DOI:
10.1039/C7RA11162B
(Paper)
RSC Adv., 2017,
7, 54892-54897
A turn-on fluorescent probe for Cd2+ detection in aqueous environments based on an imine functionalized nanoscale metal–organic framework†
Received
10th October 2017
, Accepted 26th November 2017
First published on 1st December 2017
Abstract
A novel fluorescent probe was synthesized and explored to detect cadmium ions (Cd2+) based on an imine functionalized metal–organic framework (MOF). The nanoscale MOF probe (∼100 nm) displayed a turn-on fluorescence in aqueous environments with the addition of Cd2+ which could be ascribed to the interaction between the C
N group and specific metal ions, presenting a low-toxicity, highly selective and sensitive detection method for Cd2+ with a detection limit of 0.336 μM (37.8 ppb) and a broad linear range of 0–500 μM, which is promising for application in intracellular sensing and imaging of Cd2+. The probing mechanism was studied and discussed in detail.
Introduction
As one of the extremely toxic and carcinogenic heavy metal ions, the cadmium ion (Cd2+) is widely used in many industrial fields such as the production of metal alloys, batteries, electroplating films and the control rods of atomic/nuclear reactors.1 However, Cd2+ can be easily absorbed and accumulated in the human body via food chains with a long biological half-life (about 30 years).2 Meanwhile, the intake of Cd2+ can damage immune systems and increase the incidence of various diseases such as bone pain, pathological fractures, and even kidney cancer and lung cancer.3 Therefore, the detection of trace Cd2+ is critically important.
Up to now, various techniques have been developed for the determination of Cd2+ such as atomic absorption spectrometry (AAS),4 atom emission spectrometry (AES),5 inductively coupled plasma mass spectrometry (ICP-MS),6 and synchrotron radiation X-ray spectrometry (XRS).7 However, normal restrictions of these analytical methods such as sophisticated instruments, complicated sample preparation and time-consuming processes made cheap, remote and on-site detection far from available. It is of vital importance to develop a simple, rapid and sensitive technique for Cd2+ detection. Recently, fluorescent sensors have been considered as a promising and compelling candidate, which can sensitively determine Cd2+ ions in different environments through the fluorescence intensity changes or wavelength shifts and avoid the complicated time-consuming identification problem.8 A lot of fluorescent Cd2+ probes based on small molecules and polymers have been reported.9
Among various fluorescent chemosensors, metal–organic frameworks (MOFs) have aroused tremendous attention during the past decades. By taking advantages of their fascinating luminescent properties, size-adjustable pores, and modifiable recognized sites through postsynthetic modification (PSM),10 a variety of MOFs have been explored for detecting metal ions including Ag+,11 Al3+,12 Zn2+,13 Cu2+,14 Pb2+,15 Hg2+ (ref. 16) etc. However, only a few examples of MOF probes capable of detecting Cd2+ ions, which were mainly based on luminescent lanthanide MOFs, have been reported up to date.17 There still remains two challenges in the design of MOF probes for highly selective and sensitive sensing of Cd2+ in water samples. One is high accuracy with low deviation of the probe, thus the nanoscale size and stable luminescence are expected. The other is satisfying selectivity and anti-interference ability toward congener elements, for which the sensing mechanism of the Cd2+ probe should be based on different binding affinities with various metal ions, instead of selective coordination alone.8 Thus, a nanoscale MOF whose recognition site has a better affinity with Cd2+ may be a possible solution to above problems. Given the good affinity between cadmium ions with nitrogen atoms, we can utilize the imine group as the recognition site.18 For this purpose, as the corresponding imine linkers are not commercially available and not thermal stable, the PSM strategy is needed to realize the chemical transformation from amine group.19
Enlightened by the above thoughts, we herein developed a novel nanoscale fluorescent probe based on imine functionalized MOFs via a condensation reaction of UiO-66-NH2. As a promising method, PSM of MOFs has become a new tool for exploiting and expanding the unique properties of MOFs. By transforming amine (–NH2) group of UiO-66-NH2 to imine (–N
C) group, we synthesized a 3D porous MOF UiO-66-N
CH2 as a novel Cd2+ fluorescent probe (Scheme 1). Due to the strong affinity and the coordination between Cd2+ with nitrogen atoms in imine group, the MOF probe exhibited fast response, high selectivity, and excellent sensitivity for Cd2+ detection in aqueous solution.
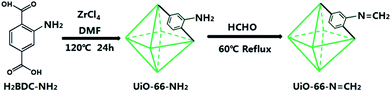 |
| Scheme 1 Schematic illustration of design of MOF-based fluorescent turn-on probe for Cd2+. Postsynthetic modification of UiO-66-NH2 to UiO-66-N CH2 via condensation reaction. | |
Experimental
Chemical reagents
All chemicals and reagents were purchased at least of analytical grade and used without further purification. The standard solutions of metal ions (Cd2+, Na+, Ca2+, Mg2+, Cu2+, Zn2+, Pb2+, Al3+ and Fe3+) were prepared from solving their nitrates in deionized water with the concentration of 0.01 M.
Physical measurements
Powder X-ray diffraction (PXRD) patterns were measured within the 2θ range of 3–50° on X'Pert Pro X-ray diffractometer with Cu-Kα radiation (λ = 1.542 Å) at room temperature. Thermal gravimetric analysis (TGA) were carried out on a Netzsch STA449C system at a heating rate of 10 K min−1 from 30 °C to 800 °C under nitrogen atmosphere in the Al2O3 crucibles. Fourier transform infrared (FTIR) spectra were recorded within 4000–400 cm−1 range on Thermo Fisher Nicolet iS10 spectrometer using KBr pellets. The pellets were prepared by mixing the MOF (about 1 mg) with KBr (about 150 mg). The mixture was then carefully grinded and compressed under the pressure of 10 kPa to form a transparent pellet. 1H NMR spectra were recorded on a Bruker Advance DMX 500 spectrometer using tetramethylsilane (TMS) as an internal standard. The emission and excitation spectra for the samples were recorded by a Hitachi F-4600 fluorescence spectrometer. The morphologies of MOFs were recorded by a S-4800 field-emission scanning electron microscope (FE-SEM). X-ray photoelectron spectroscopy (XPS) was recorded with a Thermo Fisher ESCALAB 250Xi X-ray photoelectron spectrometer with Al Kα X-ray radiation for excitation. The outer luminescent quantum efficiency was determined using an integrating sphere (BaSO4 coating) from Edinburgh FLSP920 fluorescence spectrometer. The quantum yield can be defined as the integrated intensity of the luminescence signal divided by the integrated intensity of the absorption signal.
Synthesis of UiO-66-NH2
UiO-66-NH2 was synthesized using a solvothermal method with a modification of previous reports.20 Anhydrous ZrCl4 (116.6 mg, 0.5 mmol, 1 equiv.), 2-aminoterephthalic acid (108.8 mg, 0.6 mmol, 1.2 equivs), benzoic acid (1.8320 g, 15 mmol, 30 equivs) and deionized water (36.0 μL, 4 equivs) were dissolved in 10 mL N,N-dimethylformamide (DMF) with ultrasonication for 15 min. Then the homogeneous solution was transferred into a teflon lined autoclave vessel and placed in a 120 °C oven for 24 h. After cooling overnight, the as-synthesized product was rinsed three times with DMF and ethanol to remove unreacted reagents. Then the faint yellow powders were isolated by centrifugation.
Synthesis of UiO-66-N
CH2
In a typical synthesis, the activated and dried UiO-66-NH2 (100 mg, 0.34 mmol of –NH2) was dispersed in one flask (50 mL capacity) with 15 mL of acetone. Six equivalents (per NH2 functionality, 163 μL) of 40% HCHO (formaldehyde) was added by microlitre pipette. After ultrasonication treated for several minutes, the flask with the mixed solution was heated under reflux and stirring in 60 °C oil bath for 4 h to produce the imine functionalized compound. After repeated centrifugation and rinsing using ethanol for three times, the solid powders was then dried under vacuum at 60 °C overnight to yield the final product, UiO-66-N
CH2.
Luminescent experiments
The photoluminescence properties of UiO-66-N
CH2 in different metal cation solutions were studied at 25 °C. In a typical experiment, 2.0 mg powder samples of UiO-66-N
CH2 were weighed and introduced into 2.0 mL aqueous solution of M(NO3)n (Mn+ = Cd2+, Na+, Ca2+, Mg2+, Cu2+, Zn2+, Pb2+, Al3+ and Fe3+) and dispersed ultrasonically to form suspensions for luminescent measurements. Then Cd2+ solutions at various concentrations are prepared through dilution of standard solution (0.01 M in deionized water). All the luminescent properties were observed upon excitation at 342 nm.
Digestion and analysis by 1H NMR
1H NMR spectra of MOFs were recorded on a Bruker Advance DMX 500 spectrometer. Approximately 3 mg of UiO-66-NH2 and UiO-66-N
CH2 were digested with sonication in 600 μL of DMSO-d6 and 30 μL of DCl.
Cell culture and in vitro cytotoxicity assay
Rat pheochromocytoma (PC12) cells were incubated in a humidified incubator (37 °C, 5% CO2) for three days employing Dulbecco's modified eagle medium (DMEM, Neuronbc) with 10% fetal bovine serum (FBS), and 1% penicillin/streptomycin (P/S, Boster). The toxicity of UiO-66-N
CH2 was assessed by MTT assays. The crystals were cultured with PC12 cells for 24 h at five concentrations (5, 20, 50, 80, and 100 μg mL−1) in 96-well assay plates (200 μL total volume per well). The MTT solution (50 μL) was added to each well tested and the plates were cultured for 4 h. The medium was removed and the blue dye appeared after the addition of DMSO (150 mL). Absorbance values of samples were determined with a microplate reader at λ = 490 nm. The cell viability was calculated as the ratio of the absorbance of the sample well to that of the control. The results were repeated in triplicate and averaged.
Results and discussion
Crystal synthesis and characterization
As the precursor of the MOF probe in this work, UiO-66-NH2 was prepared via the solvothermal reaction of the H2BDC-NH2 ligand with ZrCl4 in the solvent DMF (as shown in Scheme 1). UiO-66-NH2 has attracted intense research interests recently by virtue of the outstanding chemical and thermal stabilities.21 On account of the unparticipating amine groups of the ligand in coordination to the Zr6-octahedra [Zr6O4(OH)4] clusters, these free functional groups are thus potentially available for undergoing various chemical modifications. Therefore, the fluorescent probe UiO-66-N
CH2 was prepared through postsynthetic modification of UiO-66-NH2 via aldehyde-amine condensation reaction. In a typical synthesis (Scheme 1), freshly activated UiO-66-NH2 was treated by formaldehyde under reflux in acetone for 4 h to produce the corresponding imine compound UiO-66-N
CH2.
The phase purity of UiO-66-NH2 and UiO-66-N
CH2 were confirmed by overlapping powder X-ray diffraction (PXRD) patterns of simulated and as-synthesized samples. As shown in Fig. 1a, the PXRD patterns of as-synthesized UiO-66-NH2 and UiO-66-N
CH2 matched well with simulated PXRD pattern of UiO-66, which can be featured by two peaks at 7.4° and 8.5°, representing the crystal plane (111) and (200) respectively.22
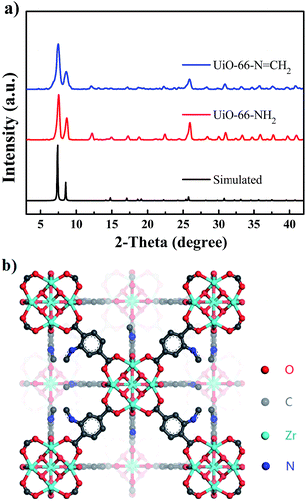 |
| Fig. 1 Synthesis and characterization of UiO-66-NH2 and UiO-66-N CH2. (a) PXRD patterns of the simulated UiO-66-NH2 (black), as-synthesized UiO-66-NH2 (red) and UiO-66-N CH2 (blue). (b) Structure depiction of UiO-66-N CH2. | |
Furthermore, to confirm the thermal stability and structural integrity of two MOFs at elevated temperatures, samples were examined by TGA (Fig. S1†). The TGA curves revealed that UiO-66-NH2 and the ethanol-treated UiO-66-N
CH2 will lose entrapped solvent molecules at 100 °C and 80 °C, respectively. Similarly, both two MOFs showed high thermal stability with decomposition temperatures up to 400 °C. The permanent porosity of the prepared UiO-66-NH2 was determined by N2 adsorption–desorption isotherm after guest removal, exhibiting a BET surface area of 934.4 m2 g−1. The reactive nature of the free –NH2 groups and the permanent porosity of UiO-66-NH2 are potentially available for conducting various modifications. And after postsynthetic modification, the synthesized UiO-66-N
CH2 displayed analogous N2 adsorption–desorption behaviour (Fig. S2†), which showed a decreased BET surface area of 689.3 m2 g−1 as expected, indicating that UiO-66-N
CH2 still maintains its permanent pores.
Field-emission scanning electron microscopy (FE-SEM) images (Fig. 2) were taken to reveal the morphology of the synthesized UiO-66-NH2. In this work, 30 equivalents benzonic acid were employed as the modulator, and the as-synthesized faint yellow powders displayed perfect octahedral morphology with particle sizes about 100 nm. It is also worth mentioning here that a smaller particle size is not only conducive to guest molecules transfer within UiO-66-NH2 crystals, but also critical to be developed as a fluorescent probe. Additionally, these materials were characterized by FTIR spectroscopy (Fig. S3†). The FTIR spectra of UiO-66-NH2 show two peaks at 3345 and 3456 cm−1 due to the existence of the symmetric and asymmetric stretching of primary amines, which demonstrates that the amino groups are free for interaction in these structures. But FTIR spectroscopy is less revealing with the framework –NH2 stretching bands dominating, thus obscuring any imine (C
N) and –OH stretches. In addition, unambiguous characterization was provided by liquid 1H NMR analysis (Fig. S4†). Samples of UiO-66-NH2 and UiO-66-N
CH2 were digested and dissolved in dilute DCl/D2O/DMSO-d6 solution and then analyzed by 1H NMR spectroscopy, which confirmed the formation of the imine compound by the appearance of new signals (7.84–7.85 ppm, d, (H)C
N) (Fig. S4d†). It is obvious that the aromatic signals of the amine (7 ppm, d, 1H; 7.34 ppm, s, 1H; 7.77 ppm, d, 1H) (Fig. S4c and d†) does not completely disappear, indicating not full conversion to the imine form.
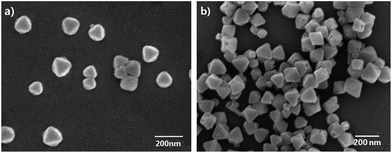 |
| Fig. 2 FE-SEM images of UiO-66-NH2 and UiO-66-N CH2. | |
Photoluminescence properties
The photoluminescent (PL) properties of the MOF probe obtained after successful PSM was first investigated at room temperature. The excitation and emission spectra of UiO-66-N
CH2 are shown in Fig. 3a. It is clearly observed that the excitation spectra collected in the wavelength range of 200–400 nm are dominated by a broad absorption band in ultraviolet region centered at about 342 nm. The excitation spectra suggest that the probe can absorb the energy of ultraviolet light efficiently. Upon 342 nm radiation in water, crystals of UiO-66-N
CH2 exhibits a broad peak centered at 468 nm.
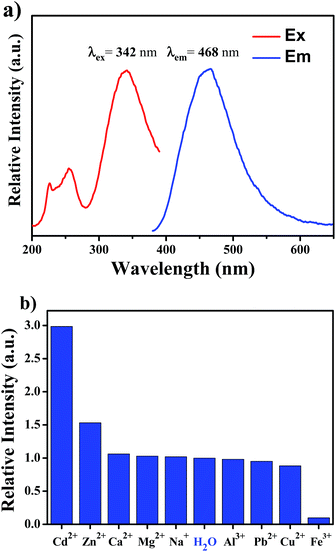 |
| Fig. 3 (a) Excitation (red line) and emission (blue line) spectra of UiO-66-N CH2; (b) the intensities at 468 nm for UiO-66-N CH2 dispersed in various metal ion aqueous solutions (500 μM) upon excitation at 342 nm. | |
To examine the potential of UiO-66-N
CH2 as a fluorescent probe for metal ions in aqueous environment, the luminescent stability of the sample in aqueous solution has been investigated. During storage in water for 2 weeks, the luminescence intensity of the UiO-66-N
CH2 suspension shows no significant change (Fig. S5a†), implying the excellent stability of fluorescence in water. Moreover, UiO-66-N
CH2 also exhibits good pH-independent fluorescence in the pH range of 4–10 (Fig. S5b†), which indicates that the influence of slight fluctuation of pH value on the fluorescent intensity and sensing properties may be neglected.
Sensing of Cd2+
High selectivity over competing species is of vital importance for a fluorescent probe. For verifying the sensing selectivity of UiO-66-N
CH2 of metal ions, the suspension-state luminescent experiments are performed. Different kinds of metal ions (Cd2+, Na+, Ca2+, Mg2+, Cu2+, Zn2+, Pb2+, Al3+ and Fe3+) have been introduced into the system of UiO-66-N
CH2, forming 500 μM aqueous suspensions after ultrasonication treatment for 1 minute. The luminescent spectra showed that the PL intensity of UiO-66-N
CH2 suspension is largely dependent on the interacted metal ion. As can be seen in Fig. 3b and S6,† only Fe3+ causes the quenching effect on the luminescence intensity of UiO-66-N
CH2. The addition of Cd2+ ion enhanced the luminescence intensity most remarkably by about 3-fold as much as that of the original one, which is the most drastic enhancement effect among all the tested ions. Meanwhile, the luminescence intensity of UiO-66-N
CH2 showed small increase by Zn2+, which is an important disruptor. The rest of other metal ions had a negligible effect on the luminescence of UiO-66-N
CH2. The above results prove that UiO-66-N
CH2 has a high selectivity for the specific recognition of Cd2+ in aqueous environment.
In Fig. 4, we measured the luminescence responses of UiO-66-N
CH2 (1 mg mL−1) immersed in Cd2+ aqueous solutions with different concentrations. It is obvious that the luminescence intensity of UiO-66-N
CH2 incorporated with Cd2+ displays a strong dependence on the concentration of Cd2+ ion. As can be seen in Fig. 4a, the emission intensity of UiO-66-N
CH2 increases gradually with the increase of Cd2+ concentration from 0 to 500 μM. Quantitatively, the enhancing effect can be analyzed by the Stern–Volmer equation:
where
I0 and
I are the luminescence intensity of the suspension at 468 nm in the absence and presence of Cd
2+, respectively,
KSV represents the Stern–Volmer quenching constant, and [Cd
2+] is the concentration of Cd
2+. There is a good linear relationship (correlation coefficient
R2 = 0.999) between
I/
I0 and [Cd
2+] in a wide concentration range (
Fig. 4b), indicating that the enhancing effect of Cd
2+ on the luminescence of
UiO-66-N
CH2 agrees the Stern–Volmer mode well. The
KSV value is calculated to be 3.81 × 10
3 M
−1, which reveals a strong enhancing effect on the
UiO-66-N
CH2 luminescence. From the slope of the fitting line (slope) and the standard deviation (SD) for eleven replicating fluorescence measurements of blank solutions (Fig. S7
†), the detection limit (3SD/slope) of
UiO-66-N
CH2 towards Cd
2+ was calculated to be 0.336 μM (37.8 ppb).
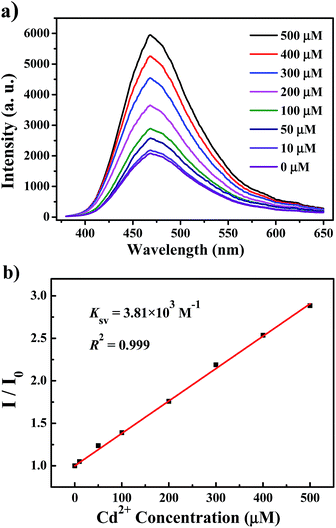 |
| Fig. 4 (a) The luminescent emission spectra (excited at 342 nm) of UiO-66-N CH2 (1 mg mL−1) in Cd2+ solutions with different concentrations. (b) Stern–Volmer plots of I/I0 versus Cd2+ concentration and the fitting line in water (R2 = 0.999; KSV = 3.81 × 103 M−1). | |
To evaluate the cytotoxicity of UiO-66-N
CH2, rat pheochromocytoma (PC12) cells were selected for in vitro studies using the MTT assay, considering the wide use of PC12 cells in assessing the cytotoxicity of nanomaterials.23 The MTT assay was carried out by incubating PC12 cells with UiO-66-N
CH2 for 24 h at various concentrations ranging from 5 to 100 μg mL−1. As revealed in Fig. S8,† the cell viability decreased slightly with the increase of the UiO-66-N
CH2 concentration, but the cell viability was still higher than 80% even at 100 μg mL−1, meaning that UiO-66-N
CH2 was a biocompatible probe with negligible cytotoxicity.
The enhancing effect on luminescence of UiO-66-N
CH2 by Cd2+ ions can be ascribed to the interaction between Cd2+ ions and the C
N group within UiO-66-N
CH2 which facilitates the efficiency of deactivation from excited state to ground state. To explore the possible mechanism for such luminescence enhancement by Cd2+, N 1s and Cd 3d X-ray photoelectron spectroscopy (XPS) studies were carried out on probe and Cd2+-incorporated probe. The N 1s peak from free imine nitrogen atoms at 399.5 eV in H2O is shifted to 400.2 eV on the addition of Cd2+ (Fig. 5), indicating the weak binding between Cd2+ and imine nitrogen atoms in Cd2+-incorporated probe. Such coordination binding of Cd2+ with the imine group of the ligand can reduce non-radiative transition and decrease energy loss, thus resulting in a ligand-centered charge transfer which may lead to the enhancing effect. The peak at 413.2 eV in the N 1s XPS spectra for Cd2+-incorporated probe is attributed to Cd 3d, as for the other peak at 406.6 eV, it should be ascribed to the signal of NO3− counterions.24 As a control experiment, we also investigated the fluorescent response of UiO-66-NH2 toward Cd2+. After immersing into the Cd2+ solution (500 μM), no obvious change was found from the luminescence intensity of UiO-66-NH2, indicating the important role of the C
N group in Cd2+ sensing.
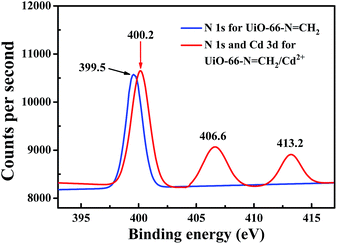 |
| Fig. 5 XPS spectra of the original probe (blue) and Cd2+-incorporated probe (red) activated in 10 mM aqueous solution of Cd(NO3)2. | |
As a luminescent probe, UiO-66-N
CH2 is not only highly selective and sensitive for Cd2+ detection, but also water-stable and nano-scale, which enable the probe to be applicable for Cd2+ sensing in aqueous and intracellular environments.
Conclusions
In summary, a novel turn-on fluorescent probe based on imine functionalized nano MOF for Cd2+ detection has been synthesized through a post-synthetic approach. The MOF probe displayed high sensitivity (detection limit, 0.336 μM), broad linear range (0–500 μM) and excellent selectivity toward Cd2+ over other relevant metal ions. Moreover, it is water-stable, nano-scale and low-toxic. All these indicate the potential of UiO-66-N
CH2 for intracellular Cd2+ turn-on sensing and imaging.
Conflicts of interest
There are no conflicts to declare.
Acknowledgements
The authors gratefully acknowledge the financial support for this work from the National Natural Science Foundation of China (Nos. 51372221, 51472217, 51432001, 51632008, and U1609219), and Zhejiang Provincial Natural Science Foundation of China (No. LZ15E020001).
Notes and references
-
(a) A. M. S. Mendes, G. P. Duda, C. W. A. Nascimento and M. O. Silva, Sci. Agric., 2006, 63, 328 CrossRef CAS;
(b) M. Lenzen, Energy Convers. Manage., 2008, 49, 2178 CrossRef CAS.
-
(a) L. T. Lu, I. C. Chang, T. Y. Hsiao, Y. H. Yu and H. W. Ma, Environ. Sci. Pollut. Res., 2007, 14, 49 CrossRef CAS;
(b) M. Zhang, Y. Liu and B. Ye, Analyst, 2011, 137, 601 RSC.
-
(a) Y. Itokawa, K. Nishino, M. Takashima, T. Nakata, H. Katio, E. Okamoto, K. Daijo and J. Kawamura, Environ. Res., 1978, 15, 206 CrossRef CAS PubMed;
(b) S. Satarug, J. R. Baker, S. Urbenjapol, M. Haswell-Elkins, P. E. Reilly, D. J. Williams and M. R. Moore, Toxicol. Lett., 2003, 137, 65 CrossRef CAS PubMed.
- A. N. Anthemidis, Microchim. Acta, 2008, 160, 455 CrossRef CAS.
- A. C. Davis, C. P. Calloway Jr and B. T. Jones, Talanta, 2007, 71, 1144 CrossRef CAS PubMed.
- E. A. Hutton, J. T. van Elteren, B. Ogorevc and M. R. Smyth, Talanta, 2004, 63, 849 CrossRef CAS PubMed.
- E. Marguí, I. Queralt and M. Hidalgo, J. Anal. At. Spectrom., 2013, 28, 266 RSC.
-
(a) T. Xia, T. Song, G. Zhang, Y. Cui, Y. Yang, Z. Wang and G. Qian, Chem.–Eur. J., 2016, 22, 18429 CrossRef CAS PubMed;
(b) J. Yin, T. Wu, J. Song, Q. Zhang, S. Liu, R. Xu and H. Duan, Chem. Mater., 2011, 23, 4756 CrossRef CAS.
-
(a) T. Cheng, T. Wang, W. Zhu, X. Chen, Y. Yang, Y. Xu and X. Qian, Org. Lett., 2011, 13, 3656 CrossRef CAS PubMed;
(b) Z. Liu, C. Zhang, W. He, Z. Yang, X. Gao and Z. Guo, Chem. Commun., 2010, 46, 6138 RSC;
(c) L. Xu, M. He, H. Yang and X. Qian, Dalton Trans., 2013, 42, 8218 RSC;
(d) H. Un, C. Huang, J. Huang, C. Huang, T. Jia and L. Xu, Chem.–Asian J., 2014, 9, 3397 CrossRef CAS PubMed;
(e) Y. Ren, N. Wu, J. Huang, Z. Xu, D. Sun, C. Wang and L. Xu, Chem. Commun., 2015, 51, 15153 RSC;
(f) Z. Xu, G. Li, Y. Ren, H. Huang, X. Wen, Q. Xu, X. Fan, Z. Huang, J. Huang and L. Xu, Dalton Trans., 2016, 45, 12087 RSC;
(g) C. Huang, L. Xu, J. Zhu, Y. Wang, B. Sun, X. Li and H. Yang, J. Am. Chem. Soc., 2017, 139, 9459 CrossRef CAS PubMed.
-
(a) Y. Cui, Y. Yue, G. Qian and B. Chen, Chem. Rev., 2012, 112, 1126 CrossRef CAS PubMed;
(b) Y. Cui, B. Li, H. He, W. Zhou, B. Chen and G. Qian, Acc. Chem. Res., 2016, 392, 86 Search PubMed.
- J. Hao and B. Yan, J. Mater. Chem. A, 2014, 2, 18018 CAS.
- X. Shi, H. Wang, T. Han, X. Feng, B. Tong, J. Shi, J. Zhi and Y. Dong, J. Mater. Chem., 2012, 22, 19296 RSC.
- Q. Tang, S. Liu, Y. Liu, J. Miao, S. Li, L. Zhang, Z. Shi and Z. Zheng, Inorg. Chem., 2013, 52, 2799 CrossRef CAS PubMed.
- B. Chen, L. Wang, Y. Xiao, F. R. Fronczek, M. Xue, Y. Cui and G. Qian, Angew. Chem., Int. Ed., 2009, 121, 508 CrossRef.
- L. Li, Q. Chen, Z. Niu, X. Zhou, T. Yang and W. Huang, J. Mater. Chem. C, 2016, 4, 1900 RSC.
- X. Xu and B. Yan, J. Mater. Chem. C, 2016, 4, 1543 RSC.
-
(a) J. Hao and B. Yan, Chem. Commun., 2015, 51, 7737 RSC;
(b) C. Liu and B. Yan, J. Colloid Interface Sci., 2015, 459, 206 CrossRef CAS PubMed;
(c) X. Xu and B. Yan, Sens. Actuators, B, 2016, 222, 347 CrossRef CAS.
-
(a) H. Zhu, J. Fan, B. Wang and X. Peng, Chem. Soc. Rev., 2015, 44, 4337 RSC;
(b) H. J. Jung, N. Singh and D. O. Jang, Tetrahedron Lett., 2008, 49, 2960 CrossRef CAS.
- S. M. Cohen, Chem. Rev., 2012, 112, 970 CrossRef CAS PubMed.
-
(a) M. Kandiah, M. H. Nilsen, S. Usseglio, S. Jakobsen, U. Olsbye, M. Tilset, C. Larabi, E. A. Quadrelli, F. Bonino and K. P. Lillerud, Chem. Mater., 2010, 22, 6632 CrossRef CAS;
(b) A. Schaate, P. Roy, A. Godt, J. Lippke, F. Waltz, M. Wiebcke and P. Behrens, Chem.–Eur. J., 2011, 17, 6643 CrossRef CAS PubMed.
-
(a) G. W. Peterson, J. J. Mahle, J. B. Decoste, W. O. Gordon and J. A. Rossin, Angew. Chem., Int. Ed., 2016, 128, 6343 CrossRef;
(b) L. Shen, W. Wu, R. Liang, R. Lin and L. Wu, Nanoscale, 2013, 5, 9374 RSC;
(c) S. S. Nagarkar, T. Saha, A. V. Desai, P. Talukdar and S. K. Ghosh, Sci. Rep., 2014, 4, 7053 CrossRef CAS PubMed.
-
(a) X. Zhang, Q. Hu, T. Xia, J. Zhang, Y. Yang, Y. Cui, B. Chen and G. Qian, ACS Appl. Mater. Interfaces, 2016, 8, 32259 CrossRef CAS PubMed;
(b) J. H. Cavka, S. Jakobsen, U. Olsbye, N. Guillou, C. Lamberti, S. Bordiga and K. P. Lillerud, J. Am. Chem. Soc., 2008, 130, 13850 CrossRef PubMed.
- K. Jiang, L. Zhang, Q. Hu, D. Zhao, T. Xia, W. Lin, Y. Yang, Y. Cui, Y. Yang and G. Qian, J. Mater. Chem. B, 2016, 4, 6398 RSC.
- S. Liu, Z. Xiang, Z. Hu, X. Zheng and D. Cao, J. Mater. Chem., 2011, 21, 6649 RSC.
Footnote |
† Electronic supplementary information (ESI) available. See DOI: 10.1039/c7ra11162b |
|
This journal is © The Royal Society of Chemistry 2017 |