140, 128–133 CrossRef CAS PubMed.
- S. J. Zhao, M. H. Lan, X. Y. Zhu, H. T. Xue, T. W. Ng, X. M. Meng, C. S. Lee, P. F. Wang and W. J. Zhang, ACS Appl. Mater. Interfaces, 2015, 7, 17054–17060 CAS.
- Y. B. Su, B. F. Shi, S. Q. Liao, J. J. Zhao, L. N. Chen and S. L. Zhao, ACS Sustainable Chem. Eng., 2016, 4, 1728–1735 CrossRef CAS.
- Y. H. Yuan, R. S. Li, Q. Wang, Z. L. Wu, J. Wang, H. Liu and C. Z. Huang, Nanoscale, 2015, 7, 16841–16847 RSC.
- Z. Wang, Y. X. Lu, H. Yuan, Z. H. Ren, C. Xu and J. Chen, Nanoscale, 2015, 7, 20743–20748 RSC.
- Q. Xu, P. Pu, J. G. Zhao, C. B. Dong, C. Gao, Y. S. Chen, J. R. Chen, Y. Liu and H. J. Zhou, J. Mater. Chem. A, 2015, 3, 542–546 CAS.
- R. E. Sturgeon, Anal. Chem., 2015, 87, 3072–3079 CrossRef CAS PubMed.
- M. Kaur, D. H. Lee, D. S. Yang, H. A. Um, M. J. Cho, J. S. Kang and D. H. Choi, Dyes Pigm., 2015, 123, 317–322 CrossRef CAS.
- R. S. Picoloto, M. Doneda, E. L. M. Flores, M. F. Mesko, E. M. M. Flores and P. A. Mello, Spectrochim. Acta, Part B, 2015, 107, 86–92 CrossRef CAS.
- M. X. Sun, Y. C. Gao, B. W. Wei and X. W. Wu, Talanta, 2010, 81, 473–476 CrossRef CAS PubMed.
- A. L. H. Muller, P. A. Mello, M. F. Mesko, F. A. Duarte, V. L. Dressler, E. I. Muller and E. M. M. Flores, J. Anal. At. Spectrom., 2012, 27, 1889–1894 RSC.
- A. M. Brouwer, Pure Appl. Chem., 2011, 83, 2213–2228 CrossRef CAS.
- B. B. Campos, R. Contreras-Cáceres, T. J. Bandosz, J. Jiménez-Jiménez, E. Rodríguez-Castellón, J. C. G. Esteves da Silva and M. Algarra, Carbon, 2016, 106, 171–178 CrossRef CAS.
- H. Q. Zhang, P. W. Dai, L. Z. Huang, Y. H. Huang, Q. T. Huang, W. X. Zhang, C. Wei and S. R. Hu, Anal. Methods, 2014, 6, 2687–2691 RSC.
- W. J. Shi, H. Fan, S. Y. Ai and L. S. Zhu, New J. Chem., 2015, 39, 7054–7059 RSC.
- A. Ananthanarayanan, Y. Wang, P. Routh, M. Alam Sk, A. Than, M. Lin, J. Zhang, J. Chen, H. D. Sun and P. Chen, Nanoscale, 2015, 7, 8159–8165 RSC.
- W. P. Wang, Y. C. Lu, H. Huang, J. J. Feng, J. R. Chen and A. J. Wang, Analyst, 2014, 139, 1692–1696 RSC.
- S. Zhu, Q. Meng, L. Wang, J. Zhang, Y. Song, H. Jin, K. Zhang, H. Sun, H. Wang and B. Yang, Angew. Chem., Int. Ed., 2013, 52, 3953–3957 CrossRef CAS PubMed.
- Y. Sun, B. Zhou, Y. Lin, W. Wang, K. S. Fernando, P. Pathak, M. J. Meziani, B. A. Harruff, X. Wang and H. Wang, J. Am. Chem. Soc., 2006, 128, 7756–7757 CrossRef CAS PubMed.
- Y. M. Guo, Z. Wang, H. W. Shao and X. Y. Jiang, Carbon, 2013, 52, 583–589 CrossRef CAS.
- A. García-Figueroa, F. Pena-Pereira, I. Lavilla and C. Bendicho, Talanta, 2017, 170, 9–14 CrossRef PubMed.
- P. Pohl, I. J. Zapata, M. A. Amberger, N. H. Bings and J. A. C. Broekaert, Spectrochim. Acta, Part B, 2008, 63, 415–421 CrossRef.
- T. Limburg and J. W. Einax, Microchem. J., 2013, 107, 31–36 CrossRef CAS.
- S. W. Yang, J. Sun, X. B. Li, W. Zhou, Z. Y. Wang, P. He, G. Q. Ding, X. M. Xie, Z. H. Kang and M. H. Jiang, J. Mater. Chem. A, 2014, 2, 8660–8667 CAS.
- S. J. Zhu, Q. N. Meng, L. Wang, J. H. Zhang, Y. B. Song, H. Jin, K. Zhang, H. C. Sun, H. Y. Wang and B. Yang, Angew. Chem., 2013, 125, 4045–4049 CrossRef.
- B. Chen, J. Ma, T. Yang, L. Chen, P. F. Gao and C. Z. Huang, Biosens. Bioelectron., 2017, 98, 36–40 CrossRef CAS PubMed.
- M. F. Jia, Z. Zhang, J. H. Li, H. J. Shao, L. X. Chen and X. b. Yang, Sens. Actuators, B, 2017, 252, 934–943 CrossRef CAS.
Footnote |
† Electronic supplementary information (ESI) available: Fluorescence spectra of CDs. XPS spectra. Effects of pH values, photostability, stability in NaCl aqueous solution. UV-vis absorption spectra and fluorescence lifetime. See DOI: 10.1039/c7ay02631e |
|
This journal is © The Royal Society of Chemistry 2018 |
Click here to see how this site uses Cookies. View our privacy policy here.