DOI:
10.1039/C7RA11142H
(Paper)
RSC Adv., 2017,
7, 56771-56778
Incorporated α-amylase and starch in an edible chitosan–procyanidin complex film increased the release amount of procyanidins
Received
10th October 2017
, Accepted 25th November 2017
First published on 15th December 2017
Abstract
The inclusion of bioactive substances improved the characteristics of chitosan film or adds new functions to it. Chitosan dissolution is a dilemmatic problem because of the low pH of edible solvents and moderate pH of inedible solvents. Procyanidins, α-amylase and starch were added in the film to enhance the antioxidant ability. Sodium bicarbonate was also employed to adjust the pH of acetic acid solution (solvent). Constituent optimisation of the film-forming solution including the pH of solutions with different amounts of sodium bicarbonate, activity of α-amylase at different pH and addition of appropriate amounts of the three main ingredients was performed. The results showed that the solvent pH should be more than 4. The released amount of reducing sugar (starch hydrolyzate) increased with the increase in the amount of enzyme addition. α-Amylase exhibited a more significant effect than procyanidins, increasing the amount of procyanidins. Full-wavelength scanning, optical test, scanning electron microscopy, X-ray diffraction (XRD), infrared (IR) and differential scanning calorimetry (DSC) were performed to measure the film's characteristics. Even the absorbance peak of procyanidins at 280 nm was increased, following its increasing addition amount; the optical values showed no significant difference. Chitosan film containing starch, α-amylase and procyanidins (CSAPW) had a smoother surface than others. Moreover, the micropores observed on CSAPW can be induced by starch hydrolysis in water immersion. The results of X-ray diffraction (XRD), IR and DSC suggested that water immersion provided the environment for enzymes to hydrolyse the starch. In addition, the hydrolysis function induced the destruction of CSAPW's crystal structure, the change of vibration of hydrogen between chitosan, starch, procyanidins, water and the variation of water evaporation temperature.
1 Introduction
Chitosan is an ideal fresh-keeping material for food due to its characteristics including edibility, nontoxicity, film formation and antimicrobial activities.1–3 Chitosan-incorporated films are attractive for research and applications, such as the shelf-life extension of sliced fresh red meats by low-density polyethylene-incorporated chitosan films,4 maintenance of the visual appearance, taste and even the overall quality of strawberries by chitosan–beeswax films.5 Compounds that can improve films' mechanical or bioactive properties have been used in the preparation and application of the chitosan film to enhance its performance.6,7 Starch, one of the most common foods and an edible ingredient, has also been used in preparing the chitosan film. The starch-incorporated chitosan film acquired preferable elongation at break-point and water vapour transmission rate by the interaction between the hydroxyl groups of starch and the amino groups of chitosan, thus possessing also an outstanding antibacterial activity.8,9 Some antioxidative substances or bioactive compounds such as essential oil, ferulic acid, maqui berry and polyphenols from thyme extracts can be added to the starch-incorporated film to limit its disadvantages.7,10–12 Based on the incorporation methods, sustained-release chitosan film with medicine release or enhanced fresh-keeping ability13–16 can be developed.
Although the chitosan complex film can exhibit multiple functions depending upon the incorporation method, the use of chitosan has been seriously restricted because of its poor solubility. Chitosan can be only dissolved in some specific organic acids and a few inorganic solvents,17 which led to a number of studies on chitosan dissolution. Various aqueous solutions and chitosan modifications were investigated to fix this problem.18,19 However, modification made chitosan inedible, and aqueous solutions were usually alkaline, acidic or toxic. Unstable compounds such as enzyme and certain antioxidants lose their activities in extreme pH.
Procyanidins, present in foods such as apples, berries, cocoa, grapes and wine, are the second most abundant natural phenolic compounds after lignin.20 They exhibit multiple physiological activities, such as antioxidant, antimicrobial, anti-inflammatory, ant-carcinogenic and anti-hypertensive activities, and have been studied extensively in recent years.21–23 In particular, the strong radical scavenging ability and high-antioxidant capacity of procyanidins were determined by in vitro tests;24,25 nevertheless, only oligomeric procyanidins (up to trimeric compounds) were bioavailable and resorbed in the intestine.20
Therefore, procyanidins were added into the chitosan film to endow its antioxidant ability. Starch and α-amylase were also employed in the film preparing process to increase the release rate of procyanidins by starch hydrolysis. Considering the dilemmatic problem that chitosan was dissolved in low pH of an edible solvent (such as acetic acid) and moderate pH of an inedible solvent,26,27 a method was developed to adjust the pH of the chitosan–acetic acid solution and afforded moderate enzyme activity.
2 Materials and methods
2.1 Materials
Medium molecular weight chitosan (CAS, 9012-76-4, deacetylation degree > 90%, Bide Biotechnology Ltd., Shanghai China), procyanidins (CAS, 4852-22-6, Baishun Biotechnology Ltd., Tianjin, China), α-amylase (CAS, 9001-19-8, Puyihua Biotechnology Ltd., Beijing, China), ABTS (CAS, 30931-67-0, Meilunbio Biotechnology Ltd., Dalian, China) and starch (CAS, 9005-25-8, Alading Ltd., Shanghai, China) were used in this study.
2.2 Film formation
2.2.1 Pre-preparation solution. Chitosan (1 g) was dispersed in 30 mL water with an electromagnetic stirrer until the homogeneous chitosan dispersion formed. Acetic acid (10 mL) was mixed with the chitosan solution and shaken for 1 min to dissolve the chitosan until the solution showed a transparent color. Subsequently, 10 mL water, certain volumes (1, 3, 5, 7 and 9 mL) of sodium bicarbonate (0.5 mol L−1) and 1 mL glycerol were blended with the homogenised solution, in sequence, with 5 min stirring intervals. Finally, water was added to obtain a total volume of 100 mL, and the solution was vacuum degassed for five minutes.
2.2.2 Film-forming solution. Pre-preparation solution (30 mL) was mixed with certain volumes (1, 3, 5, 7 and 9 mL) of procyanidin solution (1%), starch (1%) and α-amylase (1%) in sequentially with 5 min stirring intervals following the addition of each solution. After vacuum degassing, film formation was performed following the modified procedures described by Clara Pastor.28 Film was obtained by casting the film-forming solution into a plastic plate (9 cm diameter) and drying at 40 °C for 48 h in the dark.
2.3 Constituent optimisation of film-forming solution
2.3.1 pH change of film-forming solution during drying. Pre-preparation solutions were made following the description in Section 2.2.1; after vacuum degassing, it was dried at 40 °C in a 250 mL beaker. The pH of the solution was measured at residual volumes of 100, 70, 40 and 10 mL (induced by the water evaporation).
2.3.2 Enzyme activity of α-amylase at different pH. Sodium acetate–acetic acid buffer solution and phosphate buffer solution (pH = 3, 4, 5, 6 and 7) containing 1% α-amylase were stored at 40 °C for 24, 48 and 72 h. Up to 1 mL, the abovementioned solution was taken every 24 h to perform the following trial. The enzyme solution (1 mL) was mixed with equal volumes of starch (1%) and kept at 60 °C for 10 min; after cooling, 1 mL 3,5-dinitrosalicylic acid was added and the absorbance was measured by the 3,5-dinitrosalicylic acid assay.29 The absorbance representing the concentration of reduced sugar was used to evaluate the enzyme activity of hydrolysis.
2.3.3 Optimisation of starch content. Pre-preparation solutions (30 mL) containing 2 mL α-amylase (1%) were each mixed with 0, 1, 2, 3, 4 and 5 mL starch solution (1%). After drying for 24 h at 40 °C, the film was taken and submerged in 30 mL water; then, 1 mL solution was taken at 1, 3 and 5 days and blended with 0.5 mL 3,5-dinitrosalicylic acid. Absorbance detection using the 3,5-dinitrosalicylic acid assay was performed at last. The absorbance representing the concentration of reduced sugar was used to evaluate the hydrolysis degree of starch.
2.3.4 Standard curve of procyanidins. The absorbance of different concentrations of procyanidins solutions (dissolved in water) was determined by a spectrophotometer at 280 nm to establish a standard curve.30
2.3.5 Determination of procyanidins and α-amylase content. Pre-preparation solution (30 mL) mixed with 1, 2, 3, 4 and 5 mL of procyanidin solution (1%), 4 mL starch (1%) and 2 mL α-amylase (1%) was used to investigate the optimum addition volume of procyanidins in film. The same volume of the pre-preparation solution was mixed with 0, 1, 2, 3, 4 and 5 mL of α-amylase (1%), 4 mL starch (1%) and 4 mL procyanidin solution (1%) in the optimisation trial of the α-amylase addition amount. After drying for 48 h at 40 °C (following the description in Section 2.2.2), the film was taken and submerged in 30 mL water. Lixivium was taken at 1 and 7 days later and procyanidin content was detected. Films, along with the lixivium, were boiled at 95 °C for 15 min to determine their release amount under high temperature.
2.4 Optical properties
2.4.1 Colour coordinate. Colourimetry (CM-3600A, Konica Minolta, Japan) and ultraviolet-visible spectrometry (UV-2102PCS, Unico Instrument Ltd., China) were employed to determine the optical properties of the film. The central area and the four areas around it were selected in this trial, and the total colour difference (ΔE) was calculated as follows:31
2.4.2 Full-wavelength scanning. The films were cut to fit the cuvette and attached on the inner side near the light source (blanking with empty cuvette), and absorbance was recorded at every 12 nm wavelength interval from 200 nm to 992 nm.
2.5 Scanning electron microscopy
Four types of films were detected in this trial. The first contained chitosan starch, α-amylase and procyanidins (CSAP). The second was formed by chitosan, starch and procyanidins (CSP). The third was made by chitosan, starch, α-amylase and procyanidins and immersed in water for 7 days (CSAPW), and the last film constituted chitosan, starch, and procyanidins and immersed in water for 7 days (CSPW). Scanning electron microscopy (SEM) was applied in all these films.
2.6 X-ray diffraction detection
Pre-preparation solution was dried to prepare the chitosan film, the chitosan starch film containing starch (CS) and the chitosan–starch–α-amylase film (CSA) produced using starch and α-amylase. Chitosan, starch, α-amylase, procyanidins, chitosan film, CS, CSA, CSAP, CSP, CSAPW and CSPW were used in the XRD measurements.
The samples were investigated with an X-ray diffractometer (D8-ADVANCE, Bruker AXS, Germany) equipped with a copper tube operated at 35 kV and 30 mA with Cu radiation of 0.154 nm wavelength. Diffractograms were obtained by scanning from 3° to 50° at a rate of 0.5° min−1 with a step size of 0.02.32
2.7 Film stability determination by Fourier transform infrared spectroscopy
The samples investigated via XRD were also investigated by Fourier transform infrared spectroscopy (FT-IR). The FT-IR spectra of the samples were recorded using a Nicolet 5700 spectrophotometer (Thermo Nicolet 5700, USA). The powdered samples were mixed separately with an analytical grade KBr and then pressed onto discs. The spectra of the samples were recorded in the region of 4000–400 cm−1, with a total of 32 scans.33
2.8 Differential scanning calorimetry analysis
The samples studied via SEM were used to investigate the thermodynamic property by differential scanning calorimetry (DSC) in a TA Q100-DSC (USA) calorimeter. The films (100 mg) were weighted and heated from 10 °C to 140 °C at 10 °C min−1 under nitrogen atmosphere;34 an empty pan was used to adjust the TA Q100-DSC (USA) calorimeter.
3 Results and discussion
3.1 Optimisation of film-forming solution constituent
Following the increase of sodium bicarbonate, the pH of film-forming solutions rose at a range from 4.15 to 4.45 at the beginning (100 mL residual volume). The maximum pH range was from 5.01 to 5.71 at the end of drying (10 mL residual volume) (Fig. 1A). Acetic acid volatilisation and the increased concentration of sodium acetate accompanying the water evaporation elevated the solution pH. The low pH (pH = 3) significantly inhibited the enzyme activity at the chasing days with minimal difference between 24, 48 and 72 h storage. After 24 h storage, the enzyme activity displayed the highest value at pH 5 and showed a decreased value at pH 7. No significant difference was observed between the enzyme activity at pH = 4, 5, 6 and 7 (Fig. 1B).
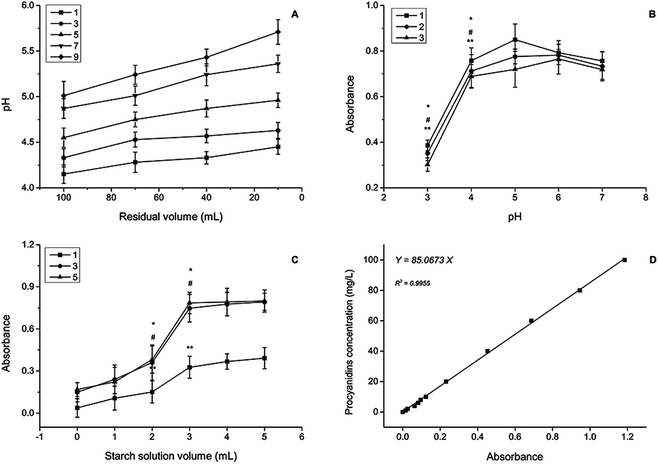 |
| Fig. 1 Constituent optimization of film-forming solution. (A) pH change of film-forming solution in the drying process. Lines of 1, 3, 5, 7 and 9 are the addition volume of sodium bicarbonate solution (mL). (B) Enzyme activity of α-amylase at different pH and storage time. Lines of 1, 2 and 3 are the film immersion time (days). (C) Optimization of starch content. Lines of 1, 3 and 5 are the film immersion time (days). (D) Standard curve of procyanidins. Same symbol represents significantly different values at p ≤ 0.05. | |
Starch solution with 3 mL addition in all of the three chasing days showed remarkable increase of reducing sugar content (Fig. 1C). Long-time immersion did not benefit the release of reducing sugar (starch hydrolysis) accounting for the amount of reducing sugar at 3 days approximated at 5 days, specifying that α-amylase presented its function within 3 days. Enzyme as a type of protein containing hydroxyls, amino, and carboxyl can be immobilised by the interlinkage between the polar groups of chitosan and enzyme.35,36 Interlinkage between starch and protein also played an important part in restricting the movement of starch.37
Procyanidin solutions (concentration range from 0 mg L−1 to 100 mg L−1) were determined by a spectrophotometer and the standard curve was established with R2 = 0.9955 (Fig. 1D). The film with more procyanidins maintained a high release amount, and no significant difference was observed between the procyanidin release amount at 1 and 7 days later for the groups with the same volume of procyanidin addition (Fig. 2A). In general, films with high procyanidin addition released more procyanidins with 3 mL as the turning point.
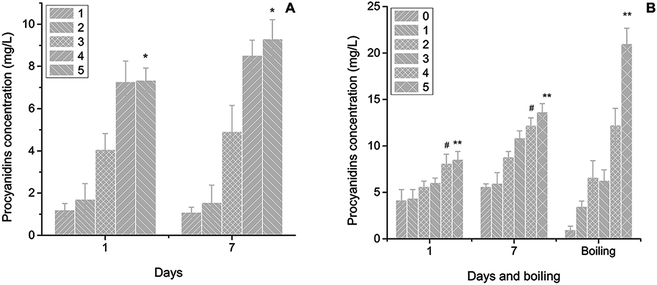 |
| Fig. 2 Determination of procyanidins and α-amylase content. (A) Determination of the addition amount of procyanidins solution. Lines of 1, 2, 3, 4 and 5 are volume of procyanidins solution (mL). (B) Determination of the addition amount of α-amylase solution. Lines of 1, 2, 3, 4 and 5 are volume of α-amylase solution (mL). Same symbol represents significantly different values at p ≤ 0.05. | |
More procyanidins release was observed at the 7 day group when the α-amylase addition amount was more than 3 mL (Fig. 2B). Starch hydrolysis destroyed the chainlike structure of starch and the network formed by the linkage between chitosan and starch facilitated the escape of procyanidins. Boiling enhanced the hydrolysis near the optimum temperature of α-amylase at the 5 mL addition of procyanidins solution. Other groups demonstrated a decreased release amount. Procyanidins including polymers (four or more catechines) representing a group of condensed flavan-3-ols can be destroyed under high temperature.38–40
3.2 Optical properties
The absorption peaks of five films located approximately from 250 nm to 400 nm were elevated following the increase of procyanidin addition, indicating the absorption peak of procyanidins (Fig. 3A).30 The peaks identified near 450 nm could represent another type of procyanidins, such as the procyanidins B2.41 The values of ΔE for five films decreased following the increase in procyanidin addition with the same trends as L* that represented the colour lightness, suggesting that procyanidins decreased the film transparency (Fig. 3B).42 The colour parameter of a*, which is the index of the red and green, indicated that the procyanidins show their red colour in films and a strong red colour was found in high procyanidin addition.43
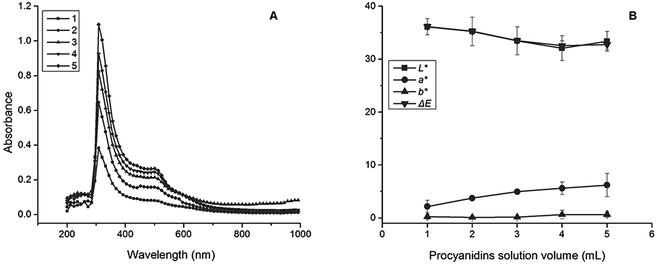 |
| Fig. 3 Optical properties of films. (A) Colour coordinate. Lines of 1, 2, 3, 4 and 5 are volume of procyanidins solution (mL). (B) Full-wavelength scanning. | |
3.3 Morphology of films
Cracks formed by drying occurred in CSAP and CSP films; wide cracks were observed on CSP and thin cracks on CSAP (arrowheads in CSP and CSAP of Fig. 4). Starch hydrolysed by α-amylase in film drying produced monosaccharides, oligosaccharides and polysaccharides that probably formed a tighter link than the starch. Enzyme as a type of protein could contribute to the tensile strength (elasticity) and reduce the breaking of film.44 The starch–chitosan film did not have better mechanical properties than this chitosan film; thus, the starch containing the amylopectin and amylose exhibited no positive effect on protecting the breaking of the film.45 CSAPW had a smooth surface and micropores on it; moreover, starch hydrolysis on immersion in water destroyed the film surface and produced the pores (arrowheads in CSAPW of Fig. 4). A rough surface with bubble shaped bulges was observed, suggesting that the destruction of the film surface via hydrolysis allowed the escape of air or carbon dioxide on immersion in water (arrowheads in CSPW of Fig. 4). Clearly, the hydrolysis function also contributed to the escape of ingredients in film, such as the saccharide produced by starch hydrolysis and the procyanidins.
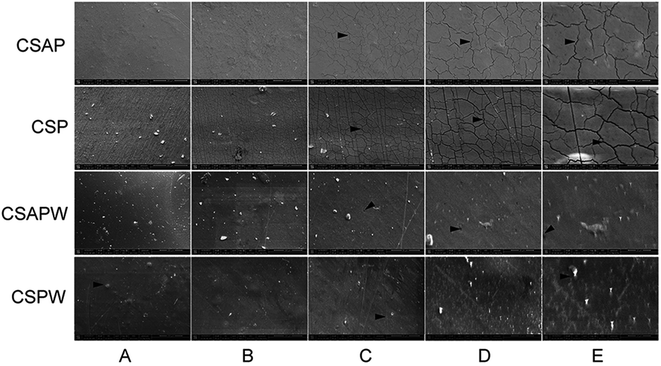 |
| Fig. 4 Morphologies of films observed using SEM. Pictures of the first line are the morphologies of CSAP (film containing chitosan starch, α-amylase and procyanidins). Pictures of the second line are the morphologies CSP (film containing by chitosan, starch and procyanidins). Pictures of the third line are the morphologies of CSAPW (film containing chitosan, starch, α-amylase and procyanidins, and it was immersed in water for 7 days). Pictures of the third line are the morphologies of CSPW (film containing chitosan, starch and α-amylase, and it was immersed in water for 7 days). The first, second, and third rows are pictures with 1000, 2000, and 4000 times magnification. | |
3.4 XRD detection
The rice starch powder had a typical diffraction pattern with strong reflection at four peaks with 2θ in the range of 16–24 (Fig. 5A). Chitosan powder was in a crystalline state because two main diffraction peaks at 2θ of approximately 12–20 and the peak at 2θ of approximately 20 of disappeared and peaks at 2θ of approximately 12 still existed with less intensity.37 CS and chitosan film subtracting the crystalline state of starch possessed similar peaks at 2θ of approximately 20, and the addition of starch increased the crystal intensity. These results demonstrated that the interlinkage between chitosan and starch showed a positive effect on maintaining the crystal structure of chitosan and its film.
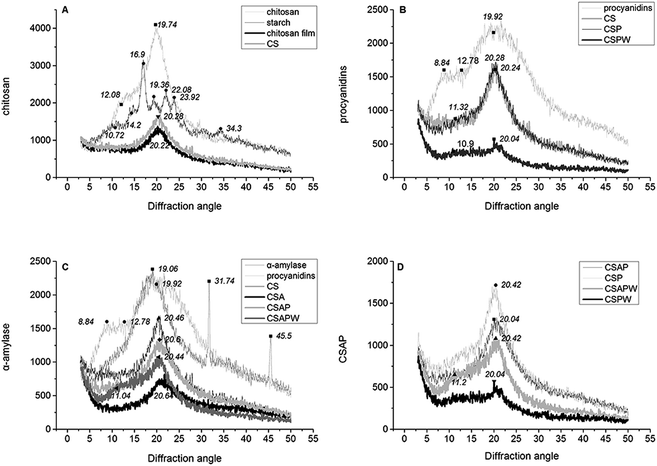 |
| Fig. 5 XRD detection with combination of ingredients and films. | |
Procyanidins with visible peaks at 2θ of 19.92 and a step-like structure peak at 2θ in the range of 8.84–12.78 of induced a slight change of the CS crystal structure at 2θ of approximately 11 (CSP and CSPW of Fig. 5B). Water immersion and dissolution of the procyanidins destroyed the crystal structure of the CSP film, which caused a strong decrease of CS typical peak at 2θ = 20 (CSPW of Fig. 5B). Water immersion resulted in the hydrolysis of starch and the release of procyanidins, which induced CSAP decrease (CSAPW of Fig. 5C). CSAPW and CSPW had relatively higher crystal intensity than the other two lines at 2θ of approximately 11, and water also induced a significantly higher peak in CSAPW than others by releasing the starch and its hydrolysate (CSPW and CSAPW of Fig. 5D).
3.5 Film stability determination by FT-IR spectra
Absorption bands at approximately 1037 cm−1 (C–O stretching) and 1153 cm−1 (asymmetric stretching of C–O–C bridge and glycosidic linkage) were characteristics of its saccharide structure (Fig. 6A).43 The peaks at 1411, 1566 and 2929 cm−1 were assigned to the C–O stretching of the C–O–H stretching of the primary alcoholic group (–CH2OH), the C–H bending of –CH2 of the pyranose ring and N–H bending in the primary amine groups (–NH2/–NH3+), respectively.12,43,46 The bands at 1657 and 2929 cm−1 were attributed to C
O stretching (amide) and the C–H stretching related with the ring methane hydrogen atoms.47 The broad band occurring at 3356 cm−1 was attributed to the vibration stretching of bound O–H. The shift of chitosan and starch peaks at 3404 cm−1 to 3356 cm−1, and the change of 1656 cm−1 to 1567 cm−1 indicated the strong hydrogen bonding between the chitosan chain and chitosan starch. Procyanidins addition improved the vibration modes of CSAP to 1412, 1567 and 3325 cm−1 (Fig. 6B) as well as that of CSAPW, illustrating that procyanidins and polysaccharide were released in the water immersion.
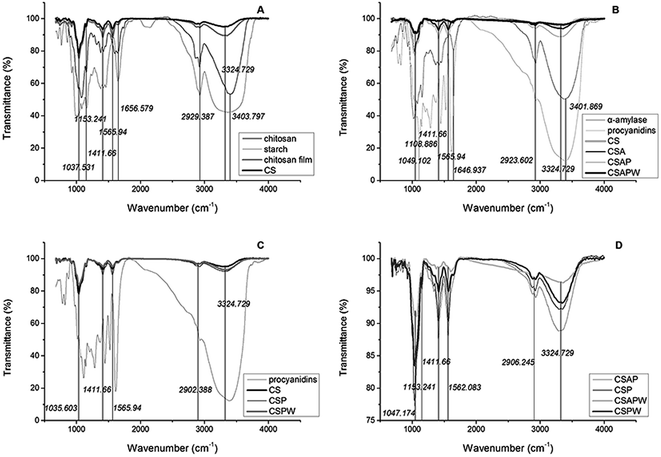 |
| Fig. 6 Stability determination of combination of ingredients and films by FT-IR spectra. | |
The vibrational stretching of O–H bond in CS was weaker than that of other groups, illustrating that the hydrogen bond between O–H and –NH2 or O–H and O–H was rigid and restricted the vibrational stretching of O–H (Fig. 6C). Procyanidins enhanced the intensity of O–H by interlinking the O–H of the chitosan and phenolic hydroxyl groups, and the dissolution of procyanidins led to the decrease in the intensity of –CH2OH at 1411 cm−1 and N–H at 1566 cm−1 by destroying the hydrogen bonds (CSPW of Fig. 6C). Stretching of C–O at 1047 cm−1, C–O–H of –CH2OH at 1411 cm−1, N–H at 1562, O–H at 3325 cm−1 and N–H bending in the primary amine groups (–NH2/–NH3+) at 2906 cm−1 was significantly decreased by the α-amylase. Water immersion plays an important role in this process by dissolving the procyanidins and saccharides (CSAPW and CSPW, Fig. 6D). Procyanidins and α-amylase also enhanced the hydrogen link of O–H at 3325 and N–H bending in the primary amine groups (–NH2/–NH3+) at 2906 cm−1, resulting in high intensity at those groups (Fig. 6D).
3.6 Differential scanning calorimetry analysis
The peaks' shapes in CSPW and CSAP were similar, and a sharper exothermic process was observed in CSAPW than the other three curves (Fig. 7). The decrease of four lines approximately at 100 °C was due to water evaporation.12 Starch hydrolysis and water immersion facilitated the escape of the hydrolyzate of starch and the procyanidins, leading to more space and binding sites of hydrogen bonds for water. This phenomenon resulted in more water permeating into CSAPW and a large area of peak observed in it. Starch hydrolysis in drying of CSAP and dissolution of starch and procyanidins of CSPW in water immersion also had the same positive effect on increasing the hydrogen bonds for restricting the water in films. Therefore, the areas of CSAP and CSPW were larger than that in CSP (Fig. 7).
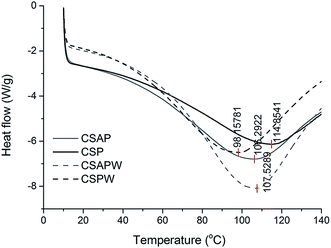 |
| Fig. 7 DSC analysis of the four films. | |
CSP with the highest water evaporation temperature exhibited the strongest water binding capacity by the linkage between the O–H, –NH2 and phenolic hydroxyl groups. Moreover, the small space resulted in more combining sites for water molecule and restricted the water evaporation. CSPW had the lowest evaporation temperature at approximately 98.2 °C. The sites of hydrogen bonds in CSAPW and CSAP exposed by the hydrolysis of α-amylase exhibited strong ability in binding the water molecule than CSPW.
4 Conclusions
The film-forming solutions obtained the lowest pH = 4.15 with 1 mL sodium bicarbonate at the beginning of drying, and the pH increased according to an approximate linear shape. α-Amylase activity maintained a relatively high level when the pH of the solvent was more than 4. Moreover, 3 day storage showed no visible effect on decreasing the activity. The trend of procyanidin release amount generally improved by the increase in amount of procyanidin addition. However, the increase of α-amylase addition can improve the release amount of procyanidins, particularly on addition of appropriate amount of α-amylase (more than 3 mL). The values of a* and b* showed the rising trend, and the L* and ΔE declined along with the increasing amount of procyanidin addition.
Micropores observed on CSAPW can be induced by starch hydrolysis in water immersion, which destroyed the film surface and produced the pores. Water immersion decreased the crystal texture of CSAPW and CSPW by dissolving the procyanidins, starch and starch hydrolysis. It also decreased the vibration of hydrogen bonds and its related groups by starch hydrolysis. CSPW maintained the lowest evaporation temperature at approximately 98.2 °C, and water evaporation temperatures of CSAPW and CSAP were lower than CSP.
Conflicts of interest
There are no conflicts to declare.
Acknowledgements
This work was supported by the Shandong Provincial Natural Science Foundation, China (ZR2017MC073).
References
- X. C. Jia, S. Lai and H. Yang, Food Control, 2015, 53, 195–205 CrossRef.
- Z. Song, F. Li, H. Guan, Y. Xu, Q. Fu and D. Li, Food Control, 2017, 74, 34–44 CrossRef CAS.
- A. M. Durango, N. F. F. Soares and N. J. Andrade, Food Control, 2006, 17, 336–341 CrossRef CAS.
- P. Suil, M. Kenneths and D. Paul, Meat Sci., 2010, 85, 493 CrossRef PubMed.
- E. Velickova, E. Winkelhausen, S. Kuzmanova, V. D. Alves and M. Moldão-Martins, LWT–Food Sci. Technol., 2013, 52, 80–92 CrossRef CAS.
- C. Xiao, L. Zhu, W. Luo, X. Song and Y. Deng, Food Chem., 2010, 121, 1003–1009 CrossRef CAS.
- E. Genskowsky, L. A. Puente, J. A. Pérez-Álvarez, J. Fernandez-Lopez, L. A. Muñoz and M. Viuda-Martos, LWT–Food Sci. Technol., 2015, 64, 1057–1062 CrossRef CAS.
- F. Liu, B. Qin, L. He and R. Song, Carbohydr. Polym., 2009, 78, 146–150 CrossRef CAS.
- X. L. Shen, J. M. Wu, Y. Chen and G. Zhao, Food Hydrocolloids, 2010, 24, 285–290 CrossRef CAS.
- F. M. Pelissari, M. V. Grossmann, F. Yamashita and E. A. Pineda, J. Agric. Food Chem., 2009, 57, 7499–7504 CrossRef CAS PubMed.
- E. Talón, K. T. Trifkovic, V. A. Nedovic, B. M. Bugarski, M. Vargas, A. Chiralt and C. González-Martínez, Carbohydr. Polym., 2017, 157, 1153 CrossRef PubMed.
- S. Mathew and T. E. Abraham, Food Hydrocolloids, 2008, 22, 826–835 CrossRef CAS.
- H. R. Zhao, K. Wang, Y. Zhao and L. Q. Pan, Biomaterials, 2002, 23, 4459 CrossRef CAS PubMed.
- B. Blanco-Fernandez, M. I. Rial-Hermida, C. Alvarez-Lorenzo and A. Concheiro, J. Appl. Polym. Sci., 2013, 129, 626–635 CrossRef CAS.
- X. Z. Shu and K. J. Zhu, Int. J. Pharm., 2000, 201, 51–58 CrossRef CAS PubMed.
- V. Chandrasekar, J. N. Coupland and R. C. Anantheswaran, J. Food Sci., 2016, 81, E2503 CrossRef CAS PubMed.
- M. Fan and Q. Hu, Carbohydr. Res., 2009, 344, 944–947 CrossRef CAS PubMed.
- C. Li, Q. Han, Y. Guan and Y. Zhang, Soft Matter, 2014, 10, 8245 RSC.
- X. Jiang, Y. Zhao and L. Hou, Carbohydr. Polym., 2016, 135, 191–198 CrossRef CAS PubMed.
- N. Köhler, V. Wray and P. Winterhalter, J. Chromatogr. A, 2008, 1177, 114–125 CrossRef PubMed.
- A. Alejo-Armijo, N. Glibota, M. P. Frías, J. Altarejos, A. Gálvez, E. Ortega-Morente and S. Salido, Int. J. Food Sci. Technol., 2016, 52, 679 CrossRef.
- M. J. Bak, V. L. Truong, H. S. Kang, M. Jun and W. S. Jeong, Oxid. Med. Cell. Longevity, 2013, 2013, 409321 Search PubMed.
- Z. P. Vila, L. Guerrero, M. Margalef, M. Quiñones, L. Arola, A. Arola-Arnal and B. Muguerza, Antihypertensive
Effect and Hepatic Lipid Improvement of Grape Seed Procyanidins in Cafeteria-Diet Rats, World Forum of Nutrition, At Reus, Tarragona, Spain, 2013, vol. 62(2), pp. 1–74 Search PubMed.
- A. M. Mendoza-Wilson, S. I. Castro-Arredondo and R. R. Balandrán-Quintana, Food Chem., 2014, 161, 155–161 CrossRef CAS PubMed.
- H. Falleh, S. Oueslati, S. Guyot, A. B. Dali, C. Magné, C. Abdelly and R. Ksouri, Food Chem., 2011, 127, 1732–1738 CrossRef CAS.
- H. Xie, S. Zhang and S. Li, Green Chem., 2006, 8, 630–633 RSC.
- M. Zeng, Z. Fang and C. Xu, J. Membr. Sci., 2004, 230, 175–181 CrossRef CAS.
- C. Pastor, L. Sánchez-González, A. Chiralt, M. Cháfer and C. González-Martínez, Food Hydrocolloids, 2013, 30, 272–280 CrossRef CAS.
- R. B. Toma and H. K. Leung, Food Chem., 1987, 23, 29–33 CrossRef CAS.
- L. J. Porter, L. N. Hrstich and B. G. Chan, Phytochemistry, 1985, 25, 223–230 CrossRef.
- H. J. Yang, J. H. Lee, M. Won and K. B. Song, Food Chem., 2016, 196, 174–179 CrossRef CAS PubMed.
- W. Shujun, G. Wenyuan, L. Hongyan, C. Haixia, Y. Jiugao and X. Peigen, Food Chem., 2006, 99, 38–44 CrossRef.
- A. Zarski, S. Ptak, P. Siemion and J. Kapusniak, Carbohydr. Polym., 2016, 137, 657–663 CrossRef CAS PubMed.
- O. Lopez, M. A. Garcia, M. A. Villar, A. Gentili, M. S. Rodriguez and L. Albertengo, LWT–Food Sci. Technol., 2014, 57, 106–115 CrossRef CAS.
- E. Taqieddin and M. Amiji, Biomaterials, 2004, 25, 1937–1945 CrossRef CAS PubMed.
- J. Zhu, Z. Zhu, Z. Lai, R. Wang, X. Guo, X. Wu, G. Zhang, Z. Zhang, Y. Wang and Z. Chen, Sensors, 2002, 2, 127–136 CrossRef CAS.
- T. Bourtoom and M. S. Chinnan, LWT–Food Sci. Technol., 2008, 41, 1633–1641 CrossRef CAS.
- S. Isabel, B. Sun, M. Anam, F. Vítorde and R. S. Jorgem, Food Chem., 2008, 108, 519–532 CrossRef PubMed.
- M. Jerez, A. Selga, J. Sineiro, J. L. Torres and M. J. Nunez, Food Chem., 2007, 100, 439–444 CrossRef CAS.
- D. K. Asami, Y. J. Hong, D. M. Barrett and A. E. Mitchell, J. Sci. Food Agric., 2003, 83, 56–63 CrossRef CAS.
- V. Glavnik, B. Simonovska and I. Vovk, J. Planar Chromatogr.--Mod. TLC, 2010, 23, 230–232 CrossRef CAS.
- J. Mei, Y. Yuan, Y. Wu and Y. Li, Int. J. Biol. Macromol., 2013, 57, 17–21 CrossRef CAS PubMed.
- A. Silva-Weiss, V. Bifani, M. Ihl, P. J. A. Sobral and M. C. Gómez-Guillén, Food Hydrocolloids, 2013, 31, 458–466 CrossRef CAS.
- L. E. Abugoch, C. Tapia, M. C. Villamán, M. Yazdani-Pedram and M. Díaz-Dosque, Food Hydrocolloids, 2011, 25, 879–886 CrossRef CAS.
- M. A. Khan, M. A. Rahman, R. A. Khan, N. Rahman, J. M. M. Islam, R. Alam and M. I. H. Mondal, Polym.-Plast. Technol. Eng., 2010, 49, 748–756 CrossRef CAS.
- A. Sionkowska, A. Planecka, J. Kozlowska, J. Skopinska-Wisniewska and P. Los, Carbohydr. Polym., 2011, 84, 900–906 CrossRef CAS.
- Y. Zhong, X. Song and Y. Li, Carbohydr. Polym., 2011, 84, 335–342 CrossRef CAS.
|
This journal is © The Royal Society of Chemistry 2017 |