DOI:
10.1039/C8NJ03721C
(Paper)
New J. Chem., 2019,
43, 85-92
A novel copper(II) phthalocyanine-modified multiwalled carbon nanotube-based electrode for sensitive electrochemical detection of bisphenol A†
Received
31st July 2018
, Accepted 7th November 2018
First published on 15th November 2018
Abstract
A novel copper(II) phthalocyanine (CuPc)-modified multiwalled carbon nanotube-based electrode was prepared for the sensitive electrochemical detection of bisphenol A, by the modification of a pencil graphite electrode via the adsorption method. The synthesized novel CuPc was characterized by spectroscopic methods and the electrodes were characterized by electrochemical, spectroscopic and microscopic methods. The limit of detection (LOD, S/N = 3) was calculated as 0.0189 μM for bisphenol A. The experimental parameters such as pH and adsorption time were optimized for the electrochemical detection of bisphenol A. The detection was done in real samples, with a high recovery value (>98%), indicating the useful application of modified electrodes as electrochemical sensors for bisphenol A. The electrodes showed excellent repeatability and stability in the differential pulse voltammetric detection of bisphenol A. The prepared novel sensor can be used for the detection of bisphenol A in many environmental analyses due to its simplicity, low cost, environmental friendliness and high sensitivity.
1. Introduction
Bisphenol A (BPA) is an organic compound that is used as a monomer for the production of polycarbonate and a precursor of epoxy resins; it is also known as 4,4′-(propane-2,2-diyl)diphenol, which is its IUPAC name.1,2 Since BPA has been used for the industrial production of food contact materials, it has become a great risk factor in human health.3,4 Besides, BPA contamination in the environment during the production and recycling process has emerged an important risk to the ecosystem.2,5,6 The sensitive and selective detection of BPA has received great attention from researchers for monitoring its levels in environmental, biological and food samples due to the harmful effects of BPA on the health of the ecology and humans.7,8 The detection of BPA can be done through various methods such as spectroscopy, chromatography, and electrochemistry.9–11 Among these methods, electrochemical methods have great advantages due to their superiorities such as high sensitivity, high selectivity, and relatively low cost.12,13 Potentiometric, amperometric and voltammetric methods have been used in the electrochemical detection of BPA.14–16 The modification of used electrodes in electrochemical methods is important for improving the selectivity and sensitivity of BPA detection.
Since the first day they were discovered, multiwalled carbon nanotubes (MWCNT) have been useful electrode materials due to their unique characteristics, large surface areas, electrical and chemical properties, high stabilities and strong adsorption behaviors.17,18 These extraordinary features of MWCNT allow them to be used in many applications such as electrochemical sensors. Because MWCNTs can be easily adsorbed on a bare electrode surface, they have been extensively investigated as modified agents for electrochemical sensor applications.19,20
Phthalocyanines (Pc) constitute a notable class of chemicals that have been applied as molecular materials due to their rich redox behavior, architectural flexibility, their high thermal and chemical stabilities, diverse coordination properties and improved spectroscopic characteristics for electrochemical sensor applications.21–23 Among the phthalocyanines containing metals, copper phthalocyanines (CuPc) have been used as efficient electrochemical sensors for the construction of new electrode materials.19 Pc-modified electrodes have been employed by many research groups through electropolymerization, physical adsorption or drop-casting of the Pc moiety on a bare electrode surface.24–26 Phthalocyanine-based materials were used by some groups to obtain advanced electrodes for the electrochemical detection of BPA.27–29 In recent years, researchers have shown that Pc-MWCNTs-based electrodes exhibit improved electrochemical responses when compared to MWCNT or Pc electrodes alone.30,31
In this study, a pencil graphite electrode (PGE) was modified by multiwalled carbon nanotubes (MWCNT-COOH) and CuPc to obtain a novel electrochemical sensor (CuPc/MWCNT-COOH/PGE) for the detection of bisphenol A (BPA). The structures of the synthesized compounds were investigated by elemental analysis, FT-IR, UV-Vis, 1H-NMR, 13C-NMR and MS techniques. The prepared CuPc/MWCNT-COOH/PGE electrode was characterized by electrochemical methods such as cyclic voltammetry, differential pulse voltammetry, and electrochemical impedance spectroscopy. The structural features of the prepared electrode were also examined by Scanning Electron Microscopic (SEM) analysis in each step of the modification. In addition, X-ray photoelectron spectroscopy (XPS) and Transmission electron microscopy (TEM) techniques were used for characterization. The parameters affecting the electrochemical response of the electrodes such as pH, adsorption time and interferences were investigated by electrochemical methods. The prepared sensor showed a linear range of 2.75 × 10−5 to 1.0 × 10−7 M with a 0.0189 μM limit of detection (LOD, S/N = 3). The recovery values were higher than 98% for three different real samples, which also supports the use of this novel sensor for the electrochemical detection of BPA in environment and food samples.
2. Experimental
2.1. Reagents and apparatus
Multiwalled carbon nanotubes (MWCNT) (D × L 110–170 nm × 5–9 μm, >90%), N,N-dimethylformamide (≥99%), phosphoric acid (>85%), potassium hydroxide (>85%), potassium hexacyanoferrate(III) (≥%99), potassium hexacyano-ferrate(II)trihydrate (≥%99), methanol (≥99.7%), bisphenol-A (≥99%), sulfuric acid (>95–97%), nitric acid (>70%), trans-4-hydroxy-3-methoxycinnamic acid (ferulic acid) (>99%), 4-nitrophthalonitrile(>99%), 1,8-diazabicyclo[5.4.0]undec-7-ene (DBU) (>98%) and copper(II) acetate (>98%) were purchased from Sigma-Aldrich. Potassium dihydrogen phosphate (≥98%), dipotassium hydrogen phosphate (≥98%), potassium hydroxide (≥85%), chloroform (≥99%), ethanol (>96%), n-pentanol (≥99%), potassium carbonate (>99%), hydrochloric acid (>37%), acetone (≥99%) and tetrahydrofuran (≥99%) were purchased from Merck. All substances were directly used without any purification. Plastic bottled water samples, which were used in real sample analysis, were purchased at a local market. The carbon-based electrode was a pencil graphite electrode (Tombow, HB, 0.5 mm). Electrochemical measurements were carried out via the Autolab PGSTAT 128N model and Gamry Reference 3000 model potentiostat/galvanostat at room temperature. Solutions were prepared with ultra-pure water (obtained by MILLIPORE Milli-Q Direct 16 model apparatus). The pH of solutions was determined using a HANNA HI 2211 pH/ORP meter. Scanning electron microscope (SEM) images of all electrodes were obtained using Zeiss-Ultraplus model equipment. A Tecnai TM G2 Spirit model (FEI Company) instrument was used for transmission electron microscope (TEM) analysis. The pictures were taken of powders of modified and unmodified PGE dispersed on a Cu grid at 87
000× magnification. Elemental analysis was carried out by a LECO CHNS 932 instrument. The UV-Vis spectra were recorded on an Agilent 8453 UV/Vis spectrophotometer. FT-IR analyses were conducted on Perkin Elmer Spectrum One model equipment. Nuclear magnetic resonance (NMR) spectra of the synthesized substances were obtained using Bruker Avance III 500 MHz equipment. LC/MS and MALDI-TOF MS spectra of products were obtained on Shimadzu LCMS-8030 Plus and Bruker Microflex LT MALDI-TOF MS model equipment, respectively.
2.2. Chemical preparation of modified MWCNT
Chemical modification of MWCNT was done to obtain –COOH groups on the surface of the nanotubes. For this process, 1.0 mg of MWCNT was treated with HNO3
:
H2SO4 (1
:
3) solution using an ultrasonic mixer at 40 °C for 6 hours (Fig. S8, ESI†). The obtained solid particles were washed three times with ultrapure water to remove all of the acid from the solution. The modified solid particles were filtered and dried. The final products were dispersed in DMF solution in an ultrasonic mixer for 15 min.32 The synthesis procedures (Fig. S1 and S2, ESI†) and characterization results (Fig. S3–S5, ESI†) of (E)-3-(4-(3,4-dicyanophenoxy)-3-methoxyphenyl)acrylic acid (1) and (E)-3-(2,9,16,23-tetrakis-(4-oxy-3-methoxyphenyl)acrylic acid)phthalociyaninato copper(II) (2) are given in the ESI.†
2.3. Preparation of modified electrodes
CuPc modified multiwalled carbon nanotube-based electrodes were used for the sensitive electrochemical detection of BPA. A pencil graphite electrode (PGE) was modified by adsorption of MWCNT-COOH and a novel CuPc compound. Firstly, MWCNT-COOH dispersed in DMF (5.0 mg/1.0 mL) was dropped on the PGE surface and the electrode was dried at room temperature. The electrode was then immersed in methanol solution containing the synthesized novel CuPc compound for adsorption on the electrode surface; the product was called CuPc/MWCNT-COOH/PGE. The amount of MWCNT-COOH was optimized by using different amount (3, 5, 7, 10 and 20 μL) in dropping solutions. The adsorption time was also optimized to obtain the best analytical response for BPA in DPV analysis.
2.4. Electrochemical characterization parameters of CuPc/MWCNT-COOH/PGE
Cyclic voltammetry (CV) and electrochemical impedance spectroscopy (EIS) were used to investigate the electrochemical behaviors of the bare and modified electrodes. The electrodes were characterized in a 0.1 M KCl and 2.5 mM Fe(CN)63−/4− solution over the potential range of 0.1 and 0.9 V versus Ag/AgCl (in 3.0 M KCl) with a 100 mV s−1 scan rate by the cyclic voltammetric method. The solution resistance (Rs), charge transfer resistance (Rct) and Warburg impedance values were investigated by EIS analysis. Electrochemical impedance spectroscopy analysis was conducted at an open circuit potential in a 105 to 10−2 Hz frequency range at an amplitude of 10 mV in 0.1 M KCl and 10.0 mM Fe(CN)63−/4− solutions. All the spectra were fitted with an equivalent circuit model as shown in Fig. 1b (inset).
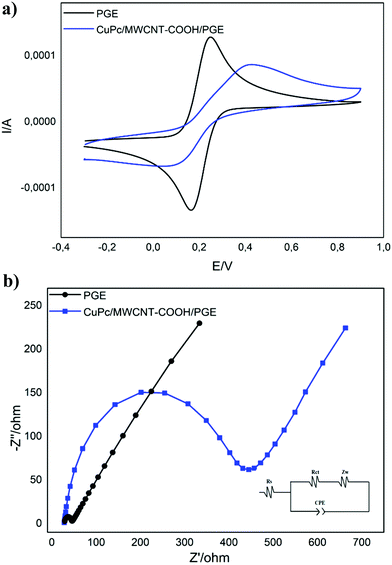 |
| Fig. 1 (a) Cyclic voltammograms (in 0.1 M KCl and 2.5 mM Fe(CN)63−/4− solutions); (b) fitted electrochemical impedance spectra of PGE and CuPc/MWCNT-COOH/PGE (inset: equivalence circuit model in 0.1 M KCl and 10 mM Fe(CN)63−/4− solutions). | |
2.5. Electrochemical detection of BPA
Plastic bottled water samples, which are commercially available in the local market, were used without any pretreatment; 1.0 mL of each water sample was diluted to 100 mL with phosphate buffer solution (pH 2.0) for analysis.
3. Results and discussion
3.1. Characterization of CuPc/MWCNT-COOH/PGE
Cyclic voltammetric and electrochemical impedance spectroscopic analyses were carried out for the electrochemical characterization of CuPc/MWCNT-COOH/PGE. The electrodes were characterized in a solution consisting of 0.1 M KCl and 2.5 mM Fe(CN)63−/4−. Fig. 1a shows the cyclic voltammograms of PGE and CuPc/MWCNT-COOH/PGE in the 0.1–0.9 V potential range. The anodic and cathodic peak potentials for PGE were determined as 0.25 and 0.17 V, respectively (Fig. 1a), and were 0.42 and 0.11 V, respectively, for CuPc/MWCNT-COOH/PGE. The reversibility and current of the peaks in PGE were higher than in CuPc/MWCNT-COOH/PGE (Fig. 1a). This was probably due to electrostatic interactions of carboxyl acid groups on the surface of the modified electrode with Fe(CN)64−/3− groups, indicating a successful surface modification.33,34Fig. 1b shows the fitted electrochemical impedance spectra of PGE and CuPc/MWCNT-COOH/PGE. Rs, Rct, Zw, and CPE represent the solution resistance, charge transfer resistance, Warburg impedance and capacitance, respectively.35,36Rs, also known as ohmic resistance, includes the resistance of electrolytes, the resistance of corrosion products located on the surface of the electrode, and the resistance of the electrical connections to the electrode. Rct is a parameter related to the resistance of the rate controlling the electrochemical reaction of the corrosion process. Zw also corresponds to the rate of mobile ion transfer to the electrode surface.37,38 The Rs values were almost the same due to same experimental conditions for PGE and modified electrodes (Fig. 1b). Rct values were 19.1 and 392.8 Ω for PGE and CuPc/MWCNT-COOH/PGE, respectively (Fig. 1b). Here, the electron transfer in the electrochemical reaction is more difficult on the modified electrode surface than that of PGE. This also showed that the surface modification of PGE by carboxylic acid groups pushed the negatively charged ions of the electrolyte. The Zw value was the lowest in CuPc/MWCNT-COOH/PGE, indicating a low diffusion rate of mobile ions to the porous surface modified electrode due to the electrostatic pushing effects of the negatively charged surface.39 FT-IR spectroscopy was used in the characterization of functional groups of the modified electrode surface (see the ESI,† Fig. S6). SEM analyses were done to determine the structural features of modified electrodes and the unmodified electrode. Graphitic flakes including the surface of PGE can be seen easily in Fig. 2a. –COOH modified MWCNT were adsorbed on the surface of PGE and separated homogeneously (Fig. 2b–d). After the last modification step with CuPc, the organometallic complex was adsorbed on the surface of the MWCNT-COOH/PGE (Fig. 2e and f). SEM analysis also supported the successful surface modification of PGE with MWCNT-COOH/CuPc. TEM images of PGE and CuPc/MWCNT-COOH/PGE can be seen in Fig. S7 (ESI†). The changes on the surface of PGE can be easily seen in Fig. S7b. Although no nano-particles can be seen in the PGE (Fig. S7a, ESI†), certain nanostructures, which are related to CuPc/MWCNT-COOH, can be seen in the modified electrode (Fig. S7b, ESI†).
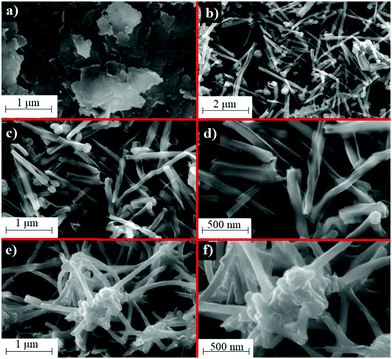 |
| Fig. 2 SEM images of (a) PGE with 25 000×, (b) MWCNT-COOH/PGE with 25 000×, (c) MWCNT-COOH/PGE with 50 000×, (d) MWCNT-COOH/PGE with 100 000×, (e) CuPc/MWCNT-COOH/PGE with 50 000×, and (f) CuPc/MWCNT-COOH/PGE with 100 000×. | |
3.2. Electrochemical detection of BPA
The modification process is of great importance in modified electrode-based sensor studies.40–43 The main purpose of the modification is to selectively improve the analytical response of modified electrodes to the analyte. Cyclic voltammetric analyses were carried out between 0.4–1.3 V in each step of the modification to investigate the electrochemical response of the electrodes to BPA. Fig. 3 shows the cyclic voltammograms of PGE, CuPc/PGE, MWCNT-COOH/PGE and CuPc/MWCNT-COOH/PGE. The obtained oxidation peak around 0.8 V corresponds to the oxidation of BPA in Fig. 3, because the PGE and CuPc/MWCNT-COOH/PGE showed no peak at this potential in the blank solution (Fig. 3). The highest anodic peak current was determined with CuPc/MWCNT-COOH/PGE. This result may be caused by the catalytic oxidation effects of the modified surface PGE by CuPc/MWCNT-COOH. A plausible oxidation reaction was proposed in eqn (1)–(3) according to the literature.44,45 Cu(II)Pc was reduced Cu(I)Pc during the oxidation of BPA (eqn (1)). The formed oxidized form of BPA probably had two tautomer forms as Intermediate I and Intermediate II in eqn (2). The bifurcated oxidation peaks were probably tautomeric transformations of two radical forms (eqn (2) and Fig. 3). In the last step, quinoid products of BPA were formed (eqn (3)). Since MWCNT-COOH/Cu(II)Pc modified PGE catalyzed the oxidation of BPA, the highest anodic peak was determined in this electrode. |  | (1) |
| 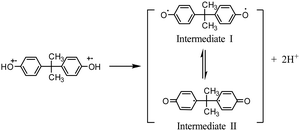 | (2) |
|  | (3) |
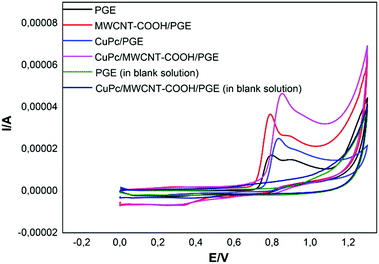 |
| Fig. 3 Cyclic voltammograms of PGE, CuPc/PGE, MWCNT-COOH/PGE and CuPc/MWCNT-COOH/PGE in 0.1 mM BPA solution (pH 2.0 buffer solution; scan rate: 100 mV s−1), and, PGE and CuPc/MWCNT-COOH/PGE in the blank solution. | |
To determine the mass transfer mechanism as adsorption or diffusion, the scanning rate using cyclic voltammetry with the CuPc/MWCNT-COOH/PGE electrode was changed from 5 to 500 mV s−1 and this is shown in Fig. 4. When the anodic peak current was plotted against the square root of the scan rates, a linear correlation was observed (Fig. 4 inset). This result supported the diffusion-controlled mass transfer mechanism for the reaction. This was also important for sensor application.37,38,45
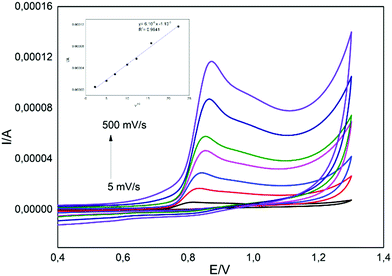 |
| Fig. 4 Cyclic voltammograms of CuPc/MWCNT-COOH/PGE in 1.0 mM BPA solution (pH 2.0 buffer solution) with different scanning rates (inset: the plot of anodic peak current against the square root of the scan rates). | |
Differential pulse voltammetry (DPV) was used to determine the analytical response of the electrodes for BPA. Fig. S9 (ESI†) shows the differential pulse voltammograms of PGE, CuPc/PGE, MWCNT-COOH/PGE and CuPc/MWCNT-COOH/PGE. The analytical response of the oxidation peak of BPA increased from PGE to CuPc/MWCNT-COOH/PGE. Since the modified electrode has the –COOH group, the selectivity and interactions of the analyte and electrodes increased in the modified materials. Besides, the activated surface of PGE during the modification process probably had a catalytic effect on the oxidation reaction of BPA (eqn (1)–(3)).44,45 In addition, each modification step caused an increase in the oxidation peak of BPA in DPV analysis (Fig. S9, ESI†). CuPc/PGE and MWCNT-COOH/PGE also has a relatively high analytical response for BPA in DPV analysis. The success of modification by adsorption was also confirmed by the obtained voltammograms in Fig. S9 (ESI†) and each modification step increased the selectivity of the electrodes for BPA.
3.3. Optimization of adsorption conditions and pH for the electrochemical detection of BPA
Adsorption conditions and the effect of pH were optimized to improve the electrochemical response of the modified electrodes for BPA. The amount of modifier and adsorption time were optimized using the analytical response of BPA. Different amounts (0.01, 0.1 and 1.0 mM) of CuPc were dissolved in methanol and these solutions were used in the optimization of the amount of modifier. The best analytical response was obtained by the time the concentration was 1.0 mM. In this amount of modifier, the electrode surface probably fully adsorbed the CuPc for the selective determination of BPA.46 When the concentration of CuPc increased from 0.01 to 1.0 mM, a larger amount of CuPc was probably adsorbed on the surface of MWCNT-COOH/PGE. Another crucial factor, which affects the selectivity and response of electrode, is the thickness of the film on the surface of the electrodes.42,43,47 Different adsorption times (1, 5, 10, 20 and 25 min) were studied to determine the effects of the adsorbed amount of CuPc and the thickness of the formed modified layers. The results obtained in DPV analysis for BPA with CuPc/MWCNT-COOH/PGEs, which were obtained at different adsorption times, are given in Fig. S10 (ESI†). When the adsorption time in the 1.0 mM CuPc solution was 10 min, the highest analytical response was determined in the DPV analysis (Fig. S9, ESI†). The higher or lower adsorption time than that of 10 min negatively affected the analytical response of the modified electrodes for BPA. The result may be explained by the interactions of CuPc molecules during the adsorption process. The entire surface was probably not modified by CuPc for adsorption times lower than 10 min. On the other hand, CuPc molecules probably formed a thicker film on the surface MWCNT-COOH/PGE, which also prevented the diffusion of BPA on the electrode surface due to steric factors.42,48
pH is another important parameter in the electrochemical sensor studies.40 To determine the effects of pH on the electrochemical detection of BPA with CuPc/MWCNT-COOH/PGE, the DPV analysis was carried out in 0.1 mM BPA solutions at different pH values (2, 3, 4, 5, 6 and 7). The effect of pH on the oxidation peak of BPA obtained with differential pulse voltammograms can be seen in Fig. 5. When the pH was changed from 2 to 7, the analytical response of electrodes decreased to pH 4.0, then increased to pH 7.0. This result showed that the amount of hydronium plays a key role in the oxidation of BPA (Fig. 5 inset).49,50 Besides, the potential of the oxidation peaks shifted to a greater cathodic area with increasing pH, indicating the electrocatalytic effects of decreasing hydronium concentration (Fig. 5 inset).49,50 This was probably related to the oxidation reaction of BPA (eqn (1)–(3)). Particularly, decreasing the concentration of hydronium by increasing the pH is supported by eqn (2), indicating the consumption of the hydronium formed. The slope of the equation, which was given as −55.7 mV pH−1 in Fig. 5 inset, is very close to the theoretical value of −57.6 mV pH−1.49–51 This also supported that the electron transfer occurs with an equal number of protons in the electrode reaction.51 On the other hand, the analytical response of the electrode at pH 2.0 for the oxidation of BPA was the highest (Fig. 5 inset). When the pH of the solution was 2, the surface of the modified electrode was probably activated to the selective oxidation of BPA by hydronium ions. This also improves the response of modified electrodes to BPA.49,50 The pH of BPA solutions was chosen as 2 for further analysis.
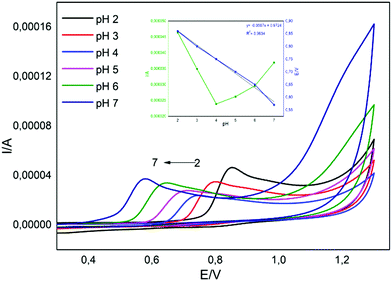 |
| Fig. 5 Cyclic voltammograms of CuPc/MWCNT-COOH/PGE in 1.0 mM BPA solutions prepared at different pH values (2, 3, 4, 5, 6 and 7; inset: anodic peak current and peak potential). | |
3.4. Effect of interferences on the electrochemical detection of BPA
Many different substances and ions such as methanol, phenol, SO42−, Na+, Cl−, Cu2+, Fe3+, Pb2+, K+, Cd2+, etc., can be used in the production and structure of food packaging materials.52,53 To determine the effects of these substances on the electrochemical detection of BPA, we studied the change in current with adding them to the BPA solutions in the DPV analysis (Fig. S12, ESI†). Sodium chloride, sodium sulfate and methanol (0.01 M each) were separately added to the 0.01 mM of BPA solutions. Since these substances were electrochemically inactive in the potential range studied, any changes in the oxidation current of BPA in DPV analysis were observed (Fig. S12a, ESI†). The concentration of each interference was more than 1000 times greater than the BPA concentration in the determination of the interference effect in the DPV analysis (Fig. S12a, ESI†). Methanol (0.001 M) was also added to the 0.01 mM BPA solutions and a decrease in the anodic peak current was observed, lower than that of 5% (Fig. S12a, ESI†). The effects of 0.1 M of Cu2+, Fe3+, Pb2+, K+, and Cd2+, which have been used in many industrial processes and are available in some water samples, were also studied for the analytical response of BPA using the modified electrode in DPV analysis, given in Table 1 (Fig. S12b, ESI†). This showed that CuPc/MWCNT-COOH/PGE has great selectivity for BPA and can be used in the electrochemical detection and monitoring of BPA in many samples.
Table 1 Interference effects of some ions on the oxidation peak of 0.01 mM of BPA (pH 2.0 buffer solution)
Species (1 mM) |
Current (μA) |
Current differences (μA) |
%RSD |
— |
148.39 |
— |
— |
Cu2+ |
147.56 |
−0.83 |
2.94 |
Fe3+ |
149.92 |
1.53 |
3.18 |
Pb2+ |
147.14 |
−1.25 |
3.32 |
K+ |
149.81 |
1.42 |
2.97 |
Cd2+ |
149.74 |
1.35 |
2.16 |
3.5. Calibration curve and analytical parameters for the electrochemical detection of BPA
The linear range, limit of detection (LOD), repeatability and stability of electrochemical sensors are crucial properties for their potential applications.39,42,43 The calibration curve method was used for the determination of BPA by the developed novel sensor as CuPc/MWCNT-COOH/PGE. The voltammograms obtained on increasing the concentration of BPA from 1.0 × 10−7 to 1.0 × 10−4 M are given in Fig. 6. The analytical response of the oxidation peak of BPA increased as a function of BPA concentration (Fig. 6). When the obtained oxidation peak currents were plotted to the concentration of PBA, two different linear ranges were determined between 2.75 × 10−5 to 1.0 × 10−4 M (Fig. 6 inset) and 2.75 × 10−5 to 1.0 × 10−7 M (Fig. 6 inset). The lower linear range was used for the electrochemical detection of BPA since the concentration of BPA was low in the studied samples. The LOD (S/N = 3) was calculated as 0.0189 μM for BPA using CuPc/MWCNT-COOH/PGE according to the equation (given in Fig. 6 inset) with the 2.75 × 10−5 to 1.0 × 10−7 M linear range. The comparison of the calculated limit of detection and studied linear range with the literature is given in Table 2. The developed method showed better performance with its high sensitivity to BPA as compared to those of many reported studies in the literature (Table 2). The repeatability of the prepared electrodes was studied by preparing ten electrodes. They were used in the DPV analysis of the same solutions and the RSD% (N = 10) was 3.42, indicating enhanced repeatability for modified electrodes. The analytical response of the modified electrode was lower by only 4% at the end of the 60th day as compared to the first day, which was also related to the high stability of CuPc/MWCNT-COOH/PGE.
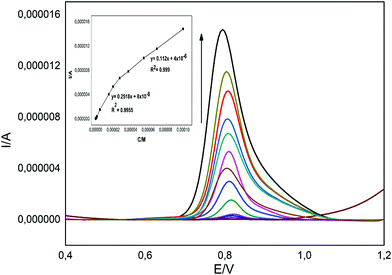 |
| Fig. 6 Differential pulse voltammograms of solutions consisting of different concentrations of BPA (inset: plot of anodic peak current to BPA concentration). | |
Table 2 Comparison of the proposed method with some of the previously reported ones
Electrode |
Technique/method |
Linear range (μM) |
Limit of detection (LOD)/μM |
Ref. |
PGE/NiPcTS |
DPV |
0.5–10.0 |
0.029 |
45
|
ITO |
DPV |
5.0–120.0 |
0.290 |
11
|
Cathodically pretreated BDD |
DPV |
0.44–5.2 |
0.210 |
54
|
Laccase–thionin–carbon black-modified screen printed electrode |
Amperometry |
0.5–50 |
0.2 |
55
|
Thionin–tyrosinase/CPE |
Amperometry |
0.15–45 |
0.15 |
56
|
EC(CYP2C9-PAM/GCE) |
Amperometry |
1.25–10 |
0.58 |
57
|
PEDOT/GCE |
CV and Amperometry |
55 and 22 |
90–410 and 40–410 |
58
|
CuPc/MWCNT-COOH/PGE |
DPV |
0.1–27.5 |
0.0189 |
This work |
3.6. Electrochemical detection of BPA in real samples
The electrochemical detection of BPA in real water samples, purchased in plastic bottles from a local market, was conducted with CuPc/MWCNT-COOH/PGE using DPV. The water samples were directly analyzed by DPV with CuPc/MWCNT-COOH/PGE without any other procedure in the pH 2.0 buffer solutions. BPA (5.0 μM) was added to three different samples and the solutions were analyzed with CuPc/MWCNT-COOH/PGE by the DPV method. The results obtained are given in Table 3. Each measurement was repeated five times and the RSD% was calculated. The recovery values were greater than 98% for the three samples (Table 3). The results showed that the methods developed using novel CuPc/MWCNT-COOH/PGE can be used for the sensitive detection of BPA.
Table 3 Electrochemical detection of CuPc/MWCNT-COOH/PGE in real samples
Sample ID |
Initial concentration of BPA (μM) |
Added concentration of BPA (μM) |
Determined concentration of BPA (μM) |
RSD (%) (n = 5) |
Recovery (%) |
1 |
0 |
5.0 |
4.984 |
3.87 |
98.00 |
2 |
0 |
5.0 |
4.987 |
3.94 |
99.60 |
3 |
0 |
5.0 |
4.985 |
3.54 |
99.78 |
4. Conclusion
In this work, a novel CuPc was synthesized and characterized in the literature for the first time. The prepared novel compound was used as a modifier for activated groups including multiwalled carbon nanotubes (MWCNT-COOH) on the PGE to obtain a novel electrochemical sensor for BPA. Spectroscopic, electrochemical and microscopic techniques were used in the characterization of the synthesized novel compound and prepared novel electrode. CuPc/MWCNT-COOH/PGE was used as an electrochemical sensor for BPA. The electrode has a linear range analytical response, 2.75 × 10−5 to 1.0 × 10−7 M, with a 0.0189 μM limit of detection in DPV analysis. pH and adsorption time were optimized for the electrochemical detection of BPA to obtain the highest electrochemical response. Any interferences effects were observed for the prepared BPA sensor in DPV analysis. The recovery value in the real sample analyses was greater than 98%, indicating the plausible application of CuPc/MWCNT-COOH/PGE as a sensor for BPA.
Conflicts of interest
There are no conflicts to declare.
Acknowledgements
We express our sincere thanks to financial support from the Yildiz Technical University Scientific Research Projects Coordination Department (Project No. FDK-2017-3130).
References
- C. A. Staples, P. B. Dom, G. M. Klecka, T. O. Sandra and L. R. Harris, Chemosphere, 1998, 36, 2149–2173 CrossRef CAS
.
- A. Ballesteros-Gómez, S. Rubio and D. Pérez-Bendito, J. Chromatogr. A, 2009, 1216, 449–469 CrossRef PubMed
.
- J. H. Kang, F. Kondo and Y. Katayama, Toxicology, 2006, 226, 79–89 CrossRef CAS PubMed
.
- J. R. Rochester, Reprod. Toxicol., 2013, 42, 132–155 CrossRef CAS
.
- D. L. Villeneuve, N. Garcia-Reyero, B. L. Escalon, K. M. Jensen, J. E. Cavallin, E. A. Makynen, E. J. Durhan, M. D. Kahl, L. M. Thomas, E. J. Perkins and G. T. Ankley, Environ. Sci. Technol., 2012, 46, 51–59 CrossRef CAS
.
- J. H. Kang, D. Aasi and Y. Katayama, Crit. Rev. Toxicol., 2007, 37, 607–625 CrossRef CAS PubMed
.
- N. Caballero-Casero, L. Lunar and S. Rubio, Anal. Chim. Acta, 2016, 908, 22–53 CrossRef CAS PubMed
.
- K. V. Ragavan, N. K. Rastogi and M. S. Thakur, TrAC, Trends Anal. Chem., 2013, 52, 248–260 CrossRef CAS
.
- K. Inoue, A. Yamaguchi, M. Wada, Y. Yoshimura, T. Makino and H. Nakazawa, J. Chromatogr. B: Biomed. Sci. Appl., 2001, 765, 121–126 CrossRef CAS
.
- S. Poorahong, C. Thammakhet, P. Thavarungkul and P. Kanatharana, J. Environ. Sci. Health, Part A: Toxic/Hazard. Subst. Environ. Eng., 2013, 48, 242–250 CrossRef CAS
.
- Q. Li, H. Li, G. F. Du and Z. H. Xu, J. Hazard. Mater., 2010, 180, 703–709 CrossRef CAS PubMed
.
- K. Varmira, M. Saed-Mocheshi and A. R. Jalalvand, Sens. Biosensing Res., 2017, 15, 17–33 CrossRef
.
- A.-N. Kawde, N. Baig and M. Sajid, RSC Adv., 2016, 6, 91325–91340 RSC
.
- L.-J. Kou, R.-N. Liang, X.-W. Wang, Y. Chen and W. Qin, Anal. Bioanal. Chem., 2013, 405, 4931–4936 CrossRef CAS
.
- S. Poorahong, C. Thammakhet, P. Thavarungkul, W. Limbut, A. Numnuam and P. Kanatharana, Microchim. Acta, 2012, 176, 91–99 CrossRef CAS
.
- X. Dong, X. Qi, N. Liu, Y. Yang and Y. Piao, Sensors, 2017, 17, 836 CrossRef
.
- S. Iijima, Nature, 1991, 354, 56–58 CrossRef CAS
.
- R. H. Baughman, Science, 2002, 297, 787–792 CrossRef CAS
.
- F. C. Moraes, L. H. Mascaro, S. A. S. Machado and C. M. A. Brett, Electroanalysis, 2010, 22, 1586–1591 CAS
.
- X. Zuo, N. Li and H. Zhang, J. Mater. Sci., 2012, 47, 2731–2735 CrossRef CAS
.
- H. Hosseini, M. Mahyari, A. Bagheri and A. Shaabani, Biosens. Bioelectron., 2014, 52, 136–142 CrossRef CAS PubMed
.
- F. D’Souza, J. Porphyrins Phthalocyanines, 2002, 06, 285–288 CrossRef
.
- S. Gorduk, O. Avciata and U. Avciata, Inorg. Chim. Acta, 2018, 471, 137–147 CrossRef CAS
.
- K. I. Ozoemena, J. Pillay and T. Nyokong, Electrochem. Commun., 2006, 8, 1391–1396 CrossRef CAS
.
- I. S. Da Silva, M. F. A. Araújo, H. A. Ferreira, J. D. J. G. Varela, S. M. C. N. Tanaka, A. A. Tanaka and L. Angnes, Talanta, 2011, 83, 1701–1706 CrossRef
.
- R. de, C. S. Luz, A. B. Moreira, F. S. Damos, A. A. Tanaka and L. T. Kubota, J. Pharm. Biomed. Anal., 2006, 42, 184–191 CrossRef
.
- H. S. Yin, Y. L. Zhou and S. Y. Ai, J. Electroanal. Chem., 2009, 626, 80–88 CrossRef CAS
.
- V. Chauke, F. Matemadombo and T. Nyokong, J. Hazard. Mater., 2010, 178, 180–186 CrossRef CAS
.
- H. Yin, Y. Zhou, J. Xu, S. Ai, L. Cui and L. Zhu, Anal. Chim. Acta, 2010, 659, 144–150 CrossRef CAS
.
- B. O. Agboola, S. L. Vilakazi and K. I. Ozoemena, J. Solid State Electrochem., 2009, 13, 1367–1379 CrossRef CAS
.
- F. C. Moraes, M. F. Cabral, S. A. S. Machado and L. H. Mascaro, Electroanalysis, 2008, 20, 851–857 CrossRef CAS
.
- M. S. Cosio, A. Pellicanò, B. Brunetti and C. A. Fuenmayor, Sens. Actuators, B, 2017, 246, 673–679 CrossRef CAS
.
- R. Pei, Z. Cheng, E. Wang and X. Yang, Biosens. Bioelectron., 2001, 16, 355–361 CrossRef CAS
.
- V. Molinero and E. J. Calvo, J. Electroanal. Chem., 1998, 445, 17–25 CrossRef CAS
.
- H. Gürsu, M. Gençten and Y. Şahin, Electrochim. Acta, 2017, 243, 239–249 CrossRef
.
- M. Gençten, K. B. Dönmez, Y. Şahin, K. Pekmez and E. SuvacI, J. Solid State Electrochem., 2014, 18, 2469–2479 CrossRef
.
- O. Koyun and Y. Sahin, Ionics, 2018, 1–11, DOI:10.1007/s11581-017-2429-7
.
- O. Koyun and Y. Sahin, Int. J. Electrochem. Sci., 2018, 13, 159–174 CrossRef CAS
.
- O. Koyun, H. Gursu, S. Gorduk and Y. Sahin, Int. J. Electrochem. Sci., 2017, 12, 6428–6444 CrossRef CAS
.
- Y. R. Kim, S. Bong, Y. J. Kang, Y. Yang, R. K. Mahajan, J. S. Kim and H. Kim, Biosens. Bioelectron., 2010, 25, 2366–2369 CrossRef CAS
.
- X. Luo, A. Morrin, A. J. Killard and M. R. Smyth, Electroanalysis, 2006, 18, 319–326 CrossRef CAS
.
- L. Özcan and Y. Şahin, Sens. Actuators, B, 2007, 127, 362–369 CrossRef
.
- L. Özcan, M. Sahin and Y. Sahin, Sensors, 2008, 8, 5792–5805 CrossRef
.
- N. Ben Messaoud, M. E. Ghica, C. Dridi, M. Ben Ali and C. M. A. Brett, Sens. Actuators, B, 2017, 253, 513–522 CrossRef CAS
.
- L. Özcan, M. Altuntaş, A. Büyüksağiş, H. Türk and S. Yurdakal, Anal. Sci., 2016, 32, 881–886 CrossRef
.
- X. Kang, J. Wang, H. Wu, I. A. Aksay, J. Liu and Y. Lin, Biosens. Bioelectron., 2009, 25, 901–905 CrossRef CAS
.
- S. Cosnier, Biosens. Bioelectron., 1999, 14, 443–456 CrossRef CAS
.
- K. Grennan, A. J. Killard, C. J. Hanson, A. A. Cafolla and M. R. Smyth, Talanta, 2006, 68, 1591–1600 CrossRef CAS
.
- H. Yin, Y. Zhou, L. Cui, X. Liu, S. Ai and L. Zhu, J. Solid State Electrochem., 2011, 15, 167–173 CrossRef CAS
.
- Z. Zheng, J. Liu, M. Wang, J. Cao, L. Li, C. Wang and N. Feng, J. Electrochem. Soc., 2016, 163, B192–B199 CrossRef CAS
.
- Q. Wang, Y. Wang, S. Liu, L. Wang, F. Gao, F. Gao and W. Sun, Thin Solid Films, 2012, 520, 4459–4464 CrossRef CAS
.
- D. W. K. Barnes and R. Sinclair, Chem. Migr. Food Contact Mater., 2007, 180–202 Search PubMed
.
- E. Fasano, F. Bono-Blay, T. Cirillo, P. Montuori and S. Lacorte, Food Control, 2012, 27, 132–138 CrossRef CAS
.
- G. F. Pereira, L. S. Andrade, R. C. Rocha-Filho, N. Bocchi and S. R. Biaggio, Electrochim. Acta, 2012, 82, 3–8 CrossRef CAS
.
- M. Cammarota, M. Lepore, M. Portaccio, D. Di Tuoro, F. Arduini, D. Moscone and D. G. Mita, Electrochim. Acta, 2013, 109, 340–347 CrossRef
.
- M. Portaccio, D. Di Tuoro, F. Arduini, M. Lepore, D. G. Mita, N. Diano, L. Mita and D. Moscone, Biosens. Bioelectron., 2010, 25, 2003–2008 CrossRef CAS
.
- P. Sun and Y. Wu, Sens. Actuators, B, 2013, 178, 113–118 CrossRef CAS
.
- E. Mazzotta, C. Malitesta and E. Margapoti, Anal. Bioanal. Chem., 2013, 405, 3587–3592 CrossRef CAS
.
Footnote |
† Electronic supplementary information (ESI) available. See DOI: 10.1039/c8nj03721c |
|
This journal is © The Royal Society of Chemistry and the Centre National de la Recherche Scientifique 2019 |
Click here to see how this site uses Cookies. View our privacy policy here.