DOI:
10.1039/C7RA11783C
(Paper)
RSC Adv., 2017,
7, 55051-55059
Ligand steric effects on α-diimine nickel catalyzed ethylene and 1-hexene polymerization†
Received
25th October 2017
, Accepted 28th November 2017
First published on 4th December 2017
Abstract
A series of ortho-dibenzhydryl or ortho-sec-phenethyl substituted α-diimine nickel complexes with systematically varied ligand steric effects were used as precatalysts for the polymerization of ethylene and 1-hexene upon activation with Et2AlCl. The effects of ligand sterics and polymerization temperature on the catalytic activity, molecular weight and polymer microstructure were evaluated in detail. In ethylene polymerization, it is possible to tune the catalytic activities [(0.98–2.41) × 106 g (mol Ni h)−1], polymer molecular weights [Mn: (1.8–13.1) × 105 g mol−1] and branching densities (55–108/1000C) over a very wide range. The molecular weights and branching structure depended on the nickel complexes as well as the polymerization temperature, and the polymer branching densities were decreased with increasing ligand steric effects and decreasing polymerization temperature. Polymerization of 1-hexene with ortho-dibenzhydryl substituted nickel complexes resulted in branched polymers (106–140/1000C) with high molecular weights [Mn: (0.64–3.88) × 104 g mol−1] and narrow molecular weight distribution (Mw/Mn = 1.16–1.52, 40–80 °C). The increasing steric hindrance of the catalyst leads to enhanced 2,1-insertion of 1-hexene and the chain-walking reaction.
Introduction
The research on ethylene and α-olefin polymerizations catalyzed by late-transition metal catalysts has been a great highlight for the development of polyolefin materials in recent years.1,2 The nickel and palladium complexes ligated by α-diimine3,4 showed good activity for ethylene and α-olefin polymerizations to produce a variety of branched polyolefins, because the metal-alkyl species can move on the polymer backbone before the next monomer insertion, i.e., chain-walking,5 via rapid β-hydride elimination and re-insertion (Scheme 1). Chain-walking polymerization can give polymers with unique structures which cannot be obtained by common vinyl polymerization.6,7 The mechanism of chain-walking for α-olefin polymerization involves 1,2- and 2,1-insertion followed by chain-walking (Scheme 1(ii–iv)), in which the active metal center undergoes chain-walking to the terminal carbon followed by monomer insertion.8 1,2-Insertion gives the methyl branch (iii) in a ω,2-enchainment, while 2,1-insertion gives a long methylene sequence in a ω,1-enchainment (iv). If the metal fails to chain walk after 1,2-insertion, the final polymer contains n-alkyl branches (ii), which is found in common poly(α-olefin)s. The highly-branched polymers with high amounts of methyl and alkyl branches are amorphous, while the chain-straightened polymers will be semi-crystalline.6
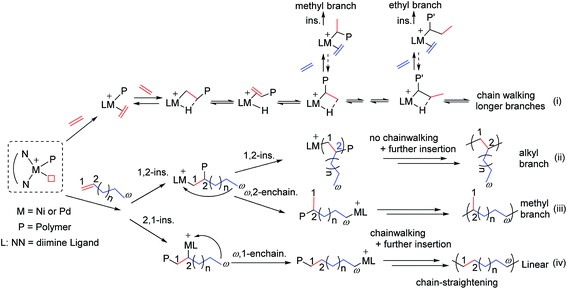 |
| Scheme 1 Chain-walking mechanism for ethylene and α-olefin polymerization catalyzed by α-diimine nickel and palladium complexes. | |
Previous studies indicated that sterically bulky ligands are usually required to afford nickel and palladium catalysts capable of generating high molecular weight polymers.2c Chain straightening polymerization of α-olefins can generate highly linear polyethylene, which is the most abundantly produced plastic owing to its inexpensive monomer, thermoplastic properties, and semi-crystalline nature.4d,8 The branching degree of the polymer can be controlled via catalyst structures (Chart 1) and polymerization conditions such as ethylene pressures or polymerization temperatures.9,10 For example, Wu et al. synthesized a camphyl-based α-diimine nickel catalyst C1 that can polymerize ethylene, propylene and α-olefins in a living fashion under the optimized conditions.11 This catalyst exhibited 1,3-enchainment fraction of 45% in propylene polymerization, and produced poly(α-olefin)s with an obvious Tm. Brookhart et al. reported a “sandwich” (8-p-tolyl naphthyl) substituted α-diimine nickel catalyst C2 that yielded highly-branched (up to 152 branches/1000C) polyethylene.12 Coates et al. reported that the living/controlled polymerization of propylene and higher α-olefins using C2-symmetric α-diimine nickel catalyst C3a bearing chiral sec-phenethyl moiety.13 Recently, the same group synthesized a dibenzobarrelene-bridged α-diimine nickel catalyst C3b bearing chiral sec-phenethyl moiety.4d The catalyst afforded high molecular weight polyethylenes with narrow dispersities and low degrees of branching, and demonstrated living behavior at room temperature and produced linear polyethylene (Tm = 135 °C) at −20 °C. Guan et al. designed a α-diimine nickel catalyst C4 bearing a macrocyclic ligand, which showed significantly higher thermal stability than the acyclic analogs, and promoted living polymerization of propylene even at 50–75 °C.14 This type of catalyst enhances both the 2,1-insertion of propylene and the chain-walking reaction. Long et al. examined α-diimine nickel catalysts C5a containing ortho-dibenzhydryl moiety for high-temperature ethylene polymerization,15 where the catalyst maintained high activities at temperatures as high as 100 °C.
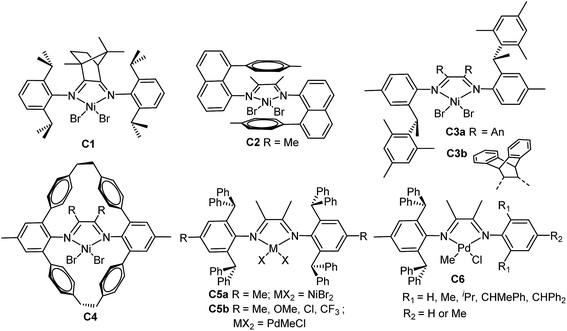 |
| Chart 1 Selected examples of α-diimine nickel and palladium catalysts. | |
Chen et al. recently reported that α-diimine palladium catalysts C5b bearing the dibenzhydryl moiety displayed high thermal stability and high activity in slow chain-walking ethylene (co)polymerization,16 producing semicrystalline polyethylene and ethylene/methyl acrylate copolymer with high molecular weight and low branching density. They also designed a series of α-diimine palladium catalysts C6 bearing both the dibenzhydryl moiety and with systematically varied ligand sterics for ethylene and 1-hexene polymerization,17 the molecular weights and branching densities could be tuned over a very wide range. In this work, we wish to report the synthesis of a series of ortho-dibenzhydryl and ortho-sec-phenethyl substituted α-diimine nickel complexes with systematically varied ligand sterics, and the investigation of the influence of ligand structure and polymerization conditions on ethylene and 1-hexene polymerizations.
Results and discussion
Synthesis and characterization of the nickel complexes
The desired ligands were prepared using the literature procedure in high yields without using column chromatography.17,18 α-Diimine nickel precatalysts used in this study are summarized in Scheme 2. Complexes 2–4 were synthesized from the reactions of the corresponding ligand with (DME)NiBr2 in high yields. Chen et al. previously reported ortho-dibenzhydryl and ortho-sec-phenethyl substituted nickel complexes 14f and 518 that exhibited high activities in ethylene polymerization and produced different branched polyethylene with high molecular weight. Complexes 1 and 5 were prepared and studied in order to obtain accurate comparisons in ethylene polymerization. This way, a series of α-diimine nickel complexes bearing ortho-dibenzhydryl and ortho-sec-phenethyl groups and with systematically varied ligand sterics could be studied in the polymerization of ethylene and 1-hexene. In addition, the methyl substituted complex 6 was also used for comparison in this study.
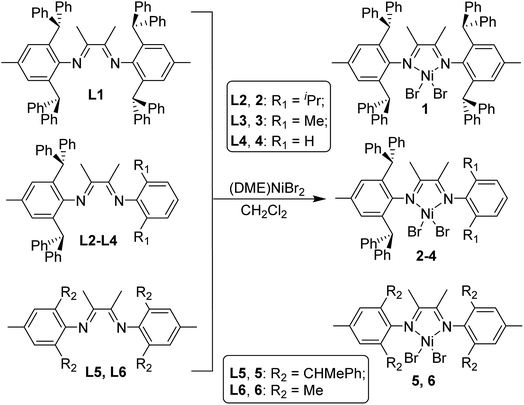 |
| Scheme 2 Synthesis of α-diimine nickel complexes 1–6. | |
X-ray crystallographic studies
Suitable crystals of complexes 2, 3 and 5 were obtained by layering n-hexane onto their dichloromethane solution at room temperature, respectively. The molecular structures of 2, 3 and 5 was confirmed by single-crystal X-ray diffraction and the corresponding ORTEP diagram with selected bond distances and angles are shown in Fig. 1, 2 and 3, respectively. Crystal data, data collection and refinement parameters are listed in Table S1 (see ESI†).
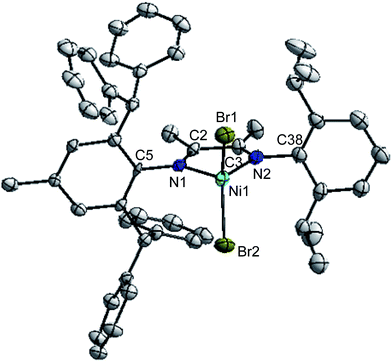 |
| Fig. 1 Molecular structure of complex 2 with thermal ellipsoids drawn at 30% probability, and H atoms have been omitted for clarity. Selected bond lengths (Å) and angles (°): Ni1–N1 = 2.018(13), Ni1–N = 2.026(13), Ni1–Br1 = 2.331(3), Ni1–Br2 = 2.334(3), N1–C2 = 1.27(2), N1–C5 = 1.43(2); N1–Ni1–N2 = 80.4(5), N1–Ni1–Br1 = 114.3(4), N2–Ni1–Br1, 110.0(5), N1–Ni1–Br2 = 111.8(4), N2–Ni1–Br2 = 118.2(4), Br1–Ni1–Br2 = 116.90(13), C2–N1–Ni1 = 111.6(11), C5–N1–Ni1 = 128.7(10). | |
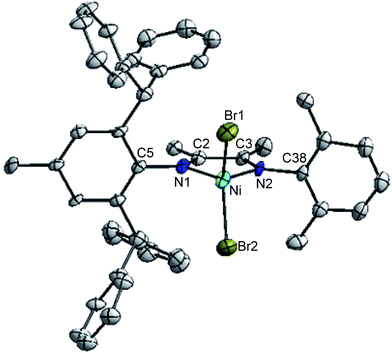 |
| Fig. 2 Molecular structure of complex 3 with thermal ellipsoids drawn at 30% probability, and H atoms have been omitted for clarity. Selected bond lengths (Å) and angles (°): Ni1–N1 = 2.018(13), Ni1–N2 = 2.026(13), Ni1–Br1 = 2.331(3), Ni1–Br2 = 2.334(3), N1–C2 = 1.27(2), N1–C5 = 1.43(2), N2–C3 = 1.17(2), N2–C38 = 1.48(2), C2–C3 = 1.53(2); N1–Ni1–N2 = 80.4(5), N1–Ni1–Br1 = 114.3(4), N2–Ni1–Br1 = 110.0(5), N1–Ni1–Br2 = 111.8(4), N2–Ni1–Br2 = 118.2(4), Br1–Ni1–Br2 = 116.90(13), C2–N1–C5 = 119.4(14), C2–N1–Ni1 = 111.6(11), C5–N1–Ni1 = 128.7(10), C3–N2–C38 = 121.4(16), C3–N2–Ni1 = 115.4(13), C38–N2–Ni1 = 123.2(11). | |
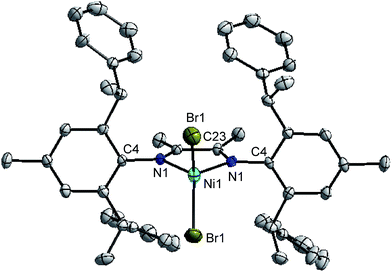 |
| Fig. 3 Molecular structure of complex 5 with thermal ellipsoids drawn at 30% probability, and H atoms have been omitted for clarity. Selected bond lengths (Å) and angles (°): Br1–Ni1 = 2.3308(12), Br1i–Ni1 = 2.3309(12), Ni1–N1 = 2.034(5), N1–C4 = 1.421(7), N1–C23 = 1.297(8), C23–C23i = 1.514(11); Br1–Ni1–Br1i = 117.30(9), N1i–Ni1–N1 = 81.2(3), N1i–Ni1–Br1 = 112.14(14), N1–Ni1–Br1 = 114.40(14), N1–Ni1–Br1i = 112.14(14), N1i–Ni1–Br1i, 114.40(14), C4–N1–Ni1, 124.5(4). | |
Both unsymmetrical α-diimine nickel complexes 2 (Fig. 1) and 3 (Fig. 2) bearing ortho-dibenzhydryl moiety exhibited similar geometries, and a distorted tetrahedron geometry was observed for the Ni center. However, complex 5 (Fig. 3) bearing ortho-sec-phenethyl groups exhibited symmetrical chiral pocket about the Ni center, and exhibited near C2-symmetry. In the solid state, the most interesting feature of ligand is the conformation of the substituents attached to N1 and N2. These groups are rotated about 180° from the position they must occupy to chelate metal Ni. The rotation has been confirmed by the crystal structure of its complex. The X-ray structures of ligand18 and complex exhibit trans and cis conformation about the central C–C bond of the backbone, respectively. Both aryl rings bonded to the imine nitrogen of the α-diimine lie nearly perpendicular to the plane formed by the nickel and coordinated nitrogen atoms. The bond angles and bond distances are consistent with those previously reported for α-diimine nickel complexes.4 In fact, the Ni1–N1 bond distances in complex 2 (2.026 Å) and 3 (2.018 Å) are similar to these in 5 (2.034 Å), as well as the Ni1–Br1 bond distances (2.331 Å for 2; 2.331 Å for 3; 2.3308 Å for 5). In addition, the Br1–Ni1–Br2 angles are 116.90° for complex 2 and 116.90° for complex 3 are similar to these in complex 5 (117.30°).
Ethylene polymerization studies
All the dibromo nickel complexes 1–6 were applied to ethylene polymerization activated by Et2AlCl at the [Al]/[Ni] molar ratio of 600 for 30 min under 9 atm of ethylene (Table 1). First, the influence of the polymerization temperature was studied by varying the temperature from 20 to 80 °C. The catalytic activities of complex 1 were increased with polymerization temperatures and the highest activity was observed at 80 °C (entry 3, Table 1). The maximum catalytic activities for complexes 2–5 were observed at 50 °C (entries 5, 8, 11 and 14, Table 1), and the polymerization at 80 °C still gave high catalytic activities on the level of 106 g of PE per (mol Ni h) (entries 6, 9, 12 and 15, Table 1). Therefore, these α-diimine nickel complexes 1–5 were highly active in ethylene polymerization, and showed much higher thermal stability than the corresponding methyl substituted complex 6.
Table 1 Effect of catalyst and temperature on ethylene polymerizationa
Entry |
Precat. |
Temp (°C) |
Yield (g) |
Activityb |
Mnc |
Mw/Mnc |
Bd |
Polymerization conditions: Ni = 2.4 μmol in CH2Cl2 (2 mL); cocatalyst Et2AlCl, Al/Ni = 600; toluene = 20 mL; 9 atm of ethylene; time = 30 min. 106 g of PE (mol of Ni)−1 h−1. Mn and Mw/Mn determined by GPC, 105 g mol−1. Branching numbers per 1000C were determined by 1H NMR. |
1 |
1 |
20 |
1.18 |
0.98 |
9.9 |
1.80 |
55 |
2 |
1 |
50 |
1.69 |
1.41 |
13.1 |
1.99 |
58 |
3 |
1 |
80 |
1.89 |
1.58 |
8.0 |
1.69 |
64 |
4 |
2 |
20 |
2.21 |
1.84 |
7.2 |
1.62 |
81 |
5 |
2 |
50 |
2.89 |
2.41 |
8.1 |
1.50 |
84 |
6 |
2 |
80 |
2.59 |
2.16 |
5.2 |
2.13 |
90 |
7 |
3 |
20 |
1.75 |
1.46 |
4.0 |
2.09 |
86 |
8 |
3 |
50 |
2.85 |
2.38 |
6.5 |
2.06 |
91 |
9 |
3 |
80 |
2.09 |
1.74 |
2.9 |
2.19 |
98 |
10 |
4 |
20 |
2.11 |
1.76 |
2.2 |
1.96 |
95 |
11 |
4 |
50 |
2.76 |
2.30 |
2.9 |
2.32 |
101 |
12 |
4 |
80 |
1.78 |
1.48 |
1.8 |
2.50 |
108 |
13 |
5 |
20 |
2.13 |
1.78 |
7.3 |
1.52 |
87 |
14 |
5 |
50 |
2.65 |
2.21 |
8.7 |
1.80 |
93 |
15 |
5 |
80 |
1.84 |
1.53 |
5.4 |
2.09 |
103 |
16 |
6 |
20 |
0.76 |
0.63 |
0.8 |
1.67 |
90 |
17 |
6 |
50 |
0.53 |
0.44 |
0.6 |
1.98 |
95 |
18 |
6 |
80 |
Trace |
— |
— |
— |
— |
On the other hand, the polymer molecular weight was strongly depended on the polymerization temperature with narrow molecular weight distributions of Mw/Mn ≤ 2.50. The maximum molecular weight was observed at 50 °C (entries 2, 5, 8, 11 and 14, Table 1). Further increase of temperature caused a decrease in the molecular weight accompanied by broadening of the molecular weight distribution (entries 3, 6, 9, 12 and 15, Table 1). This suggests that fast chain transfer takes place at higher temperatures.1a
Then, the effect of catalyst precursors 1–6 were investigated with [Al]/[Ni] ratio of 600 (entries 1–18, Table 1). Complexes 1–5 produced polymers in high yields with high molecular weights (Mn ≥ 1.8 × 105 g mol−1) and narrow molecular weight distributions (Mw/Mn = 1.50–2.50). Steric effect of ortho-position in the anilinic moiety can be evaluated by comparing 1 to 4 and 5, 6. At 50 °C and 9 atm of ethylene pressure, the polymerization activity decreased in the following order, 2 ≥ 3 ≥ 4 ≥ 5 ≥ 1 > 6 (entries 2, 5, 8, 11, 14 and 17, Table 1).
Complex 2 with isopropyl groups showed the highest activity among these complexes, but gave the lower molecular weight than that of complex 1. At 50 °C, the molecular weight of the polymers decreased in the following order, 1 ≥ 5 ≥ 2 ≥ 3 ≥ 4 ≥ 6. These results indicated that the rate of chain propagation was greatly promoted by the bulky ortho-dibenzhydryl and ortho-sec-phenethyl substituents of the ligand's aryl rings. The highest molecular weight of the polymer obtained by complex 1 suggests that the bulky ortho-dibenzhydryl could efficiently suppress chain-transfer reactions.
The branching densities of the obtained polyethylenes were determined by 1H NMR spectroscopy,19 and the results are shown in Table 1. The branching densities were increased with polymerization temperature from 20 to 80 °C (1, 55–64/1000C; 2, 81–90/1000C; 3, 86–98/1000C; 4, 95–108/1000C; 5, 87–103/1000C; 6, 90–95/1000C). The branching densities were decreased by increasing the steric bulkiness of the ortho substituents on the α-diimine ligands by comparing 1 to 4. For example, at 50 °C, the number of chain branching was depended on the precatalysts and decreased in the following order, 4 [2,6-di(H), 101/1000C] > 3 [2,6-di(Me), 91/1000C] > 2 [2,6-di(iPr), 84/1000C] > 1 [2,6-di(CHPh2), 58/1000C] (entries 2, 5, 8 and 11, Table 1). These results indicated that the introduction of dibenzhydryl substituent into the ortho-position of the imines ligands can decrease the branching degree of the polyethylenes.15,16 In addition, complex 5 [2,6-di(CHMePh), 50 °C, 93/1000C] led to slightly lower branching density than complex 6 [2,6-di(Me), 50 °C, 95/1000C].
The branching structures analysis based on 13C NMR showed that 74 methyl, 7 ethyl, 5n-propyl, 4n-butyl, 1-sec-butyl (branch on branch structure) and 17 longer chains (>C4 branches) exist for the polyethylene produced by complex 4 at 80 °C (Fig. 4(i), entry 12, Table 1). In contrast, only 55 methyl branches were observed for complex 1 at 20 °C (Fig. 4(ii), entry 1, Table 1). The microstructure difference and the lower degree of branching density for the sterically bulkier catalysts are probably due to the relatively greater preference of insertion of ethylene into a primary metal alkyl species versus insertion into a secondary metal-alkyl species.16 The rationale for the formation of the major types of branches (methyl, ethyl, propyl, butyl and longer chains) in the polyethylenes obtained in this work is shown in Scheme 1(i).
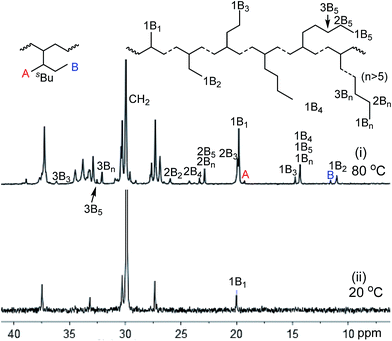 |
| Fig. 4 13C NMR spectra of polyethylenes obtained by complex 1 at 20 °C (ii) and complex 4 at 80 °C (i) (A and B refer to methyl carbon of sec-butyl branches, entries 1 and 12, Table 1). | |
1-Hexene polymerization studies
Chen et al. recently reported the tuning of polyethylene and poly(1-hexene) microstructures using a series of α-diimine palladium complexes C6 bearing both the dibenzhydryl moiety and with systematically varied ligand sterics.17 In the above-mentioned ethylene polymerization, a series of α-diimine dibenzhydryl substituted nickel complexes 1–4 with systematically varied ligand sterics showed high activities and high thermal stability, and produced polyethylene with high molecular weight. The tuning in ligand sterics enables the tuning of the polymer microstructures such as molecular weight and branching density. Therefore, polymerization of 1-hexene using these complexes 1–4 activated by Et2AlCl were carried out at various polymerization temperatures (20–80 °C) with the [Al]/[Ni] ratio of 500, and the results are listed in Table 2. In comparison with polymerization of ethylene, a decrease in catalytic activity was observed, which is due to the steric bulkiness of the 1-hexene monomer. The lowest catalytic activity was observed for 1 among these complexes, because bulky steric hindrance of complex 1 is unfavorable for 1-hexene monomer insertion, especially at lower temperature (no polymer was obtained at 20 °C, entry 1, Table 2).
Table 2 Polymerization of 1-hexene by α-diimine nickel complexes 1–4a
Entry |
Precat. |
Temp (°C) |
Yield (g) |
Act.b |
Mnc |
Mw/Mnc |
Bd |
Mol fraction of C6 unit (χ)d |
Linear (χL) |
Methyl-branched (χM) |
Alkyl-branched (χA) |
Polymerization conditions: Ni = 10 μmol in CH2Cl2 (2 mL); cocatalyst Et2AlCl, Al/Ni = 500; monomer concentration [1-hexene] = 1 M; time: t = 3 h; solvent: toluene, total volume: 20 mL. Activity in 104 g (mol Ni)−1 h−1. Mn and Mw/Mn determined by GPC, 105 g mol−1. Determined using 1H NMR spectroscopy.8 |
1 |
1 |
20 |
Trace |
— |
— |
— |
— |
— |
— |
— |
2 |
1 |
40 |
0.03 |
0.10 |
0.66 |
1.16 |
111.3 |
0.332 |
0.526 |
0.142 |
3 |
1 |
60 |
0.11 |
0.37 |
1.22 |
1.20 |
106.7 |
0.360 |
0.521 |
0.119 |
4 |
1 |
80 |
0.16 |
0.53 |
1.63 |
1.28 |
105.9 |
0.365 |
0.519 |
0.116 |
5 |
2 |
20 |
0.84 |
2.80 |
3.16 |
2.14 |
118.6 |
0.289 |
0.428 |
0.283 |
6 |
2 |
40 |
0.79 |
2.63 |
3.88 |
1.29 |
119.0 |
0.286 |
0.492 |
0.222 |
7 |
2 |
60 |
0.65 |
2.17 |
1.17 |
1.32 |
121.6 |
0.271 |
0.565 |
0.164 |
8 |
2 |
80 |
0.40 |
1.33 |
0.80 |
1.32 |
123.0 |
0.262 |
0.590 |
0.148 |
9 |
3 |
20 |
0.46 |
1.53 |
1.67 |
1.16 |
124.5 |
0.253 |
0.427 |
0.320 |
10 |
3 |
40 |
0.75 |
2.50 |
2.06 |
1.25 |
118.1 |
0.292 |
0.460 |
0.248 |
11 |
3 |
60 |
0.82 |
2.73 |
3.39 |
1.31 |
118.7 |
0.288 |
0.523 |
0.189 |
12 |
3 |
80 |
0.83 |
2.77 |
3.51 |
1.23 |
119.0 |
0.286 |
0.558 |
0.156 |
13 |
4 |
20 |
1.10 |
3.67 |
— |
— |
— |
— |
— |
— |
14 |
4 |
40 |
0.64 |
2.13 |
1.83 |
1.51 |
134.6 |
0.193 |
0.585 |
0.222 |
15 |
4 |
60 |
0.54 |
1.80 |
1.12 |
1.52 |
138.9 |
0.167 |
0.613 |
0.220 |
16 |
4 |
80 |
0.25 |
0.83 |
0.64 |
1.33 |
140.0 |
0.160 |
0.631 |
0.209 |
As shown in Table 2, both the catalytic activities of complexes 1, 3 and the molecular weight of the obtained polymers were slightly increased with polymerization temperatures (entries 1–4 and 9–12), but the opposite trend was observed for complexes 2 and 4 (entries 6–8 and 13–16). Complex 2 showed the highest polymer molecular weight (Mn = 3.88 × 105 g mol−1, entry 6, Table 2) at 40 °C among these catalysts. Complexes 2 and 3 showed good activity for 1-hexene polymerization to produce polymers with high molecular weight and narrow molecular weight distribution (Mw/Mn = 1.16–1.32, entries 6–12, Table 2), indicating the living characteristics of the polymerizations.
Generally, polymer microstructure is strongly dependent on catalyst structure and polymerization temperature. The branching structures of the poly(1-hexene)s produced by α-diimine nickel complexes 1–4 were determined and analyzed to study the relationship between polymer microstructure and catalyst structure.
1H NMR spectroscopy analyses have shown that the branching degree of the obtained poly(1-hexene)s are lower than the theoretical value (166.7/1000C) due to the 2,1-insertion of 1-hexene and the 1,6-enchainment (Scheme 1(iv)). The branching degree could be only tuned over a narrow range (106–140/1000C, Table 2) comparing with that in ethylene polymerization. For example, the branching densities (106–111/1000C, entries 2–4, Table 2) of the polymers obtained by complex 1 decreased with increasing temperature, but complex 4 increased slightly (135–140/1000C, entries 14–16, Table 2). However, the branching densities of the obtained polymers by complexes 2 and 3 are very close to 120 branches per 1000C, and are not depend on the polymerization temperature (entries 5–12, Table 2).
Polymers were analyzed by quantitative NMR spectroscopy to obtain the mole fractions of the three major C6 units: linear (χL), methyl-branched (χM), and alkyl-branched (χA) (see ESI† for details).8 The highly branched polymers with methyl and alkyl branches will be amorphous, while the chain-straightened (χL) polymers will be semi-crystalline. As shown in the polymer microstructures by complexes 1 and 2, the mole fractions of the three major C6 units decreased in the following order, χM > χL ≥ χA. The increasing steric hindrance of catalyst leads to enhanced insertion for 2,1-insertion of 1-hexene (χL: 1 > 2 ≈ 3 > 4) and the chain-walking reaction. This indicated that the steric hindrance of ortho-dibenzhydryl substituent on catalysts could suppress chain-transfer reactions (Fig. 5). The predominance of methyl branch (χM = 0.43–0.63) means that the predominate 1,2-insertion followed by chain-walking forms a 2,ω-enchainment (Scheme 1(iii)).
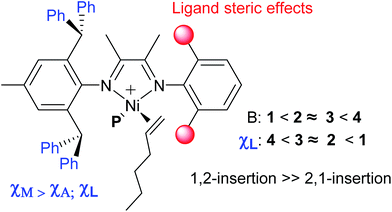 |
| Fig. 5 Ligand steric effects of the 1-hexene insertion for α-diimine nickel complexes 1–4. | |
In addition, the polymerization temperature also influences the branch-type distribution. The mole fraction of linear enchainment (χL) of the obtained amorphous polymers (no Tm observed) by complexes 1 and 3 slightly increased from 0.33 to 0.37 and 0.25 to 0.29 with increasing temperatures, while χA slightly decreased to 0.12 and 0.16 (entries 2–4 and 9–12, Table 2). The use of the complexes 2 and 4 result in a considerable decline in χL with increasing temperature (entries 5–8 and 14–16, Table 2), and the obtained polymers were amorphous.
Conclusion
In summary, polymerizations of ethylene and 1-hexene were catalyzed using a series of α-diimine nickel complexes bearing ortho-dibenzhydryl or ortho-sec-phenethyl groups activated by Et2AlCl. The ligands were modified in an attempt to change the coordination environment, steric effects and the electronic density of the metal center, eventually to modulate the activity in the polymerization of ethylene/1-hexene and to control the microstructure of polymers. The polymerization results indicated the possibility of precise microstructure control, depending on the catalyst structure and polymerization temperature, which in turn strongly affects the physical properties. The sterically bulky complex showed higher catalytic activities and thermal stability in ethylene polymerization and produced polyethylene with high molecular weight and much lower polyethylene branching density. The polyethylene branching densities (55–108/1000C) were decreased with increasing the bulkiness of the ligand and decreasing the polymerization temperature. However, sterically bulky complex is unfavorable for 1-hexene monomer insertion, and leads to low catalytic activity in 1-hexene polymerization.
Experimental section
General considerations
All experiments were carried out under a dry N2 atmosphere by using standard Schlenk techniques or a glovebox. Research grade ethylene was purified by passing it through an Agilent oxygen/moisture trap. 1H and 13C NMR spectra were recorded with a Bruker Ascend 400 spectrometer at ambient temperature unless otherwise stated. The chemical shifts of the 1H and 13C NMR spectra were referenced to tetramethylsilane (TMS). The molecular weight and the molecular weight distribution of the polymers were determined by gel permeation chromatography (GPC, Tosh, Tokyo, Japan) equipped with two linear Styragel columns at 40 °C using THF as a solvent and calibrated with polystyrene standards, and THF was employed as the eluent at a flow rate of 0.35 mL min−1. Elemental analysis was performed by the Analytical Center of the University of Science and Technology of China. Mass spectra were recorded on a P-SIMS-Gly from Bruker Daltonics Inc (EI+). 1-Hexene was purchased from Kanto Chemical Co on Aldrich Chemical Company were dried over CaH2, and distilled before use. Dichloromethane, toluene, THF, and hexane were purified by solvent purification systems. α-Diimine ligands L1–617,18 and complexes 1,4f,15a 5,18 618 were prepared according to reported procedures. Other chemicals were commercially obtained and purified with common procedures.
Synthesis of complexes 2–4
All complexes were prepared in a similar manner by the reaction of (DME)NiBr2 (DME = 1,2-dimethoxyethane) with the corresponding ligands in dichloromethane. A typical synthetic procedure of 2–4 is as follows: (DME)NiBr2 (0.31 g, 1.0 mmol) and ligand17 (1.0 mmol) were combined in a Schlenk flask under a N2 atmosphere. CH2Cl2 (20 mL) was added, and the reaction mixture was stirred at room temperature for 12 h. The resulting suspension was filtered. The solvent was removed under vacuum, and the resulting powder was washed with diethyl ether (2 × 10 mL), and then dried under vacuum at room temperature to obtain a brown solid powder.
(i-PrN^N)NiBr2 (2). (0.75 g, 93%): anal. calcd for (C49H50Br2N2Ni): C, 66.47; H, 5.69; N, 3.16. Found: C, 66.79; H, 5.60; N, 3.45. MALDI-TOF-MS (m/z): calcd for C49H50BrN2Ni: 803.2511, found: 803.1885 [M − Br]+.
(MeN^N)NiBr2 (3). (0.71 g, 95%): anal. calcd for (C45H42Br2N2Ni): C, 65.17; H, 5.10; N, 3.38. Found: C, 65.49; H, 5.42; N, 3.41. MALDI-TOF-MS (m/z): calcd for C45H42BrN2Ni: 747.1885, found: 747.1255 [M − Br]+.
(HN^N)NiBr2 (4). (0.66 g, 92%): anal. calcd for (C43H38Br2N2Ni): C, 64.45; H, 4.78; N, 3.50. Found: C, 64.49; H, 4.52; N, 3.45. MALDI-TOF-MS (m/z): calcd for C43H38BrN2Ni: 719.1572, found: 719.1244 [M − Br]+.
X-ray structure determinations
Single crystals of complexes 2, 3 and 5 for X-ray analysis were obtained by dissolving the nickel complex in CH2Cl2, followed by slow layering of the resulting solution with at room temperature. Data collections were performed at 296(2) K on a Bruker SMART APEX diffractometer with a CCD area detector, using graphite monochromated MoKα radiation (λ = 0.71073 Å). The determination of crystal class and unit cell parameters was carried out by the SMART program package. The raw frame data were processed using SAINT and SADABS to yield the reflection data file. The structures were solved by using the SHELXTL program. Refinement was performed on F2 anisotropically for all non-hydrogen atoms by the full-matrix least-squares method. The hydrogen atoms were placed at the calculated positions and were included in the structure calculation without further refinement of the parameters. Details of the crystal data and structure refinements for complex 2 are listed in Table S1.†
Procedure for ethylene polymerization
In a typical experiment, a 350 mL glass thick-walled pressure vessel was charged with required amount of AlEt2Cl, 20 mL toluene and a magnetic stir bar in the glovebox. The pressure vessel was connected to a high pressure polymerization line and the solution was degassed. The vessel was warmed to the desired temperature using an oil bath and allowed to equilibrate for 5 min. Then 2.4 μmol of nickel complex in 2 mL CH2Cl2 was injected into the vessel via syringe. With rapid stirring, the reactor was pressurized and maintained at 9.0 atm of ethylene. After the desired amount of polymerization time, the vessel was vented and terminated in acidified methanol (methanol/HCl = 50/1). The polymers obtained were adequately washed with methanol and dried under vacuum at 50 °C for 24 h. Analysis of the polyethylene branching by 1H NMR spectroscopy: branching density, branches/1000C = (CH3/3)/[(CH + CH2 + CH3)/2] × 1000. CH3 (alkyl methyl, alk-CH3, m, 0.70–0.95 ppm), CH2 and CH (alk-CH and alk-CH2, m, ca. 1.00–1.45 ppm) refer to the intensities of the methyl, methylene and methine resonances in 1H NMR spectra.19
Procedure for 1-hexene polymerization
In a typical procedure, a round-bottom Schlenk flask with stirring bar was heated 1 h at 150 °C under vacuum and cooled to room temperature. After drying the reactor under N2 atmosphere, toluene was added to the reactor. 1-Hexene was added to the toluene kept at polymerization temperature via a syringe. Then the co-catalyst AlEt2Cl was added to the toluene and the mixture was stirred for 10 min. Polymerization was started by the addition of the catalyst solution (10 μmol, 2 mL CH2Cl2) into the reactor via syringe, and the total volume of the solution was kept at 20 mL. After the desired amount of time, the polymerization was terminated by adding 50 mL of the acidified methanol (methanol/HCl = 50/1). The polymers obtained were adequately washed with methanol and dried in vacuum at 40 °C to a constant weight. Poly(1-hexene)s show long methylene sequences [linear (χL)], methyl branch (χM) and alkyl branches [i.e., butyl and longer than hexyl branches (χA)].4e,17 Analysis of the poly(1-hexene) branching by 1H NMR spectroscopy:8 branching density, branches/1000C = (CH3/3)/[(CH + CH2 + CH3)/2] × 1000. CH3 (alk-CH3 (χM), d, 0.70–0.87 ppm; alkyl methyl (χA), t, 0.87–0.95 ppm), CH2 and CH (alk-CH and alk-CH2, m, ca. 1.0–1.45 ppm) refer to the intensities of the methyl, methylene and methine resonances in 1H NMR spectra. 2,1-Insertion was calculated by the following equation: 2,1-Ins.% = χL = 2,1-insertion = (166.7 − B)/166.7,11b,12 B = branches per 1000C; χM + χA = 1 − χL.
Conflicts of interest
There are no conflicts to declare.
Acknowledgements
This work was supported by National Natural Science Foundation of China (NSFC, 21704094), the Chinese Postdoctoral Science Foundation (2017M612076), Advanced Catalysis and Green Manufacturing Collaborative Innovation Center (ACGM2016-06-01) and Yixing Taodu Ying Cai Program.
Notes and references
-
(a) S. D. Ittel, L. K. Johnson and M. Brookhart, Chem. Rev., 2000, 100, 1169–1203 CrossRef CAS PubMed;
(b) T. R. Younkin, E. F. Connor, J. I. Henderson, S. K. Friedrich, R. H. Grubbs and D. A. Bansleben, Science, 2000, 287, 460–462 CrossRef CAS PubMed;
(c) J. M. Rose, F. Deplace, N. A. Lynd, Z. Wang, A. Hotta, E. B. Lobkovsky, E. J. Kramer and G. W. Coates, Macromolecules, 2008, 41, 9548–9555 CrossRef CAS;
(d) C. Chen, S. Luo and R. F. Jordan, J. Am. Chem. Soc., 2008, 130, 12892–12893 CrossRef CAS PubMed;
(e) C. Chen, S. Luo and R. F. Jordan, J. Am. Chem. Soc., 2010, 132, 5273–5284 CrossRef CAS PubMed;
(f) D. Zhang and C. L. Chen, Angew. Chem., Int. Ed., 2017, 56, 14672–14676 CrossRef CAS PubMed.
-
(a) R. Nakano and K. Nozaki, J. Am. Chem. Soc., 2015, 137, 10934–10937 CrossRef CAS PubMed;
(b) X. L. Sui, S. Y. Dai and C. L. Chen, ACS Catal., 2015, 5, 5932–5937 CrossRef CAS;
(c) M. Chen, W. P. Zou, Z. G. Cai and C. L. Chen, Polym. Chem., 2015, 6, 2669–2676 RSC;
(d) Y. Ota, S. Ito, M. Kobayashi, S. Kitade, K. Sakata, T. Tayano and K. Nozaki, Angew. Chem., Int. Ed., 2016, 55, 7505–7509 CrossRef CAS PubMed;
(e) Z. Jian, L. Falivene, G. Boffa, S. Ortega Sánchez, L. Caporaso, A. Grassi and S. Mecking, Angew. Chem., Int. Ed., 2016, 55, 14378–14595 CrossRef CAS PubMed;
(f) B. P. Yang, S. Y. Xiong and C. L. Chen, Polym. Chem., 2017, 8, 6272–6276 RSC;
(g) T. Liang and C. L. Chen, Organometallics, 2017, 36, 2338–2344 CrossRef CAS;
(h) Y. N. Na, D. Zhang and C. L. Chen, Polym. Chem., 2017, 8, 2405–2409 RSC.
-
(a) J. C. Yuan, F. Z. Wang, W. B. Xu, T. J. Mei, J. Li, B. N. Yuan, F. Y. Song and Z. Jia, Organometallics, 2013, 32, 3960–3968 CrossRef CAS;
(b) L. Zhu, Z. S. Fu, H. J. Pan, W. Feng, C. L. Chen and Z. Q. Fan, Dalton Trans., 2014, 43, 2900–2906 RSC;
(c) W. P. Zou and C. L. Chen, Organometallics, 2016, 35, 1794–1801 CrossRef CAS;
(d) R. K. Wang, M. H. Zhao and C. L. Chen, Polym. Chem., 2016, 7, 3933–3938 RSC;
(e) S. Yuan, E. Yue, C. Wen and W. H. Sun, RSC Adv., 2016, 6, 7431–7438 RSC;
(f) P. Huo, J. Li, W. Liu, G. Mei and X. H. He, RSC Adv., 2017, 7, 51858–51863 RSC;
(g) Y. N. Na, X. Wang, K. Lian, Y. Zhu, W. Li, Y. Luo and C. L. Chen, ChemCatChem, 2017, 9, 1062–1066 CrossRef CAS;
(h) M. H. Zhao and C. L. Chen, ACS Catal., 2017, 7, 7490–7494 CrossRef CAS.
-
(a) J. C. Yuan, F. Z. Wang, B. N. Yuan, Z. Jia, F. Y. Song and J. Li, J. Mol. Catal. A: Chem., 2013, 370, 132–139 CrossRef CAS;
(b) F. Z. Wang, R. Tanaka, Q. S. Li, J. C. Yuan, Y. Nakayama and T. Shiono, J. Mol. Catal. A: Chem., 2015, 398, 231–240 CrossRef CAS;
(c) L. H. Guo and C. L. Chen, Sci. China: Chem., 2015, 58, 1663–1673 CrossRef CAS;
(d) B. K. Long, J. M. Eagan, M. Mulzer and G. W. Coates, Angew. Chem., Int. Ed., 2016, 55, 7222–7226 CrossRef;
(e) F. Z. Wang, R. Tanaka, Z. G. Cai, Y. Nakayama and T. Shiono, Polymers, 2016, 8, 160 CrossRef;
(f) L. H. Guo, S. Y. Dai and C. L. Chen, Polymers, 2016, 8, 37 CrossRef;
(g) L. H. Guo, W. Liu and C. L. Chen, Mater. Chem. Front., 2017, 1, 2487–2494 RSC.
-
(a) G. Chen, X. S. Ma and Z. Guan, J. Am. Chem. Soc., 2003, 125, 6697–6704 CrossRef CAS PubMed;
(b) B. K. Bahuleyan, G. W. Son, D. W. Park, C. S. Ha and I. Kim, J. Polym. Sci., Part A: Polym. Chem., 2008, 46, 1066–1082 CrossRef CAS;
(c) C. Chen and R. F. Jordan, J. Am. Chem. Soc., 2010, 132, 10254–10255 CrossRef CAS PubMed;
(d) R. K. Wang, X. L. Sui, W. M. Pang and C. L. Chen, ChemCatChem, 2016, 8, 434–440 CrossRef CAS;
(e) M. Li, X. B. Wang, Y. Luo and C. L. Chen, Angew. Chem., Int. Ed., 2017, 129, 11762–11767 CrossRef.
-
(a) E. Yue, L. Zhang, Q. Xing, X. P. Cao, X. Hao, C. Redshaw and W. H. Sun, Dalton Trans., 2014, 43, 423–431 RSC;
(b) Y. Ota, S. Ito, J. Kuroda, Y. Okumura and K. Nozaki, J. Am. Chem. Soc., 2014, 136, 11898–11901 CrossRef CAS PubMed;
(c) Z. B. Jian, B. C. Moritz and S. Mecking, J. Am. Chem. Soc., 2015, 137, 2836–2839 CrossRef CAS PubMed;
(d) L. H. Guo, S. Y. Dai, X. L. Sui and C. L. Chen, ACS Catal., 2016, 6, 428–441 CrossRef CAS;
(e) X. H. Hu, S. Y. Dai and C. L. Chen, Dalton Trans., 2016, 45, 1496–1503 RSC;
(f) K. Lian, Y. Zhu, W. Li, S. Y. Dai and C. L. Chen, Macromolecules, 2017, 50, 6074–6080 CrossRef CAS.
-
(a) Y. Chen, L. Wang, H. Yu, Y. Zhao, R. Sun, G. Jing, J. Huang, H. Khalid, N. M. Abbasi and M. Akram, Prog. Polym. Sci., 2015, 45, 23–43 CrossRef CAS;
(b) S. Y. Dai, X. L. Sui and C. L. Chen, Chem. Commun., 2016, 52, 9113–9116 RSC;
(c) X. L. Sui, C. W. Hong, W. M. Pang and C. L. Chen, Mater. Chem. Front., 2017, 1, 967 RSC;
(d) M. Chen and C. L. Chen, ACS Catal., 2017, 7, 1308–1312 CrossRef CAS.
-
(a) T. Vaidya, K. Klimovica, A. M. LaPointe, I. Keresztes, E. B. Lobkovsky, O. Daugulis and G. W. Coates, J. Am. Chem. Soc., 2014, 136, 7213–7216 CrossRef CAS PubMed;
(b) F. Z. Wang, S. S. Tian, R. P. Li, W. M. Li and C. L. Chen, Chin. J. Polym. Sci., 2018, 36, 1–6 CrossRef;
(c) C. Y. Rong, F. Z. Wang, W. M. Li and M. Chen, Organometallics, 2017, 36, 4458–4464 CrossRef CAS.
-
(a) L. H. Guo, H. Gao, Q. Guan, H. Hu, J. Deng, J. Liu, F. Liu and Q. Wu, Organometallics, 2012, 31, 6054–6062 CrossRef CAS;
(b) Q. Mahmood, Y. N. Zeng, X. X. Wang, Y. Sun and W. H. Sun, J. Organomet. Chem., 2015, 798, 401–407 CrossRef;
(c) Q. Mahmood, Y. N. Zeng, X. X. Wang, Y. Sun and W. H. Sun, Dalton Trans., 2017, 46, 6934–6947 RSC.
-
(a) S. Kong, K. Song, T. Liang, C. Y. Guo, W. H. Sun and C. Redshaw, Dalton Trans., 2013, 42, 9176–9187 RSC;
(b) C. J. Stephenson, J. P. McInnis, C. Chen, M. P. Weberski, A. Motta, M. Delferro and T. J. Marks, ACS Catal., 2014, 4, 999–1003 CrossRef CAS;
(c) H. Hu, H. Gao, D. Chen, G. Li, Y. Tan, G. Liang, F. Zhu and Q. Wu, ACS Catal., 2015, 5, 122–128 CrossRef CAS;
(d) F. Z. Wang, R. Tanaka, Z. G. Cai, Y. Nakayama and T. Shiono, Macromol. Rapid Commun., 2016, 37, 1375–1381 CrossRef CAS PubMed;
(e) F. Z. Wang, R. Tanaka, Z. G. Cai, Y. Nakayama and T. Shiono, Polymer, 2017, 127, 88–100 CrossRef CAS.
-
(a) F. S. Liu, H. Y. Gao, Z. L. Hu, H. B. Hu, F. M. Zhu and Q. Wu, J. Polym. Sci., Part A: Polym. Chem., 2012, 50, 3859–3866 CrossRef CAS;
(b) J. Liu, D. R. Chen, H. Wu, Z. F. Xiao, H. Y. Gao, F. M. Zhu and Q. Wu, Macromolecules, 2014, 47, 3325–3331 CrossRef CAS.
- D. F. Zhang, E. T. Nadres, M. Brookhart and O. Daugulis, Organometallics, 2013, 32, 5136–5143 CrossRef CAS.
-
(a) A. E. Cherian, J. M. Rose, E. B. Lobkovsky and G. W. Coates, J. Am. Chem. Soc., 2005, 127, 13770–13771 CrossRef CAS PubMed;
(b) J. M. Rose, A. E. Cherian and G. W. Coates, J. Am. Chem. Soc., 2006, 128, 4186–4187 CrossRef CAS PubMed.
-
(a) D. H. Camacho and Z. Guan, Macromolecules, 2005, 38, 2544–2546 CrossRef CAS;
(b) D. H. Camacho, E. V. Salo, J. W. Ziller and Z. Guan, Angew. Chem., Int. Ed., 2004, 43, 1821–1825 CrossRef CAS PubMed.
-
(a) J. L. Rhinehart, L. A. Brown and B. K. Long, J. Am. Chem. Soc., 2013, 135, 16316–16319 CrossRef CAS PubMed;
(b) J. L. Rhinehart, N. E. Mitchell and B. K. Long, ACS Catal., 2014, 4, 2501–2504 CrossRef CAS.
-
(a) S. Y. Dai, X. L. Sui and C. L. Chen, Angew. Chem., Int. Ed., 2015, 54, 9948–9953 CrossRef CAS PubMed;
(b) S. Y. Dai and C. L. Chen, Angew. Chem., Int. Ed., 2016, 55, 13281–13285 CrossRef CAS PubMed.
-
(a) S. Y. Dai, S. X. Zhou, W. Zhang and C. L. Chen, Macromolecules, 2016, 49, 8855–8862 CrossRef CAS;
(b) L. H. Guo, C. Zou, S. Y. Dai and C. L. Chen, Polymers, 2017, 9, 122 CrossRef.
- F. Z. Wang, J. C. Yuan, F. Y. Song, J. Li, Z. Jia and B. N. Yuan, Appl. Organomet. Chem., 2013, 27, 319–327 CrossRef CAS.
-
(a) J. C. Jenkins and M. Brookhart, Organometallics, 2003, 22, 250–256 CrossRef CAS;
(b) E. F. McCord, S. J. McLain, L. T. J. Nelson, S. D. Ittel, D. Tempel, C. M. Killian, L. K. Johnson and M. Brookhart, Macromolecules, 2007, 40, 410–420 CrossRef CAS.
Footnote |
† Electronic supplementary information (ESI) available: NMR spectra of ligands and polymers; GPC curves of polymers. CCDC 1576125, 1576349, 1468457 contain the supplementary X-ray crystallographic data for complexes 2, 3 and 5. For ESI and crystallographic data in CIF or other electronic format see DOI: 10.1039/c7ra11783c |
|
This journal is © The Royal Society of Chemistry 2017 |
Click here to see how this site uses Cookies. View our privacy policy here.