DOI:
10.1039/C7RA12165B
(Paper)
RSC Adv., 2017,
7, 55427-55433
Two hybrid polyoxometalates constructed from Preyssler P5W30 clusters and Schiff base exhibiting interesting third-order NLO properties†
Received
6th November 2017
, Accepted 23rd November 2017
First published on 7th December 2017
Abstract
Two Preyssler P5W30-based compounds: [Mn(H2O)2(DAPSC)]2{[Na3(H2O)2Mn0.5(H2O)4][Mn(H2O)(DAPSC)]2[H3P5W30O110]}·7.5H2O (1) and [Co(H2O)2(DAPSC)]{[Co(H2O)2(DAPSC)][Na1.5(H2O)2]}{[Na0.5(H2O)2Co0.5(H2O)4][Co(H2O)(DAPSC)]2[H4P5W30O110]}·6H2O (2) modified by Schiff base 2,6-diacetylpyridine bis(semicarbazone) (DAPSC) and transition metal (TM) ions have been successfully isolated and structurally characterized by IR spectroscopy, PXRD, and single-crystal XRD. Structural analysis reveals that compound 1 is composed of a {[Na3(H2O)2Mn0.5(H2O)4][Mn(H2O)(DAPSC)]2[H3P5W30O110]}4− architecture (Section A), two isolated [Mn(H2O)2(DAPSC)]2+ groups (Section B), and seven and a half lattice water molecules. Compounds 1–2 represent the first Preyssler-type compounds modified by Schiff base and TM ions. Additionally, the electrocatalytic and the third-order NLO properties have been investigated.
Introduction
Polyoxometalates (POMs), a peculiar subunit of discrete transition metal-oxo clusters with oxygen-rich surfaces and strong coordinating ability, are intriguing secondary building blocks for constructing various inorganic–organic hybrid materials with potential applications in catalytic reactions,1 magnetic materials,2 nonlinear optics,3 materials science4 and electrochemistry.5 Since the distinguished Keggin-type polyoxoanion [α-PMo12O40]3− was isolated,6 POM chemistry has been rapidly developed in recent decades. In the above-mentioned area, transition metal complexes (TMCs) are commonly employed to decorate well-known POMs, for instance, Keggin-, Dawson-, Anderson- and Lindquist-type.7 In the documented larger POM clusters, the well-known Preyssler [P5W30O110]15− (abbreviating P5W30) anionic clusters attracted our considerable attention, which were discovered in 1970 (ref. 8) and structurally characterized through X-ray diffraction 15 years later.9 The P5W30 polyoxoanion possesses many merits: (i) the internal cavity can accommodate distinct metal cations with appropriate scale; (ii) abundant oxygen-rich surface and high stability over a large pH range of 1–10; (iii) high negative charges can capture TM cations. However, examples of selecting P5W30 anion as precursor to design and synthesize of POMs with decorated or expanding configuration have seldom been documented.10 The headmost expanding structures built on Preyssler anions and rare earth cations were successfully prepared by Wang et al.11 Therefore, rational design and synthesis of charming Preyssler-type POMs modified by TMCs have been a hot area of research in recent years. The Wang12 and Sun13 groups reported some inorganic–organic hybrid materials based on P5W30 anions and TM cations, they show moderate electrocatalytic abilities toward the reduction of NO2− and H2O2.
Schiff bases are a significant class of organic ligands and have been studied widely. Polydentate Schiff base ligands are capable of combining various metals through imine nitrogen, hydroxyl and carbonyl groups, it will alter the electron structure and improve the catalytic performance. The documented on POMs decorated by Schiff base are relatively rare,14 while the hybrid polyoxometalate constructed P5W30 and Schiff base ligands has not been prepared. On account of the solubility and steric-hindrance effects, we selected DAPSC as conjugated organic ligand. Owing to DAPSC possesses more coordination sites and a weaker conjugated effect, it was good candidate for decorating POMs to establish inorganic–organic hybrid materials. Herein, we have successfully obtained two unprecedented Preyssler P5W30 modified by planar Schiff base, formulated as [Mn (H2O)2(DAPSC)]2{[Na3(H2O)2Mn0.5(H2O)4][Mn(H2O)(DAPSC)]2[H3P5W30O110]}·7.5H2O (1) and [Co(H2O)2(DAPSC)]{[Co(H2O)2(DA PSC)][Na1.5(H2O)2]}{[Na0.5(H2O)2Co0.5(H2O)4][Co(H2O)(DAPSC)]2[H4P5W30O110]}·6H2O (2), respectively. Additionally, the electrochemical properties and electrocatalytic activities of both compounds were investigated. The molecular 2PA cross section σ of compounds 1–2 were 888 GM and 707 GM, which manifests that the Schiff base in the modified Preyssler polyoxoanions will strengthen the third-order NLO responses.
Experimental section
Materials and physical methods
(NH4)14NaP5W30O110·31H2O was synthesized according to the literature.15 DAPSC was isolated applying a previous approach.16 All other chemical reagents and solvents were purchased commerically and used without further purification. Elemental analysis (C, H, and N) was carried out on a PerkinElmer 2400 CHN elemental analyzer. FTIR spectra were measured in the range of 400–4000 cm−1 on a FTIR-8900 IR spectrometer using KBr pellets. TG analyses were recorded on a Pekin-Elmer Pyris Diamond TG analyzer. PXRD data were measured on a Bruker D8X diffractometer with graphite monochromatized Cu Kα radiation (λ = 0.154 nm) at room temperature. Two-photon absorption (2PA) cross-sections were gained by using a Chameleon II femtosecond laser pulse and a Ti:95 sapphire system (680–1080 nm) with 80 MHz repetition rate and a 140 fs pulse duration. Electrochemical measurements were conducted on a CHI760 electrochemical workstation. A conventional three-electrode system was utilized. A Ag/AgCl (3 mol L−1, KCl) and Pt foil were used as the counter electrode and reference electrode. The working electrode was a modified glassy carbon electrode (GCE).
Preparation of [Mn(H2O)2(DAPSC)]2{[Na3(H2O)2Mn0.5(H2O)4][Mn(H2O)(DAPSC)]2[H3P5W30O110]}·7.5H2O (1)
A mixture of (NH4)14NaP5W30O110·31H2O (0.2 g, 0.024 mmol), DAPSC (0.02 g, 0.073 mmol), MnCl2·4H2O (0.099 g, 0.5 mmol) and 10 mL distilled water and stirred for 30 min at ambient temperature. The resulting suspension was transformed into 25 mL Teflon-lined stainless-steel autoclave and heated at 120 °C for 72 h. After the autoclave cooled over 12 h to room temperature, the orange strip crystals were isolated and washed with distilled water in 40% yield (based on Mn). Anal. calcd. (Found) for C44H102Mn4.5N28Na3O137.5 P5W30: C, 5.74 (5.85); H, 1.12 (1.24); N, 4.26 (4.37)%. IR (KBr) of compound 1 (cm−1): 3416(w), 1663(s), 1163(s), 1085(m), 934(w), 910(m), 796(s), 755(w).
Preparation of [Co(H2O)2(DAPSC)]{[Co(H2O)2(DAPSC)][Na1.5(H2O)2]}{[Na0.5(H2O)2Co0.5(H2O)4][Co(H2O)(DAPSC)]2[H4P5W30O110]}·6H2O (2)
A mixture of (NH4)14NaP5W30O110·31H2O (0.2 g, 0.024 mmol), DAPSC (0.02 g, 0.073 mmol) and CoCl2·6H2O (0.1189 g, 0.5 mmol) was dissolved in sodium acetate buffer (10 mL, 0.5 mol L−1, pH = 4.0) and stirred for 100 min at room temperature. The resulting suspension was sealed in 25 mL Teflon-lined stainless-steel autoclave and kept at 120 °C for 72 h. After being cooled to room temperature, the red block crystals were obtained and washed with distilled water in 45% yield (based on Co). Anal. calcd. (Found) for C44H104Co4.5N28Na2 O138P5W30: C, 5.74 (5.89); H, 1.14 (1.35); N, 4.26 (4.35)%. IR (KBr) of compound 2 (cm−1): 3422(w), 1665(s), 1161(s), 1075(m), 934(w), 915(m), 792(s), 751(w).
X-ray crystallography
X-ray analyses data for 1–2 were performed on a Bruker Apex II CCD diffractometer at 296 K, with graphite monochromatized Mo-Kα radiation (λ = 0.71073 Å). Structures was solved by direct methods and refined by full-matrix least-squares using SHELXL-2014 crystallographic software package. The non-hydrogen atoms were refined anisotropically. CCDC 1562173 and 1562174.† All the crystallographic information for 1–2 are listed in Table 1. Selected bond lengths and angles for 1–2 are listed in Tables S2–S5.†
Table 1 Crystal data for compounds 1–2
R1 = Σ||Fo| − |Fc||/Σ|Fo|. wR2 = Σ[w(Fo2 − Fc2)2]/Σ[w(Fo2)2]1/2. |
Compounds |
1 |
2 |
Formula |
C44H102Mn4.5N28Na3O137.5P5W30 |
C44H104Co4.5N28Na2O138P5W30 |
Fw |
9210.08 |
9215.06 |
T (K) |
296(2) |
296(2) |
Wavelength (Å) |
0.71073 |
0.71073 |
Crystal system |
Triclinic |
Triclinic |
Space group |
P![[1 with combining macron]](https://www.rsc.org/images/entities/char_0031_0304.gif) |
P![[1 with combining macron]](https://www.rsc.org/images/entities/char_0031_0304.gif) |
a (Å) |
18.523(4) |
18.672(6) |
b (Å) |
19.013(4) |
19.068(6) |
c (Å) |
28.923(6) |
28.995(9) |
α (°) |
80.663(3) |
81.025(4) |
β (°) |
79.162(3) |
78.083(4) |
γ (°) |
74.784(3) |
73.649(4) |
V (Å3) |
9585(3) |
9639(5) |
Z |
2 |
2 |
Dc (Mg m−3) |
3.191 |
3.173 |
μ/mm−1 |
18.352 |
18.339 |
F(000) |
8205 |
8203 |
θ range (°) |
0.722–25.025 |
0.722–25.025 |
Limiting indices |
−21 ≤ h ≤ 22 |
−22 ≤ h ≤ 22 |
−22 ≤ k ≤ 21 |
−22 ≤ k ≤ 22 |
−33 ≤ l ≤ 34 |
−34 ≤ l ≤ 33 |
Reflections collected/unique/Rint |
68 738/33 477/0.0975 |
68 277/33 529/0.0586 |
Data/restraints/parameters |
33 477/516/2301 |
33 529/1100/2320 |
GOF |
1.035 |
1.077 |
R1a, wR2b [I > 2σ(I)] |
0.0623, 0.1593 |
0.0691, 0.1809 |
R1, wR2 (all data) |
0.0974, 0.1798 |
0.0963, 0.1946 |
Results and discussion
Synthesis
Over the past few years, hydrothermal method has been proved to be a cogent approach in preparation of metal and Schiff base decorated Preyssler P5W30 polyoxoanion. During a conventional hydrothermal method, numerous elements can impact on the nucleation and crystal development of final products, for example, initial reactant, reactant concentration, solvents, reaction time, pH values, and reaction temperature. It is a remarkable fact that the selection appropriate precursors and solvent is vital to the success of this kind of reactions. Namely, the chosen solvent as well as organic and inorganic subunits should be favored to each other. Thus we use buffer solution, which offers a moderate pH environment for TM, Schiff base and POMs. The experimental PXRD patterns of the bulk products of compounds 1–2 are consistent with the simulated ones calculated from X-ray single-crystal diffraction (Fig. S1 and S2†), which indicates the phase purity of the two compounds. The intensity difference between experimental and simulation PXRD patterns may be ascribed to the variation in the preferred orientation of the powder sample during measurements. The bond valence calculations17 suggest that the valence of Mn and Co atoms in compounds 1 and 2 are both in +2.
Description of the structures
Crystal structure of 1. X-ray single-crystal structural analysis shows that complex 1 crystallizes in triclinic P
space group. As shown in Fig. 1, its asymmetric structural unit contains a {[Na3(H2O)2Mn0.5(H2O)4][Mn(H2O)(DAPSC)]2[H3P5W30O110]}4− architecture (Section A), two isolated [Mn(H2O)2(DAPSC)]2+ TMC cations (Section B), seven and a half lattice water molecules. The Preyssler-type P5W30 polyoxoanion comprises five PW6 units, 30 μ1-O and 60 μ2-O atoms spread on the shell. The P5W30 anion owns a perfect 5-fold symmetry axis. In a mirror plane includes five P atoms perpendicular to this axis. All of 30 W atoms in the P5W30 shell are located on four parallel planes perpendicular to the center axis: each of the external and internal planes includes 5 and 10 W atoms (Fig. S11†). All W centers are connected with 6 O atoms, exhibiting a WO6 octahedron geometry.9 Two [Mn(H2O)2(DAPSC)]2+ fragments are bonded to the Preyssler P5W30 anion by Mn–O bonds to form an interesting framework in Section A. Both Mn1 and Mn3 are heptacoordinated and display distorted pentagonal bipyramid geometry {MnN3O4} coordination modes (Fig. S9†). The coordination geometries of Mn1 and Mn3 are defined by three N atoms and two oxygen atoms from a DAPSC ligand. The other two oxygen atoms, one from coordinated water molecule and the other from the Preyssler P5W30 anion, respectively. It is noteworthy that all nonhydrogen atoms of each [Mn(H2O)2(DAPSC)]2+ unit are almost planar in Section A.
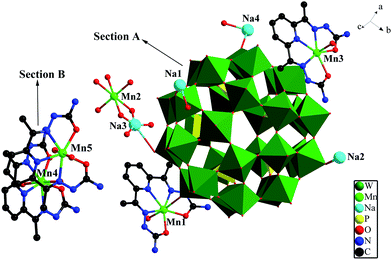 |
| Fig. 1 The asymmetric unit of compound 1. Color codes: PO4, yellow tetrahedra WO6, green octahedra; W, green; Mn, bright green; Na, turquoise; P, pink; O, red; N, bule; C, black. (All the hydrogen atoms and free water molecules have been omitted for clarity). | |
Compared with other polyoxometalates modified by Schiff bases, this is the first Preyssler P5W30 polyoxoanion decorated by Schiff base ligand. In Section A, the occupancies of Na2 and Na4 are both 75%, Na3 at half occupancy. The polyoxoanion connect to another P5W30 cluster by means of Mn2, Na3 and O atoms, which forms a amusing dimer configuration (Fig. 2). Additionally, the two free [Mn(H2O)2(DAPSC)]2+ coordination cations (Section B) are regarded as counter ions to sustain balance of charge. Mn4 and Mn5 are in hepta-coordinated MnN3O4 pentagonal bipyramid environment, the axial two oxygen atoms are both from two coordinated water molecules. The Mn–N bond and the Mn–O bond lengths are in the range of 2.25(2)–2.33(2) and 2.129(16)–2.27(2) Å. The aforementioned values are in agreement with those observed in other known Mn-contained complexes.18
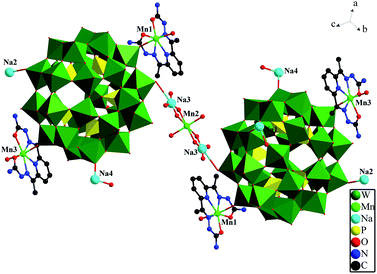 |
| Fig. 2 The dimer of compound 1. | |
It is notable that compound 1 possesses hydrogen bonds between the neighboring clusters, which extremely reinforces the whole structural stability. By means of hydrogen bonds and electrostatic interaction, the adjacent Preyssler-type {P5W30} polyoxoanion and the [Mn(H2O)(DAPSC)]2+ cations alternately connect with each other to give a 3D supramolecular network (Fig. 3).
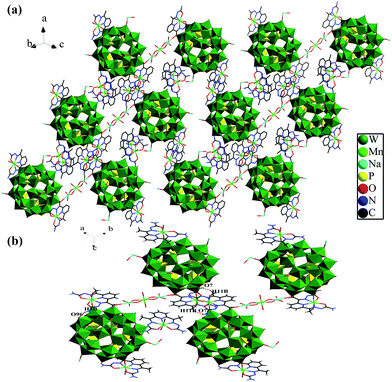 |
| Fig. 3 (a) Polyhedral and stick representation of 3D supramolecular framework of compound 1, (b) the hydrogen-bonding interactions in compound 1. | |
Crystal structure of 2. The crystallographic analysis reveals that compounds 1 and 2 are isomorphic. Their structure, bond lengths and angles, and water molecules are slightly distinct. The basic structural unit contain a {[Na0.5(H2O)2Co0.5(H2O)4][Co(H2O)(DAPSC)]2[H4P5W30O110]}5.5− structure (Section A), two isolated coordination cations: [Co(H2O)2(DAPSC)]2+ (Section B) and {[Co(H2O)2(DAPSC)][Na1.5(H2O)2]}3.5+ (Section C), and six lattice water molecules (Fig. 4). In Section C, Na1 atom has a tetracoordinated geometry, which is defined by two water molecules and two oxygen atoms from one DAPSC ligand. Na2 and Na3 atoms are at half occupancy. The coordination mode of Co ions are shown in Fig. S10.† The Co–N bond and the Co–O bond lengths are in the range of 2.16(2)–2.23(3) and 2.114(16)–2.40(5) Å. The aforementioned values are in accordance with those observed for known Co-contained complexes.19 Co4 ion is hexa-coordinated with a distorted octahedral coordination geometry defined by six oxygen atoms from four water molecules (the occupancies of O27W and O30W are both 50%). The coordination modes of Co1, Co3 and Co4, Co5 are similar to Mn1 and Mn4. Sun groups reported two hybrid M–O cluster (1α and 1β),20 the Co ions are six-coordinated and display octahedral geometry {CoN2O4} coordination modes. Each P5W30 unit is linked to adjacent clusters through double O–Co–O bridges in 1α and a single O–Co–O bridge in 1β to form 2D and 3D frameworks. Comparing with above-mentioned two complexes, compounds 1–2 are greatly different. The Co ions (except for Co4) are in seven-coordinated CoN3O4 pentagonal bipyramid environment. The P5W30 anion is linked to one neighbor through a single O–Na–O–Co–O–Na–O bridge form a amusing dimer (Fig. 5). Similar to compound 1, compound 2 exhibits 3D supramolecular framework by strong hydrogen-bonding interactions (Fig. S12†).
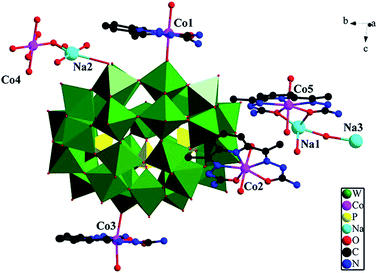 |
| Fig. 4 The asymmetric unit of compound 2. Color codes: PO4, yellow tetrahedra WO6, green octahedra; W, green; Co, pink; P, yellow; Na, turquoise; O, red; C, black; N, bule. (All the hydrogen atoms and crystallization water molecules have been omitted for clarity). | |
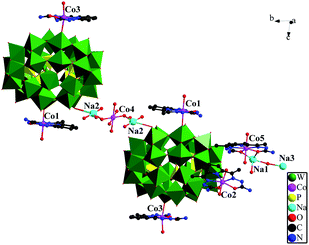 |
| Fig. 5 The dimer of compound 2. | |
IR spectra
The IR spectra of 1–2 were recorded varies from 400 to 4000 cm−1 with KBr pellets (Fig. S3 and S4†). In IR spectra, characteristic vibration patterns of the P5W30 anions, namely, ν(P–Oa), ν(W–Oterminal), ν(W–Ob/c), (Ob/c: corner and edge-shared oxygen atoms) appear at 1163 and 1085, 934 and 916, and 796 and 755 cm−1 for 1; 1161 and 1075, 934 and 915, and 792 and 751 cm−1 for 2, respectively.
Thermogravimetric (TG) analysis
In the cause of examine the thermostabilities of compounds 1–2, thermogravimetric analyses were investigated in N2 atmosphere. The TG curves of both compounds show some similarities in the range of 25–800 °C (Fig. S5 and S6†). Both compounds illustrate a consecutive weight-loss process, and the observed entire weight losses are 16.68% and 16.18% for compounds 1 and 2, respectively, which correspond to the loss of all water molecules and the decomposition of the DAPSC ligands.
Voltammetric behavior of 1 and 2-GCEs
A naphthol-modified glassy carbon electrode (GCE) was prepared as working electrode to study the electrochemical behaviors of compounds 1–2 in 1 mol L−1 sulfuric acid aqueous solution at different scanning speed. As depicted in Fig. 6a and b, from 0 to −800 mV, three pairs of reversible redox peaks related to redox reactions are explored for 1 and 2-GCE. The average peak potentials E1/2 = 0.5(Epa + Epc) of I/I′, II/II′, and III/III′ are −0.300, −0.496, and −0.687 V for 1-GCE as well as −0.314, −0.504, and −0.658 V for 2-GCE. Two pairs of reversible redox peaks I/I′ and II/II′ correspond to two successive double electron processes of the modified P5W30 polyoxoanion.10b,13,21 The cyclic voltammetry behavior of 1 and 2-GCEs were recorded at changing scan rates. The cathodic peak potentials move to the minus orientation and the homologous anodic peak potentials move toward the positive orientation with enhancing scanning rates, which indicates that the redox process of 1 and 2-GCEs are surface-controlled.22
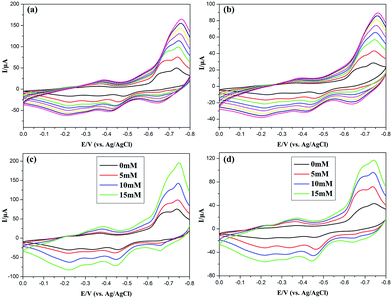 |
| Fig. 6 (a, b) Cyclic voltammograms of the 1-, and 2-GCEs in 1 mol L−1 sulfuric acid aqueous solution at distinct scanning speeds (from inside to outside: 50, 100, 150, 200, 250, 300, 350, 400 mV s−1). (c, d) Cyclic voltammograms of the 1-, and 2-GCEs in 1 mol L−1 sulfuric acid aqueous solution containing H2O2 in different concentration with a scanning speed of 100 mV s−1. | |
Electrocatalytic properties of 1 and 2-GCEs for the reduction of H2O2
POMs have been widely employed as electrocatalysts for the reduction of H2O2. Fig. 6c and d reveal 1 and 2-GCEs show good electrocatalytic activities on the reduction of H2O2 in 1 M H2SO4 aqueous solution with the potential changing from 0 mV to −800 mV. With the increase of the concentration of H2O2 from 0.0 to 15.0 mmol L−1, the reduction peak currents increase clearly while the corresponding oxidation peak currents remarkably reduced, which indicates that the 1 and 2-GCEs have good electrocatalytic ability for the reduction of H2O2.
Nonlinear optical properties
DAPSC is an outstanding conjugated polydentate ligand, and the incorporation of POMs with Schiff base may be able to enhance the NLO response. Herein, we explored the electronic spectra of both compounds in DMF solution with concentration of 1.0 × 10−4 mol L−1 at ambient temperature. The 2PA coefficient β and 2PA cross section of σ were calculated by the open-aperture Z-scan method with a femtosecond laser pulse and Ti:95 sapphire system.23 Fig. 7 and 8 reveal the open-aperture Z-scan data of 1–2. The red empty circles are the test data and the black solid line on behalf of the theoretical fitting line modified by the equations:24 |
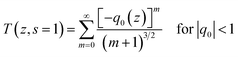 | (1) |
|
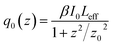 | (2) |
here, z0 = πω02/λ is the diffraction distance of the beam, In which ω0 is the spot size at focus, λ is wave length of the beam, z is sample position. I0 is the input intensity at the focus z = 0, Leff = (1 − e−αL)/α is the effective length, where α is the linear absorption coefficient, L is the sample length. By using the above equations, we inference that the 2PA absorption coefficient β is calculated as 0.002099 cm GW−1 and 0.001715 cm GW−1 for 1–2, respectively. Furthermore, the molecular 2PA cross-section σ could be determined by the subjacent formula:where h, ν, NA, and d are the Planck constant, frequency of input intensity, the Avogadro's constant, and is the concentration of the compound, respectively. According to formula (3), the molecular 2PA cross-section σ of both compounds were computed as 888 GM and 707 GM (1 GM = 10−50 cm4 per photon). It is well known that the modified π-electron delocalization in the framework leaded to the third-order nonlinear optics response. But so far the investigation of POMs in the area of nonlinear optical materials basically concentrate on the well-known Keggin- and Dawson-type polyoxoanions.25 As far as we know, two compounds have bigger molecular TPA cross section σ comparing with other reported NLO materials.26 The third-order NLO properties of two compounds manifest that POMs modified by Schiff base lead to a powerful NLO response, and could be promising candidates for making NLO materials.
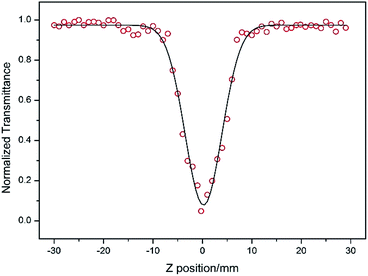 |
| Fig. 7 Z-scan data for compound 1 in DMF at 1.0 × 10−4 mol L−1 measured by open aperture Z-scan method. | |
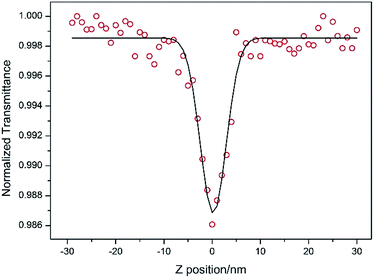 |
| Fig. 8 Z-scan data for compound 2 in DMF at 1.0 × 10−4 mol L−1 measured by open aperture Z-scan method. | |
Conclusions
In conclusion, two novel based on Preyssler P5W30 compounds modified by Schiff base and TM ions were synthesized under hydrothermal conditions. The cyclic voltammograms of both compounds have been studied. Both compounds show better electrocatalytic activities toward the reduction of H2O2. Moreover, the both compounds have moderate molecular 2PA cross section σ, hence they own potential applications in NLO materials. The successful isolation of compounds 1–2 can provide new synthetic strategy for the construct of distinct POMs-based inorganic–organic hybrid materials by means of combining diverse flexible organic molecules. Further work in this area will be focused on preparing Preyssler-based compounds with fascinating structure and rich physicochemical properties.
Conflicts of interest
There are no conflicts to declare.
Acknowledgements
This work was supported by the NSFC (Grant. 21571103), Qing Lan project and the Major Natural Science Projects of the Jiangsu Higher Education Institution (Grant. 16KJA150005).
Notes and references
-
(a) S. S. Wang and G. Y. Yang, Chem. Rev., 2015, 115, 4893 CrossRef CAS PubMed;
(b) H. Lv, Y. V. Geletii, C. Zhao, J. W. Vickers, G. Zhu, Z. Luo, J. Song, T. Lian, D. G. Musaev and C. L. Hill, Chem. Soc. Rev., 2012, 41, 7572 RSC;
(c) D. L. Long, R. Tsunashima and L. Cronin, Angew. Chem., Int. Ed., 2010, 49, 1736 CrossRef CAS PubMed;
(d) C.-Y. Sun, S.-X. Liu, D.-D. Liang, K.-Z. Shao, Y.-H. Ren and Z.-M. Su, J. Am. Chem. Soc., 2009, 131, 1883 CrossRef CAS PubMed;
(e) W. B. Kim, T. Voitl, G. J. Rodriguez-Rivera and J. A. Dumesic, Science, 2004, 305, 1280 CrossRef CAS PubMed.
-
(a) M. A. AlDamen, J. M. Clemente-Juan, E. Coronado, C. Martí-Gastaldo and A. Gaita-Ariño, J. Am. Chem. Soc., 2008, 130, 8874 CrossRef CAS PubMed;
(b) U. Kortz, A. Müller, J. van Slageren, J. Schnack, N. S. Dalal and M. Dressel, Coord. Chem. Rev., 2009, 253, 2315 CrossRef CAS;
(c) J. M. Clemente-Juan, E. Coronado and A. Gaita-Arino, Chem. Soc. Rev., 2012, 41, 7464 RSC;
(d) S. T. Zheng and G. Y. Yang, Chem. Soc. Rev., 2012, 41, 7623 RSC.
-
(a) C.-G. Liu, W. Guan, L.-K. Yan, Z.-M. Su, P. Song and E.-B. Wang, J. Phys. Chem. C, 2009, 113, 19672 CrossRef CAS;
(b) H. Miao, H.-X. Wan, M. Liu, Y. Zhang, X. Xu, W.-W. Ju, D.-r. Zhu and Y. Xu, J. Mater. Chem. C, 2014, 2, 6554 RSC;
(c) Y. Dong, X. Xu, G. Zhou, H. Miao, G. Hu and Y. Xu, Dalton Trans., 2015, 44, 18347 RSC;
(d) H. Miao, Y. Dong, Z. Chen, X. He, G. Hu and Y. Xu, Dalton Trans., 2016, 45, 12717 RSC.
-
(a) H. Y. An, E. B. Wang, D. R. Xiao, Y. G. Li, Z. M. Su and L. Xu, Angew. Chem., Int. Ed., 2006, 45, 904 CrossRef CAS PubMed;
(b) D. L. Long, E. Burkholder and L. Cronin, Chem. Soc. Rev., 2007, 36, 105 RSC;
(c) M. S. Wang, G. Xu, Z. J. Zhang and G. C. Guo, Chem. Commun., 2010, 46, 361 RSC;
(d) H. B. Wu, B. Y. Xia, L. Yu, X. Y. Yu and X. W. Lou, Nat. Commun., 2015, 6, 6512 CrossRef CAS PubMed.
-
(a) B. Keita, I. M. Mbomekalle, Y. W. Lu, L. Nadjo, P. Berthet, T. M. Anderson and C. L. Hill, Eur. J. Inorg. Chem., 2004, 2004, 3462 CrossRef;
(b) J. Zhang, A. M. Bond, D. R. MacFarlane, S. A. Forsyth, J. M. Pringle, A. W. A. Mariotti, A. F. Glowinski and A. G. Wedd, Inorg. Chem., 2005, 44, 5123 CrossRef CAS PubMed.
- J. Berzelius, Ann. Phys., 1826, 6, 369 CrossRef.
-
(a) M. Yuan, Y. Li, E. Wang, C. Tian, L. Wang, C. Hu, N. Hu and H. Jia, Inorg. Chem., 2003, 42, 3670 CrossRef CAS PubMed;
(b) Y. Q. Lan, S. L. Li, K. Z. Shao, X. L. Wang and Z. M. Su, Dalton Trans., 2008, 3824 RSC;
(c) Y. Kikukawa, K. Yamaguchi and N. Mizuno, Angew. Chem., Int. Ed., 2010, 49, 6096 CrossRef CAS PubMed;
(d) H. Yang, S. Gao, J. Lu, B. Xu, J. Lin and R. Cao, Inorg. Chem., 2010, 49, 736 CrossRef CAS PubMed;
(e) L. Wang, P. Yin, J. Zhang, J. Hao, C. Lv, F. Xiao and Y. Wei, Chem.–Eur. J., 2011, 17, 4796 CrossRef CAS PubMed.
- C. Preyssler, Bull. Soc. Chim. Fr., 1970, 1, 30 CAS.
- M. H. Alizadeh, S. P. Harmalker, Y. Jeannin, J. Martin-Frere and M. T. Pope, J. Am. Chem. Soc., 1985, 107, 2662 CrossRef CAS.
-
(a) F. Hussain, U. Kortz, B. Keita, L. Nadjo and M. T. Pope, Inorg. Chem., 2006, 45, 761 CrossRef CAS PubMed;
(b) C.-Y. Yang, L.-C. Zhang, Z.-J. Wang, L. Wang, X.-H. Li and Z.-M. Zhu, J. Solid State Chem., 2012, 194, 270 CrossRef CAS;
(c) T. P. Hu, Y. Q. Zhao, Z. Jaglicic, K. Yu, X. P. Wang and D. Sun, Inorg. Chem., 2015, 54, 7415 CrossRef CAS PubMed.
- Y. Lu, Y. Li, E. Wang, X. Xu and Y. Ma, Inorg. Chim. Acta, 2007, 360, 2063 CrossRef CAS.
- X. Wang, J. Li, A. Tian, H. Lin, G. Liu and H. Hu, Inorg. Chem. Commun., 2011, 14, 103 CrossRef CAS.
- Y. Q. Zhao, K. Yu, L. W. Wang, Y. Wang, X. P. Wang and D. Sun, Inorg. Chem., 2014, 53, 11046 CrossRef CAS PubMed.
-
(a) Q. Wu, Y. G. Li, Y. H. Wang, R. Clerac, Y. Lu and E. B. Wang, Chem. Commun., 2009, 5743 RSC;
(b) A. Yokoyama, T. Kojima, K. Ohkubo and S. Fukuzumi, Inorg. Chem., 2010, 49, 11190 CrossRef CAS PubMed;
(c) Y. Bai, G.-Q. Zhang, D.-B. Dang, P.-T. Ma, H. Gao and J.-Y. Niu, CrystEngComm, 2011, 13, 4181 RSC;
(d) X. Meng, C. Qin, X. L. Wang, Z. M. Su, B. Li and Q. H. Yang, Dalton Trans., 2011, 40, 9964 RSC;
(e) Q. Wu, W. L. Chen, D. Liu, C. Liang, Y. G. Li, S. W. Lin and E. B. Wang, Dalton Trans., 2011, 40, 56 RSC;
(f) C. Zou, Z. Zhang, X. Xu, Q. Gong, J. Li and C. D. Wu, J. Am. Chem. Soc., 2012, 134, 87 CrossRef CAS PubMed.
- M. H. Alizadeh, S. P. Harmalker, Y. Jeannin, J. Martin-Frere and M. T. Pope, J. Am. Chem. Soc., 1985, 107, 2662 CrossRef CAS.
- A. A. Abu-Hussen and W. Linert, Spectrochim. Acta, Part A, 2009, 74, 214 CrossRef PubMed.
-
(a) I. Brown and D. Altermatt, Acta Crystallogr., Sect.
B: Struct. Sci., 1985, 41, 244 Search PubMed;
(b) R. M. Wood and G. J. Palenik, Inorg. Chem., 1998, 37, 4149 CrossRef CAS PubMed;
(c) I. D. Brown, Chem. Rev., 2009, 109, 6858 CrossRef CAS PubMed.
-
(a) H. Liu, C. J. Gomez-Garcia, J. Peng, J. Sha, Y. Li and Y. Yan, Dalton Trans., 2008, 6211 RSC;
(b) L. Yang, Q. Liu, P. Ma, J. Niu and J. Wang, Dalton Trans., 2015, 44, 13469 RSC.
- X. B. Han, Z. M. Zhang, T. Zhang, Y. G. Li, W. Lin, W. You, Z. M. Su and E. B. Wang, J. Am. Chem. Soc., 2014, 136, 5359 CrossRef CAS PubMed.
- Y. Q. Zhao, K. Yu, L. W. Wang, Y. Wang, X. P. Wang and D. Sun, Inorg. Chem., 2014, 53, 11046 CrossRef CAS PubMed.
-
(a) M. R. Antonio and M.-H. Chiang, Inorg. Chem., 2008, 47, 8278 CrossRef CAS PubMed;
(b) Z. Zhang, S. Yao, Y. Qi, Y. Li, Y. Wang and E. Wang, Dalton Trans., 2008, 3051 RSC.
- D. Martel and A. Kuhn, Electrochim. Acta, 2000, 45, 1829 CrossRef CAS.
- M. Yin, H. Li, S. Tang and W. Ji, Appl. Phys. B: Lasers Opt., 2000, 70, 587 CrossRef CAS.
-
(a) W. Zhao and P. Palffy-Muhoray, Appl. Phys. Lett., 1994, 65, 673 CrossRef CAS;
(b) D. McLean, P. Fleitz, T. Pottenger, R. Sutherland, M. Brant and D. Brandelik, Opt. Lett., 1993, 18, 858 CrossRef CAS PubMed;
(c) M. Sheik-Bahae, A. A. Said, T.-H. Wei, D. J. Hagan and E. W. Van Stryland, IEEE J. Quantum Electron., 1990, 26, 760 CrossRef CAS.
-
(a) G. Hu, H. Miao, H. Mei, S. Zhou and Y. Xu, Dalton Trans., 2016, 45, 7947 RSC;
(b) Y. Dong, G. Hu, H. Miao, X. He, M. Fang and Y. Xu, Inorg. Chem., 2016, 55, 11621 CrossRef CAS PubMed;
(c) J. Guo, D. Zhang, L. Chen, Y. Song, D. Zhu and Y. Xu, Dalton Trans., 2013, 42, 8454 RSC;
(d) C. G. Liu, W. Guan, P. Song, Z. M. Su, C. Yao and E. B. Wang, Inorg. Chem., 2009, 48, 8115 CrossRef CAS PubMed.
-
(a) H. Miao, H. X. Wan, M. Liu, Y. Zhang, X. Xu, W. W. Ju and Y. Xu, J. Mater. Chem. C, 2014, 2, 6554 RSC;
(b) Y. Zhang, L. Huang, H. Miao, H. X. Wan, H. Mei, Y. Liu and Y. Xu, Chem.–Eur. J., 2015, 21, 3234 CrossRef CAS PubMed;
(c) Y. Dong, X. Xu, G. Zhou, H. Miao, G. Hu and Y. Xu, Dalton Trans., 2015, 44, 18347 RSC.
Footnote |
† Electronic supplementary information (ESI) available: Summary of selected bond lengths and angles; table of hydrogen bond lengths and bond angles; IR, XRD, TG and coordination modes of 1 and 2. CCDC reference numbers: 1562173 (1) and 1562174 (2). For ESI and crystallographic data in CIF or other electronic format see DOI: 10.1039/c7ra12165b |
|
This journal is © The Royal Society of Chemistry 2017 |