Highly electrocatalytic counter electrodes based on carbon black for cobalt(III)/(II)-mediated dye-sensitized solar cells†
Received
11th October 2016
, Accepted 14th November 2016
First published on 14th November 2016
Abstract
While carbon black (CB) is an ordinary, commercial and low-cost carbonaceous material, it displays great potential as an alternative to noble metals and conductive polymers in terms of a counter electrode catalyst for dye-sensitized solar cells (DSSCs) using a Co(bpy)32+/3+ (bpy = 2,2′-bipyridine) redox couple. First, the electrocatalytic activity of this low crystalline material in relation to the cobalt-based redox reaction is carefully studied. The experimental results show that both the heat treatment and the amount of CB loading are key parameters affecting the electrochemical behavior of the resultant CB thin films. A well-prepared CB thin film exhibits better electrocatalysis than sputtered platinum does. This CB film demonstrates its ability to replace common platinum as an efficient counter electrode in DSSCs using a Co(bpy)32+/3+ electrolyte. This material is even more suitable than platinum for fabrication of semitransparent counter electrodes for bifacial photovoltaic applications. Most importantly, a highly efficient Y123-sensitized solar cell is assembled using a CB thin film, revealing a power conversion efficiency of 8.81% without any striking mass transport obstruction. The feasibility of this cost-effective CB material for utilization in DSSCs is definitely verified.
Introduction
At present, worldwide, most electricity is generated from “dirty” energy resources, such as nuclear power, coal, natural gas and crude oil. There is some urgency to acquire a clean, abundant, renewable and environmentally friendly energy source, and solar energy is seen as a prime candidate. The dye-sensitized solar cell (DSSC), which has attracted a great deal of attention since 1991,1,2 is a promising means for the utilization of solar energy. A typical DSSC consists of a photoelectrode for generating photoelectrons, as well as an electrolyte and a counter electrode. The electrolyte normally contains redox couples, which are responsible for the charge shuttling through the electrolyte layer, as well as for the scavenging of photoexcited holes,3 while the counter electrode is employed to collect and transfer electrons from the external circuit to the electrolyte.4,5
Due to certain drawbacks associated with conventional iodide/triiodide redox couples (I−/I3−),6 such as high corrosiveness to many metallic substrates, competitive light absorption hampering photon utilization and low redox potential limiting the photovoltage of related cell devices, the development of alternative redox couples for DSSC applications is inevitable.7–10 In recent years, a type of one-electron outer-sphere redox couple, cobalt complexes,11–18 has emerged as a practical and interesting alternative redox mediator since it does not display the disadvantages of I−/I3−. So far, remarkable photovoltaic conversion efficiencies and high photovoltages have been reported as a judicious optimization of TiO2 thin films, and unique photosensitizers have been implemented.19–23
The selection of material for a counter electrode in DSSCs is dependent on its electrical conductivity and electrocatalytic activity related to the redox reaction of the employed electrolyte. The effective charge transfer properties exhibited in the counter electrode material is one of the vital factors dominating the photovoltaic performance.24,25 Platinum (Pt), the material most widely used as the counter electrode, has been introduced in DSSCs using different kinds of redox couples;7–9,26,27 however, Pt's high cost and scarcity limit its application, especially for the mass production of modular devices. In addition, it has been suggested that the electrocatalytic activity of Pt might gradually diminish when popular I−/I3− redox couples have been executed for a long time, thus causing a reduced photocurrent and inferior stability of the resultant devices.28,29
Developing an alternative Pt-free counter electrode for DSSCs using cobalt(III)/(II)-based electrolytes is definitely a challenge, as it must not only be cost effective but also exhibit evident electrocatalysis in regard to redox reactions. Recently, conductive polymers,30–33 metal compounds,24,34–39 carbonaceous materials24,40–49 and versatile combinations34–37 have emerged as promising candidates. Undoubtedly, carbonaceous materials are catalysts with the lowest cost, and carbon nanomaterials in various forms, such as nanosheets, nanohorns, nanoplatelets, nanotubes and nanoparticles, have been utilized for DSSC applications.24,40–49 Recently, Kavan et al. fabricated cobalt(III)/(II)-mediated DSSCs with graphene-related materials as counter electrode catalysts, and conducted a thorough study of their electrocatalytic characteristics.40,41,43,47
Carbon black is a solid carbon composed of aggregates of carbon particles; it is widely employed as a reinforcing agent in rubber-related products. In addition, carbon black has been introduced in the preparation of counter electrodes in DSSCs using I−/I3− redox couples.50–52 To the best of our knowledge, however, reports on using carbon black as the counter electrode material for cobalt-mediated DSSCs are extremely rare, and the electrochemical behavior of this carbonaceous material related to the cobalt(III)/(II)-based redox reaction has not yet been thoroughly investigated. In this study, the electrocatalytic activity of carbon black is systematically analyzed, and the applicability of this low-cost material for use in DSSCs is evaluated as well. It is demonstrated that commercial carbon black not only reveals outstanding electrocatalysis in relation to the Co(bpy)32+/3+ redox reaction, but is also a promising material to replace the noble Pt and conductive polymers for the fabrication of cost-effective DSSCs.
Results and discussion
Characteristics of carbon black thin films
Carbon black (CB) is of great importance in applications such as coatings and polymers and in the printing industry. In this study, a commercial specialty CB is utilized to replace noble metals and expensive polymers as counter electrode catalysts in DSSCs. The Raman spectrum of the investigated CB powders is shown in Fig. 1(a). On the basis of reports in the relevant literature,53,54 the characteristic peaks at ∼1580 cm−1 and ∼1350 cm−1 are attributed to the G band associated with the ideal graphitic lattice and the D band related to disordered graphite, respectively. The features of the broad bands in this spectrum and the D/G band intensity ratio (ID/IG) of ∼1.26 both substantially suggest a highly disordered graphitic structure with a very low grade of crystallinity. This result was expected because, according to the manufacturer's specifications, these CB powders were produced using a furnace black process, where the partial combustion at a high temperature gives rise to the introduction of defects in the graphite lattice. It also can be seen in Fig. 1(b) that this CB presents a typical aggregate of nanoparticles (∼30 nm in diameter) without a precise crystalline structure. More specific properties of this specialty CB are illustrated in the ESI.†
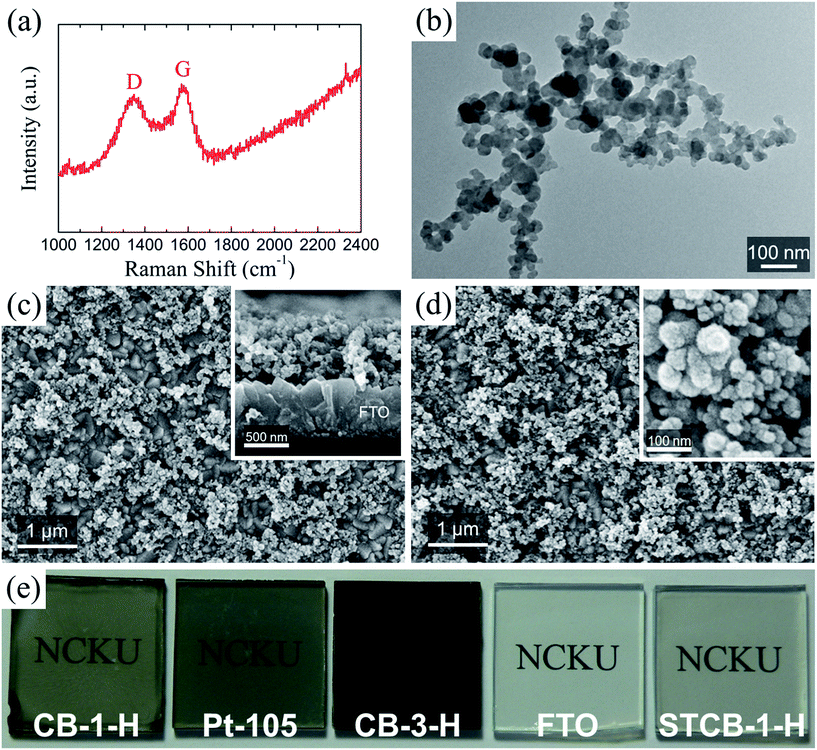 |
| Fig. 1 (a) Raman spectrum at a 532 nm excitation wavelength of carbon black. (b) TEM image of carbon black. Top-view SEM images of the (c) CB-1-H and (d) CB-3-H films with the insets showing cross-sectional and magnified images of the corresponding films, respectively. (e) Digital photographs of FTO substrates coated with the various thin films. | |
All CB thin films in this study were prepared using the spin-coating method. A CB dispersion, consisting of 1.25 wt% CB powders, 0.17 wt% ethyl cellulose and 98.58 wt% ethanol, was dropped onto an FTO conductive glass, and then immediately spun at 2000 rpm for 20 s. Heat treatment was then conducted at 450 °C for 30 min under ambient conditions. The amount of CB deposition was adjusted according to the number of employed coating layers; the CB film prepared by n coating layers with heat treatment is denoted as CB-n-H. The surface morphology of the resultant CB films was investigated using SEM. It can be observed in Fig. 1(c) that the external surface of the FTO substrate coated with a single CB layer (i.e. CB-1-H) is partly covered with aggregated CB clusters, as compared with that of the pristine FTO glass exhibited in Fig. S1 (ESI†). The inset of Fig. 1(c) indicates that the thickness of the resultant CB cluster is around 0.55–0.75 μm, and also that the mesoporous structure of CB catalysts can provide a high electroactive area for the occurrence of redox reactions. As shown in Fig. S2 and S3 (ESI†), furthermore, it is clear that increasing the CB coating layer results in a broader CB cover over the FTO substrates, as well as a larger catalyst thickness, both suggesting an enhanced CB loading. Fig. 1(d) displays the surface morphology of the CB-3-H thin film; the appearance of this film is much darker than that of the CB-1-H film [see Fig. 1(e)], and Fig. S3(c) (ESI†) also illustrates that the cluster thickness increases to around 1.1–1.4 μm. As shown in Fig. S4 (ESI†), however, the surface of the FTO coated with 1 CB layer without heat treatment (denoted as CB-1) is almost covered with CB clusters and ethyl cellulose, and the CB particles are densely surrounded by ethyl cellulose molecules. Therefore, the decomposition of ethyl cellulose during heat treatment can be deduced, which thereby yields a fine particulate morphology, as observed in the inset of Fig. 1(d).
Electrochemistry of the carbon black thin film
The electrochemical characteristics of the prepared CB thin films were carefully studied using cyclic voltammetry (CV), electrochemical impedance spectroscopy (EIS) and Tafel polarization. A platinum thin film prepared by sputter coating for 105 s (denoted as Pt-105) was employed for purposes of comparison. In terms of the DSSC, the reduction of the oxidized redox mediator occurs at the counter electrode, so the electrocatalytic activity of a material utilized for the counter electrode plays a crucial role in the eventual cell performance. Fig. 2 presents the CV curves of the various electrodes obtained at a scan rate of 100 mV s−1 using a three-electrode configuration in conjunction with an electrolyte consisting of 13.2 mM [Co(II)(bpy)3](PF6)2 and 0.1 M TBAPF6 in acetonitrile. A higher cathodic peak current and a smaller peak-to-peak separation potential (ΔEpp) in the CV curve of an electrode, respectively, illustrate a larger electroactive area and an accelerated charge transfer at the electrode/electrolyte interface, both representative of superior electrocatalytic ability.55,56
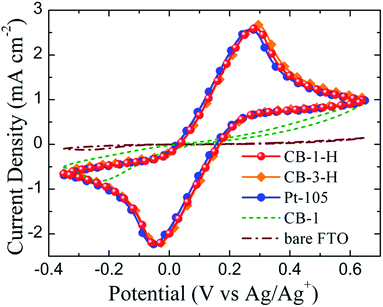 |
| Fig. 2 Cyclic voltammograms of various thin film electrodes measured using a three-electrode system at a scan rate of 100 mV s−1. | |
The pristine FTO and CB-1 electrodes reveal asymmetric curves and relatively small cathodic peaks, indicating the poor electrochemical behavior of both electrodes in the Co(bpy)32+/3+ redox reaction, as well as illustrating the disastrous effect of the presence of ethyl cellulose on the electrocatalysis of CB-based electrodes. In contrast, enhanced peak currents and a pair of distinct peaks are evident in the CV curves of the CB-1-H, CB-3-H and Pt-105 electrodes. The cathodic peak currents of the three electrodes are nearly similar, but the ΔEpp of the CB-3-H electrode is a little larger than that of the CB-1-H or Pt-105 electrode. It is inferred that these three electrodes are able to provide sufficient electrocatalytic sites for the operation of conventional DSSCs.
EIS measurement is widely utilized in the evaluation of interfacial properties and electrochemical characteristics. Symmetrical dummy cells, consisting of two identical CB or Pt electrodes, were assembled for the EIS analyses at a 0 V bias. As exhibited in Fig. 3(a), the Nyquist plots of the studied electrodes show semicircles in the high frequency range, corresponding to the charge transfer at the electrode/electrolyte interface, as well as semicircles in the low frequency range associated with a bulk Nernst diffusion of redox couples. The semicircle related to the Nernst diffusion in the pores is not observed,33,42 possibly due to the incomplete coverage of CB particles over the FTO substrates. The interfacial charge transfer resistance (RCT) can be acquired by fitting the Nyquist spectrum to a well-known equivalent circuit (see Fig. S5 in the ESI†). As summarized in Table 1, the RCT values of the CB-1-H, CB-1 and Pt-105 electrodes are 0.39, 337 and 1.19 Ω cm2, respectively. The RCT value of the Pt-105 electrode is about three times higher than that of the CB-1-H electrode, implying that the CB clusters display a superior electrocatalytic ability towards the Co(bpy)32+/3+ redox reaction, and so are more suitable to be introduced as the counter electrode in DSSCs. Furthermore, a dramatic increase of nearly three orders of magnitude in the RCT value is observed in the CB-1 electrode over that of the CB-1-H electrode, thus reflecting the necessity of heat treatment for CB-coated electrodes, as discussed earlier. Regarding the CB-3-H electrode, the corresponding Nyquist spectrum reveals a semicircle in the high frequency range with very small radii, for which the RCT value is difficult to determine accurately, but better electrocatalytic activity can be fairly anticipated. Besides, a terrible RCT value of ∼1.06 × 106 Ω cm2 for the FTO electrode, obtained by roughly fitting the related Nyquist spectrum (see Fig. S6 in the ESI†), is in line with expectations, as there is no catalyst coated on the FTO surface.
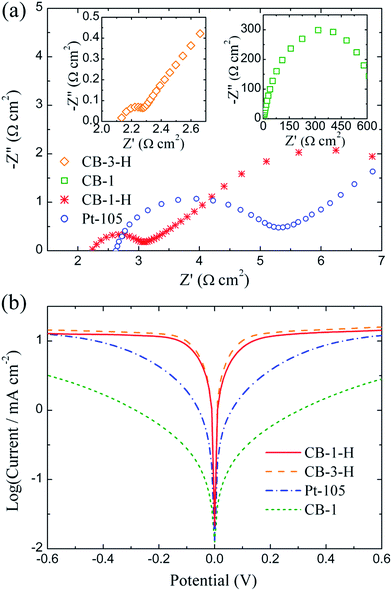 |
| Fig. 3 Electrochemical behavior of Pt-105 and carbon black-derived electrodes: (a) Nyquist plots at 0 V; (b) Tafel polarization curves (10 mV s−1) of the related symmetrical dummy cells. | |
Table 1 Charge transfer resistances and optical transmittances of various counter electrodes together with the corresponding photovoltaic parameters of Co(bpy)32+/3+-mediated DSSCs using Z907 dye sensitizers
Counter electrode |
R
CT
(Ω cm2) |
T
550 nm
(%) |
J
sc (mA cm−2) |
V
oc (mV) |
FF |
η (%) |
Charge transfer resistance derived from impedance measurements.
Optical transmittance at a wavelength of 550 nm with respect to air as a basis.
|
CB-1-H |
0.39 |
49.4 |
11.81 |
852 |
0.71 |
7.14 |
CB-1 |
337 |
N/A |
11.40 |
825 |
0.38 |
3.57 |
CB-3-H |
<0.39 |
22.8 |
11.74 |
853 |
0.72 |
7.21 |
Pt-105 |
1.19 |
38.3 |
11.82 |
846 |
0.71 |
7.10 |
FTO |
∼1.06 × 106 |
77.7 |
1.46 |
765 |
0.07 |
0.08 |
Tafel polarization was carried out at a scan rate of 10 mV s−1 using symmetrical dummy cells identical to those prepared for the EIS measurement. Theoretically, the limiting current density (Jlim), with respect to the diffusion of the redox shuttles [i.e. Co(bpy)32+/3+], and the exchange current density (J0) related to the intrinsic electron transfer at equilibrium at the electrode/electrolyte interface, can be estimated in the diffusion zone and the Tafel zone of a polarization curve. As is clearly evident in Fig. 3(b), the trend of approaching the plateaus is much more rapid for the CB-1-H electrode as compared to the Pt-105 electrode, indicating that a larger J0 is to be expected for the CB-1-H electrode according to the published literature.40,41,43,47 This feature not only is in accordance with the tendency observed in the EIS results, but also indicates the extraordinary electrocatalytic activities of CB electrodes. Moreover, the diffusion coefficient of Co(bpy)33+ (D) of the CB-1-H electrode was calculated to be 8.7 × 10−6 cm2 s−1 using the equation of 2ncFD = Jlimδ (n, c, F and δ are the number of electrons, concentration, Faraday constant and Surlyn thickness, respectively); this value was roughly comparable to that published in Kavan's work.41
The electrochemical stability of CB-1-H and Pt-105 electrodes was also examined by repeated CV measurements at a scan rate of 50 mV s−1 using the same dummy cells. As shown in Fig. S7 (ESI†), the Pt-105 electrode clearly reveals variant CV curves at different scanning cycles, whereas the limiting current density of the CB-1-H electrode is gradually enhanced during the first 50 cycles. The CV characteristics of the CB-1-H electrode are still unknown, requiring further electrochemical analysis. The curves of the CB-1-H electrode, however, do not change their shape during the scanning period; they appear to approach a steady state beyond the fiftieth cycle illustrating that the CB film is considerably more stable than platinum in relation to the Co(bpy)32+/3+ redox reaction. Consequently, based on the electrochemical results, as discussed above, the distinctive effect of heat treatment on CB-coated electrodes was illustrated, and the superior electrocatalytic activity of commercial CB powders over sputtered platinum was clearly demonstrated as well.
Counter electrode prepared using carbon black in Z907 DSSC
The Pt-105 and CB thin films mentioned above were introduced as counter electrodes in the assembly of Co(bpy)32+/3+-mediated DSSCs using Z907 ruthenium dye sensitizers. The J–V curves of the corresponding cell devices measured under simulated AM 1.5G (100 mW cm−2) irradiation are depicted in Fig. 4(a), and the related photovoltaic characteristics are summarized in Table 1. It is not surprising that the cell device using bare FTO without any catalyst coating shows no typical photovoltaic characteristics. The DSSC equipped with a CB-1-H electrode presents a short-circuit photocurrent density (Jsc) of 11.81 mA cm−2, an open-circuit photovoltage (Voc) of 852 mV, a fill factor (FF) of 0.71 and a conversion efficiency (η) of 7.14%. These parameters are eminently consistent with those of a cell device employing the CB-3-H electrode. This indicates that the CB-1-H film possessing a mesoporous structure is able to offer sufficient electrocatalytic sites for the representative operation of a Co(bpy)32+/3+-mediated DSSC, and the films containing greater amounts of catalyst (i.e. CB-m-H, m > 1) are not beneficial for the improvement in photovoltaic performance (more results are summarized in Table S1 in the ESI†). In addition, the solar cell based on an ethyl cellulose-rich electrode (CB-1) reveals an anomalous J–V characteristic, reflecting marked decreases in both FF (0.38) and efficiency (3.57%). This feature can be reasonably attributed to the weak electrocatalysis related to the reduction of Co(bpy)33+ complex ions, as discussed previously.
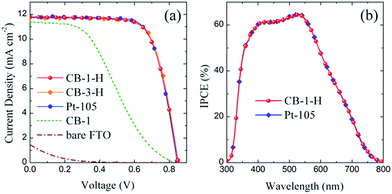 |
| Fig. 4 (a) Photovoltaic J–V curves of Z907 DSSCs using various counter electrodes measured under standard 1 sun irradiation. (b) IPCE spectra of Z907 DSSCs employing CB-1-H and Pt-105 counter electrodes. | |
Furthermore, it is noteworthy that there is no significant discrepancy in the photovoltaic performance between the DSSCs using CB-1-H and Pt-105 as the counter electrodes. Also, the incident photon-to-current efficiency (IPCE) spectra of these two cell devices are virtually identical, as exhibited in Fig. 4(b). On the basis of these results, regarding the efficient counter electrode material utilized in cobalt(III)/(II)-mediated DSSCs, it is clear that commercial CB powders rival conventional platinum. The stability of a DSSC employing the CB-1-H electrode measured over 36 days under ambient conditions is illustrated in Fig. S8 (ESI†). Although there are some minor fluctuations, the cell device can maintain almost 100% of its initial photovoltaic behavior within the test period. In short, a well-known carbonaceous material, carbon black, has been demonstrated to possess an impressive ability as an efficient counter electrode catalyst for Co(bpy)32+/3+-mediated DSSC applications.
Semitransparent carbon black film for bifacial DSSC applications
Commercial CB powders are further demonstrated to be an appropriate candidate for bifacial photovoltaic applications.55,57–59 In order to fulfill the demand for high optical transmittance, the recipe for CB dispersion was adapted to 0.19 wt% CB powders, 0.15 wt% ethyl cellulose and 99.66 wt% ethanol. A transparent CB film was prepared by spin-coating the dispersion once on the FTO substrate followed by a heat treatment; as exhibited in Fig. 1(e), the resultant film (denoted as STCB-1-H) evidences a semitransparent feature, slightly more opaque than the pristine FTO, but more transparent than the CB-1-H film. The UV-vis spectra, as shown in Fig. 5(a), represent the optical transmittances of various thin film electrodes. The CB-1-H and Pt-105 films have transmittances of 49.4% and 38.3%, respectively, at a wavelength of 550 nm with respect to air as a basis (T550 nm). Both are consistent with their observable degree of transparency. Furthermore, the transmittance spectrum of the STCB-1-H film (T550 nm = 73.4%) is located just slightly below that of bare FTO, and it is nearly identical to that of the Pt film prepared by sputter coating for 15 s (denoted as Pt-15 with T550 nm = 72.3%), implying its applicability in the fabrication of bifacial DSSCs.
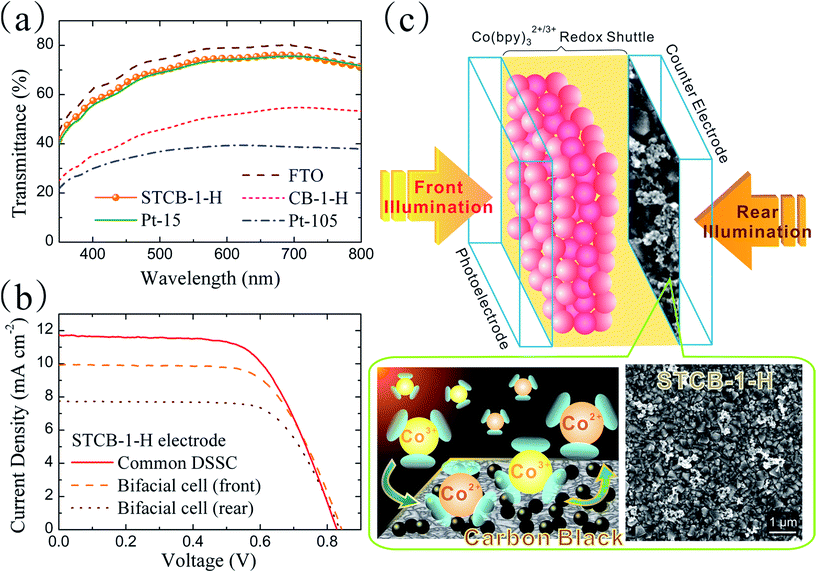 |
| Fig. 5 (a) Optical transmittances of various electrodes with respect to air as the basis. (b) Photovoltaic J–V curves of Z907 DSSCs assembled with STCB-1-H cathodes; a double-layered TiO2 film is utilized in the common DSSC, and the bifacial cell, composed of a single ∼4.0 μm transparent TiO2 film, is measured from the front and rear illumination. (c) Schematic illustration of a bifacial DSSC based on a semitransparent counter electrode in conjunction with Co(bpy)32+/3+ redox shuttles. | |
The J–V curves and photovoltaic parameters of Z907 cell devices using STCB-1-H as the counter electrode are shown in Fig. 5(b) and Table 2, respectively. A common DSSC is assembled using a conventional photoelectrode consisting of a double-layered TiO2 architecture (i.e. transparent layer and light-scattering layer). Compared to the DSSC equipped with a CB-1-H counter electrode, inferior performance results are exhibited for the solar cell employing the STCB-1-H electrode (η of 6.12%). The dramatic decrease in FF (from 0.71 to 0.63) can be ascribed to the shortage of electrocatalytic sites originating from the extremely low amount of CB catalyst loaded onto the FTO substrate, as exhibited in Fig. 5(c). The inferior electrocatalysis of the STCB-1-H film is also verified by the higher RCT value of 9.83 Ω cm2 (see Fig. S9 in the ESI†). This problem is often encountered as the transparent characteristic of an electrode is required. With regard to the common DSSC adopting a Pt-15 electrode, it even reveals much lower conversion efficiency (3.36%). It thereby illustrates that even though there is merely a small amount of catalyst, the deposited CB clusters revealing a mesoporous structure can present considerable electroactive area leading to such electrocatalytic activity. More coating layers are undoubtedly advantageous for the electrocatalysis, but will cause the sacrifice of optical transparency. As a consequence, the semitransparent CB thin film (i.e. STCB-1-H) appears to be a suitable candidate for a counter electrode in bifacial Co(bpy)32+/3+-mediated DSSCs.
Table 2 Photovoltaic characteristics of Z907 DSSCs using Co(bpy)32+/3+ redox couples and STCB-1-H counter electrodes (T550 nm = 73.4%)
Devicea |
J
sc (mA cm−2) |
V
oc (mV) |
FF |
η (%) |
A double-layered TiO2 architecture is introduced in the common DSSC, and the bifacial cell is composed of a single transparent TiO2 film.
Irradiation from the photoelectrode.
Irradiation from the counter electrode.
The device using Pt-15 as a counter electrode.
|
Common DSSCb |
11.72 |
829 |
0.63 |
6.12 |
Common DSSC (Pt-15)b,d |
11.18 |
834 |
0.36 |
3.36 |
Bifacial cell (front)b |
9.92 |
843 |
0.65 |
5.44 |
Bifacial cell (rear)c |
7.74 |
838 |
0.68 |
4.41 |
To attain the feature of irradiation from dual sides, as illustrated in Fig. 5(c), the photoelectrode in a bifacial DSSC is composed of only a single transparent TiO2 layer with a thickness of ∼4.0 μm. The J–V curves and related parameters of the bifacial Z907 DSSC, using STCB-1-H as a counter electrode, are shown in Fig. 5(b) and Table 2, respectively. It is evident that the striking discrepancy in Jsc is the primary cause for the different cell performance when the direction of illumination is changed. This result is due to the longer distance for photons travelling to the dye-sensitized layers as irradiated from the rear side, where the photon might be intercepted by counter electrode materials or redox couples. In addition, the specific value of conversion efficiencies measured from the rear (4.41%) to the front (5.44%) illumination exceeds 0.8, which is highly comparable to those reported in other studies.57,59 Consequently, the feasibility of the semitransparent thin film prepared using commercial CB for bifacial DSSC applications is successfully demonstrated.
Carbon black counter electrode in highly efficient Y123 DSSC
A well-known organic molecule, Y123 dye, has been employed as the photoactive sensitizer for highly efficient DSSCs.16,19,30,40,41,43,44 In this work, in order to boost the power conversion efficiency and simultaneously examine the applicability of CB counter electrodes for highly efficient DSSCs, a Y123 dye sensitizer was introduced. The transparent layer fabricated by larger TiO2 particles (∼30 nm in diameter) to a thickness of ∼7.5 μm was employed to relieve the diffusion limitation of the Co(bpy)33+ complexes. The J–V curve of the Co(bpy)32+/3+-mediated Y123 DSSC assembled using a CB-1-H counter electrode is depicted in Fig. 6(a). The photovoltaic characteristics of this device, measured under 1 sun irradiation (100 mW cm−2), are a Jsc of 13.44 mA cm−2, a Voc of 886 mV, a FF of 0.74 and an η of 8.81%, as summarized in Table 3. According to the related IPCE spectrum, as exhibited in the inset of Fig. 6(a), the wavelength range, with respect to the photon utilization for the Y123 dye, is not as broad as that for the Z907 dye, but higher IPCE values over a wide range from 350 nm to 650 nm are revealed in the Y123 cell device. Therefore, a drastically improved photovoltaic performance can be deduced from the superior light harvesting ability of the Y123 dye, as well as a possibly better charge collection efficiency. Of particular importance is that the highly efficient Co(bpy)32+/3+-mediated DSSC has been well fabricated using commercial CB as a counter electrode catalyst.
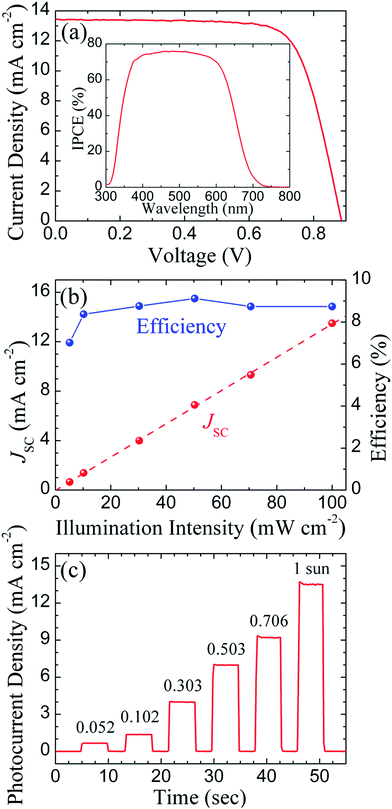 |
| Fig. 6 Photovoltaic behavior of the Y123 DSSC employing a CB-1-H counter electrode in conjunction with the Co(bpy)32+/3+ redox couple: (a) J–V curve measured under standard 1 sun irradiation and an inset depicting the IPCE spectrum; (b) Jsc and power conversion efficiency plotted as a function of the illumination intensity; (c) photocurrent transient dynamics detected under different light intensities (in sun). | |
Table 3 Photovoltaic characteristics of a Co(bpy)32+/3+-mediated Y123 DSSC equipped with a CB-1-H counter electrode measured under different irradiation intensities
Light intensity (Sun) |
J
sc (mA cm−2) |
V
oc (mV) |
FF |
η (%) |
1 |
13.44 |
886 |
0.74 |
8.81 |
0.706 |
9.32 |
883 |
0.75 |
8.74 |
0.503 |
6.88 |
877 |
0.76 |
9.12 |
0.303 |
3.99 |
864 |
0.77 |
8.76 |
0.102 |
1.38 |
834 |
0.74 |
8.37 |
0.052 |
0.65 |
806 |
0.69 |
7.02 |
Apart from the standard 1 sun, this Y123 cell device was further investigated under various light illuminations. The resultant J–V curves and related parameters are exhibited in Fig. S10 (ESI†) and Table 3, respectively. The dependence of Jsc and η on the illumination intensity is illustrated in Fig. 6(b). In terms of power conversion efficiency, the Y123 device performed well and displayed an efficiency of 7.02% at an intensity of 5.2 mW cm−2, implying its possible utilization under indoor or dim light conditions. In addition, it is obvious that the Jsc displays a near linear response to increasing light intensity, which suggests not only an efficient charge extraction, but also the possible absence of any mass transport limitation.13,19,30,41
The diffusion limitations that arise from the slow mass transport of Co(bpy)33+ complexes through mesoporous TiO2 film to the counter electrode have been known to interfere with photovoltaic behavior.13,30,60 The photocurrent transient dynamics of the Y123 solar cell at different illumination intensities is shown in Fig. 6(c). Small photocurrent spikes can be observed when lights with the intensities of 70.6 and 100 mW cm−2 were switched on; the peak currents at each spike are only slightly higher than their steady-state currents, denoting that the diffusion limitation is too trivial to affect the cell performance. In contrast, the photocurrent dynamics behaves as a steady state without any spikes at lower illumination intensities. To summarize, these results readily explain the linear behavior of the photocurrents mentioned above, and further demonstrate that the DSSC assembled using commercial CB powders as a counter electrode material can be utilized in a wide light-intensity range.
Conclusions
In conclusion, a commercial carbonaceous material, carbon black, was utilized herein to fabricate the counter electrodes in dye-sensitized photovoltaic devices employing Co(bpy)32+/3+ as the redox couple. This CB has a disordered graphitic structure with an extremely low grade of crystallinity. The electrocatalysis of the resultant CB thin films in regard to the Co(bpy)32+/3+ redox reaction has been thoroughly investigated. The experimental results reveal that the electrochemical behavior of CB-coated FTO electrodes is dominated by the heat treatment, as well as the amount of CB deposition. A well-prepared CB thin film presents an electrocatalytic activity which is markedly superior to that of a conventional sputtered Pt. A Z907-sensitized solar cell fabricated using this CB thin film as the counter electrode shows a highly comparable photovoltaic performance to that of the device equipped with a Pt counter electrode. This commercial CB material is further introduced to other advanced photovoltaic applications. Based on the similar transparencies, a semitransparent CB thin film is much better than the Pt one as the counter electrode catalyst for bifacial DSSCs. A highly efficient Y123-sensitized solar cell is also assembled using a CB counter electrode. It exhibits an exceptional efficiency of 8.81% without any significant mass transport limitations. This study demonstrates that a commercial CB powder is not only an efficient electrocatalyst in relation to the cobalt complex redox reaction, but also a remarkable candidate to replace the expensive Pt and conductive polymers for the low-cost counter electrode in DSSCs.
Experimental section
Preparation of carbon black and platinum thin film electrodes
Fluorine-doped tin oxide-coated glass (FTO, TEC 7, 2.2 mm thick, 7 Ω sq−1) was used as the substrate to fabricate the carbon black (CB) and platinum (Pt) electrodes in this study. The blank FTO substrate was first drilled with two holes on dual sides, as necessary when employed as a counter electrode in a cell device. Prior to coating CB or Pt films, the FTO substrates were ultrasonicated with a cleaner, deionized water and ethanol for 15 min, successively. The CB films were deposited on FTO using the spin coating method. In brief, the CB dispersion, containing CB powders, ethyl cellulose and ethanol, was dropped on the as-prepared FTO substrates, and then spun at 2000 rpm for 20 s. Uniform CB films were obtained after drying at room temperature, and a geometric area about 5 × 5 mm2 was further defined by erasing other parts of the CB deposition. A heat treatment under ambient conditions was finally conducted on area-defined CB films at 450 °C for 30 min. In addition, Pt thin films were deposited on the as-prepared FTO substrates by utilizing an automatic sputter coater (108auto, Cressington Scientific Instruments, England). The sputter coating process was carried out in an argon atmosphere at a pressure of ∼0.06 mbar with a controlled current of 40 mA. In this study, the amount of Pt deposition over the FTO was adjusted by the coating time only; the Pt electrodes prepared by sputter coating for 105 and 15 s were denoted as Pt-105 and Pt-15, respectively.
Fabrication of the solar cell device
A complete sandwich-type solar cell device was fabricated by assembling a dye-sensitized photoelectrode and a CB-coated (or Pt-coated) counter electrode with a sealing Surlyn (25 μm-thick, Meltonix 1170-25, Solaronix) as a spacer. The cobalt-based electrolyte was injected into the assembled cell device through predrilled holes on the counter electrode; the device was then sealed using 25 μm-thick Surlyn and a square of slide glass. The electrolyte composition for the Z907 device was 0.132 M [Co(II)(bpy)3](PF6)2, 0.02 M [Co(III)(bpy)3](PF6)3, 0.2 M tBP and 0.1 M LiClO4 in acetonitrile; for the Y123 device, it was 0.22 M [Co(II)(bpy)3](PF6)2, 0.05 M [Co(III)(bpy)3](PF6)3, 0.2 M tBP and 0.1 M LiClO4 in acetonitrile. The redox mediators, [Co(II)(bpy)3](PF6)2 and [Co(III)(bpy)3](PF6)3, were synthesized according to the reported literature.11,13 The active area of each cell device for illumination was defined as 0.16 cm2, and at least five cell devices under each condition were assembled in order for the reproducibility of the photovoltaic performance to be examined.
The symmetrical dummy cells for measuring impedance spectra, Tafel polarizations and the electrochemical stability were fabricated by using two electrodes which were both coated with the identical CB or Pt films, but only one with holes drilled on dual sides. The two electrodes were sealed and separated by 25 μm-thick Surlyn, leaving an active area of 0.5 × 0.5 cm2. The same electrolyte as that utilized in the Z907 device was further injected into the sandwich dummy cell through the holes, and finally the holes were sealed using the same procedure mentioned above.
Characterization and measurements
The Raman spectrum of the CB powder was accumulated using a confocal DXR Raman microscopy system (Thermo Scientific, USA) with a 532 nm laser at 7 mW. A transmission electron microscope (TEM, H-7500, Hitachi, Japan) was utilized to analyze the CB nanoparticles. The top-view and cross-sectional images of the CB-loaded FTO electrodes were taken using a scanning electron microscope (SEM, SU8010, Hitachi, Japan). A UV-vis spectrometer (Cintra 10e, GBC Scientific Equipment, Australia), operating in transmission mode, was used to record the optical spectra of the CB and Pt electrodes. Cyclic voltammetry was performed on a CH Instruments potentiostat (CHI627D, USA) using a three-electrode system at a scan rate of 100 mV s−1. A platinum foil and an Ag/Ag+ reference electrode were employed as the counter and reference electrodes, respectively; the electrolyte solution was 13.2 mM [Co(II)(bpy)3](PF6)2 and 0.1 M TBAPF6 in acetonitrile. The photovoltaic characteristics (J–V curves) of the solar cell devices were measured using a digital sourcemeter (6240A, computer-controlled, ADCMT, Japan), scanning from 0 V to an open-circuit voltage at a scan rate of 50 mV s−1 under simulated sunlight irradiation; the solar simulator (XES-301S, AM 1.5G, AAA class, San-Ei Electric, Japan) was calibrated by using a certified reference silicon cell (BK7, PV Measurements). The incident photon-to-current efficiency spectra of the solar cell devices were detected using a xenon lamp-equipped quantum efficiency measurement system (QE-R3011, Enlitech, Taiwan) operating in DC mode. A potentiostat (PGSTAT30, Autolab) equipped with an FRA module was employed to execute the electrochemical impedance spectroscopy (EIS) and Tafel polarizations. The EIS measurements were carried out at 0 V over a frequency range of 100 kHz to 100 mHz with applied 10 mV AC sinusoidal signals; the Tafel polarization analyses were conducted at a scan rate of 10 mV s−1.
Acknowledgements
This work is supported by the Ministry of Science and Technology (MOST) of Taiwan (MOST 103-2221-E-006-248-MY3, 104-2119-M-006-003 and 105-2623-E-006-011-ET). Partial support of this work by the Ministry of Education of Taiwan under the Headquarters of University Advancement at the National Cheng Kung University is also acknowledged. The authors thank Prof. Peter Chen for fruitful discussion and Wei-Hsun Lin for helpful experimental assistance. I-Ping Liu is grateful to Hsing Tian Kong in Taiwan for the long-term scholarship.
References
- B. O'Regan and M. Grätzel, Nature, 1991, 353, 737–740 CrossRef.
- S. Zhang, X. Yang, Y. Numata and L. Han, Energy Environ. Sci., 2013, 6, 1443–1464 CAS.
- J. Wu, Z. Lan, J. Lin, M. Huang, Y. Huang, L. Fan and G. Luo, Chem. Rev., 2015, 115, 2136–2173 CrossRef CAS PubMed.
- T. N. Murakami and M. Grätzel, Inorg. Chim. Acta, 2008, 361, 572–580 CrossRef CAS.
- M. Wu and T. Ma, J. Phys. Chem. C, 2014, 118, 16727–16742 CAS.
- G. Boschloo and A. Hagfeldt, Acc. Chem. Res., 2009, 42, 1819–1826 CrossRef CAS PubMed.
- H. Nusbaumer, J.-E. Moser, S. M. Zakeeruddin, M. K. Nazeeruddin and M. Grätzel, J. Phys. Chem. B, 2001, 105, 10461–10464 CrossRef CAS.
- M. Wang, N. Chamberland, L. Breau, J.-E. Moser, R. Humphry-Baker, B. Marsan, S. M. Zakeeruddin and M. Grätzel, Nat. Chem., 2010, 2, 385–389 CrossRef CAS PubMed.
- T. Daeneke, T.-H. Kwon, A. B. Holmes, N. W. Duffy, U. Bach and L. Spiccia, Nat. Chem., 2011, 3, 211–215 CrossRef CAS PubMed.
- F. Bella, C. Gerbaldi, C. Barolob and M. Grätzel, Chem. Soc. Rev., 2015, 44, 3431–3473 RSC.
- S. A. Sapp, C. M. Elliott, C. Contado, S. Caramori and C. A. Bignozzi, J. Am. Chem. Soc., 2002, 124, 11215–11222 CrossRef CAS PubMed.
- H. Nusbaumer, S. M. Zakeeruddin, J.-E. Moser and M. Grätzel, Chem.–Eur. J., 2003, 9, 3756–3763 CrossRef CAS PubMed.
- S. M. Feldt, E. A. Gibson, E. Gabrielsson, L. Sun, G. Boschloo and A. Hagfeldt, J. Am. Chem. Soc., 2010, 132, 16714–16724 CrossRef CAS PubMed.
- D. Zhou, Q. Yu, N. Cai, Y. Bai, Y. Wang and P. Wang, Energy Environ. Sci., 2011, 4, 2030–2034 CAS.
- J. Liu, J. Zhang, M. Xu, D. Zhou, X. Jing and P. Wang, Energy Environ. Sci., 2011, 4, 3021–3029 CAS.
- J.-H. Yum, E. Baranoff, F. Kessler, T. Moehl, S. Ahmad, T. Bessho, A. Marchioro, E. Ghadiri, J.-E. Moser, C. Yi, Md. K. Nazeeruddin and M. Grätzel, Nat. Commun., 2012, 3, 631 CrossRef PubMed.
- F. Bella, N. Vlachopoulos, K. Nonomura, S. M. Zakeeruddin, M. Grätzel, C. Gerbaldi and A. Hagfeldt, Chem. Commun., 2015, 51, 16308–16311 RSC.
- H. Ellis, R. Jiang, S. Ye, A. Hagfeldt and G. Boschloo, Phys. Chem. Chem. Phys., 2016, 18, 8419–8427 RSC.
- A. Yella, H.-W. Lee, H. N. Tsao, C. Yi, A. K. Chandiran, Md. K. Nazeeruddin, E. W.-G. Diau, C.-Y. Yeh, S. M. Zakeeruddin and M. Grätzel, Science, 2011, 334, 629–634 CrossRef CAS PubMed.
- S. Mathew, A. Yella, P. Gao, R. Humphry-Baker, B. F. E. Curchod, N. Ashari-Astani, I. Tavernelli, U. Rothlisberger, Md. K. Nazeeruddin and M. Grätzel, Nat. Chem., 2014, 6, 242–247 CrossRef CAS PubMed.
- K. Kakiage, Y. Aoyama, T. Yano, T. Otsuka, T. Kyomen, M. Unno and M. Hanaya, Chem. Commun., 2014, 50, 6379–6381 RSC.
- K. Kakiage, Y. Aoyama, T. Yano, K. Oya, T. Kyomen and M. Hanaya, Chem. Commun., 2015, 51, 6315–6317 RSC.
- K. Kakiage, Y. Aoyama, T. Yano, K. Oya, J.-I. Fujisawa and M. Hanaya, Chem. Commun., 2015, 51, 15894–15897 RSC.
- L. Wang, E. W.-G. Diau, M. Wu, H.-P. Lu and T. Ma, Chem. Commun., 2012, 48, 2600–2602 RSC.
- S. Yun, Y. Liu, T. Zhang and S. Ahmad, Nanoscale, 2015, 7, 11877–11893 RSC.
- M.-H. Yeh, S.-H. Chang, L.-Y. Lin, H.-L. Chou, R. Vittal, B.-J. Hwang and K.-C. Ho, Nano Energy, 2015, 17, 241–253 CrossRef CAS.
- J. Burschka, V. Brault, S. Ahmad, L. Breau, M. K. Nazeeruddin, B. Marsan, S. M. Zakeeruddin and M. Grätzel, Energy Environ. Sci., 2012, 5, 6089–6097 CAS.
- E. Olsen, G. Hagen and S. E. Lindquist, Sol. Energy Mater. Sol. Cells, 2000, 63, 267–273 CrossRef CAS.
- G. Syrrokostas, A. Siokou, G. Leftheriotis and P. Yianoulis, Sol. Energy Mater. Sol. Cells, 2012, 103, 119–127 CrossRef CAS.
- H. N. Tsao, J. Burschka, C. Yi, F. Kessler, M. K. Nazeeruddin and M. Grätzel, Energy Environ. Sci., 2011, 4, 4921–4924 CAS.
- S. Ahmad, T. Bessho, F. Kessler, E. Baranoff, J. Frey, C. Yi, M. Grätzel and M. K. Nazeeruddin, Phys. Chem. Chem. Phys., 2012, 14, 10631–10639 RSC.
- H. Wang, Q. Feng, F. Gong, Y. Li, G. Zhou and Z.-S. Wang, J. Mater. Chem. A, 2013, 1, 97–104 CAS.
- B.-W. Park, M. Pazoki, K. Aitola, S. Jeong, E. M. J. Johansson, A. Hagfeldt and G. Boschloo, ACS Appl. Mater. Interfaces, 2014, 6, 2074–2079 CAS.
- Y. Li, Q. Feng, H. Wang, G. Zhou and Z.-S. Wang, J. Mater. Chem. A, 2013, 1, 6342–6349 CAS.
- Y. Li, H. Wang, Q. Feng, G. Zhou and Z.-S. Wang, ACS Appl. Mater. Interfaces, 2013, 5, 8217–8224 CAS.
- Z. Wang, H. Xu, Z. Zhang, X. Zhou, S. Pang and G. Cui, Chin. J. Chem., 2014, 32, 491–497 CrossRef CAS.
- J. He, J. M. Pringle and Y.-B. Cheng, J. Phys. Chem. C, 2014, 118, 16818–16824 CAS.
- S. Shukla, N. H. Loc, P. P. Boix, T. M. Koh, R. R. Prabhakar, H. K. Mulmudi, J. Zhang, S. Chen, C. F. Ng, C. H. A. Huan, N. Mathews, T. Sritharan and Q. Xiong, ACS Nano, 2014, 8, 10597–10605 CrossRef CAS PubMed.
- S. K. Swami, N. Chaturvedi, A. Kumar, R. Kapoor, V. Dutta, J. Frey, T. Moehl, M. Grätzel, S. Mathew and M. K. Nazeeruddin, J. Power Sources, 2015, 275, 80–89 CrossRef CAS.
- L. Kavan, J.-H. Yum, M. K. Nazeeruddin and M. Grätzel, ACS Nano, 2011, 5, 9171–9178 CrossRef CAS PubMed.
- L. Kavan, J.-H. Yum and M. Grätzel, Nano Lett., 2011, 11, 5501–5506 CrossRef CAS PubMed.
- J. D. Roy-Mayhew, G. Boschloo, A. Hagfeldt and I. A. Aksay, ACS Appl. Mater. Interfaces, 2012, 4, 2794–2800 CAS.
- L. Kavan, J.-H. Yum and M. Grätzel, ACS Appl. Mater. Interfaces, 2012, 4, 6999–7006 CAS.
- M. Stefik, J.-H. Yum, Y. Hu and M. Grätzel, J. Mater. Chem. A, 2013, 1, 4982–4987 CAS.
- S. M. Feldt, E. A. Gibson, G. Wang, G. Fabregat, G. Boschloo and A. Hagfeldt, Polyhedron, 2014, 82, 154–157 CrossRef CAS.
- P. Zhai, C.-C. Lee, Y.-H. Chang, C. Liu, T.-C. Wei and S.-P. Feng, ACS Appl. Mater. Interfaces, 2015, 7, 2116–2123 CAS.
- L. Kavan, P. Liska, S. M. Zakeeruddin and M. Grätzel, Electrochim. Acta, 2016, 195, 34–42 CrossRef CAS.
- H. M. Kim, I.-Y. Jeon, I. T. Choi, S. H. Kang, S.-H. Shin, H. Y. Jeong, M. J. Ju, J.-B. Baek and H. K. Kim, J. Mater. Chem. A, 2016, 4, 9029–9037 CAS.
- S. Carli, L. Casarin, Z. Syrgiannis, R. Boaretto, E. Benazzi, S. Caramori, M. Prato and C. A. Bignozzi, ACS Appl. Mater. Interfaces, 2016, 8, 14604–14612 CAS.
- B. Zhao, H. Huang, P. Jiang, H. Zhao, X. Huang, P. Shen, D. Wu, R. Fu and S. Tan, J. Phys. Chem. C, 2011, 115, 22615–22621 CAS.
- G. Kang, J. Choi and T. Park, Sci. Rep., 2016, 6, 22987 CrossRef CAS PubMed.
- C.-T. Li, C.-T. Lee, S.-R. Li, C.-P. Lee, I.-T. Chiu, R. Vittal, N.-L. Wu, S.-S. Sun and K.-C. Ho, J. Power Sources, 2016, 302, 155–163 CrossRef CAS.
- A. Sadezky, H. Muckenhuber, H. Grothe, R. Niessner and U. Pöschl, Carbon, 2005, 43, 1731–1742 CrossRef CAS.
- C. H. A. Wong, A. Ambrosi and M. Pumera, Nanoscale, 2012, 4, 4972–4977 RSC.
- S. H. Seo, M. H. Kim, E. J. Jeong, S. H. Yoon, H. C. Kang, S. I. Cha and D. Y. Lee, J. Mater. Chem. A, 2014, 2, 2592–2598 CAS.
- S. H. Seo, E. J. Jeong, J. T. Han, H. C. Kang, S. I. Cha, D. Y. Lee and G.-W. Lee, ACS Appl. Mater. Interfaces, 2015, 7, 10863–10871 CAS.
- S. Peng, J. Liang, S. G. Mhaisalkar and S. Ramakrishna, J. Mater. Chem., 2012, 22, 5308–5311 RSC.
- Q. Tai, B. Chen, F. Guo, S. Xu, H. Hu, B. Sebo and X.-Z. Zhao, ACS Nano, 2011, 5, 3795–3799 CrossRef CAS PubMed.
- S. Ito, S. M. Zakeeruddin, P. Comte, P. Liska, D. Kuang and M. Grätzel, Nat. Photonics, 2008, 2, 693–698 CrossRef CAS.
- J. J. Nelson, T. J. Amick and C. M. Elliott, J. Phys. Chem. C, 2008, 112, 18255–18263 CAS.
Footnote |
† Electronic supplementary information (ESI) available: Experimental materials and preparation of photoelectrodes; photovoltaic parameters of Z907 cells using various CB counter electrodes; SEM images of FTO and various CB films; equivalent circuit for EIS fitting; Nyquist plots of FTO and STCB-1-H dummy cells; electrochemical stability of CB-1-H and Pt-105 electrodes; stability of a Z907 cell device measured under ambient conditions; photovoltaic J–V curves of a Y123 cell device measured under different irradiation intensities. See DOI: 10.1039/c6ta08818j |
|
This journal is © The Royal Society of Chemistry 2017 |
Click here to see how this site uses Cookies. View our privacy policy here.