DOI:
10.1039/C7RA11086C
(Paper)
RSC Adv., 2017,
7, 55296-55300
A mitochondria-targeting ratiometric fluorescent probe for the detection of hypochlorite based on the FRET strategy†
Received
9th October 2017
, Accepted 29th November 2017
First published on 5th December 2017
Abstract
In this study, a ratiometric fluorescent probe (IRP) for −OCl was designed based on the fluorescence resonance energy transfer (FRET) platform. The probe was fabricated by integrating an imidazo[1,5-a]pyridine moiety (donor) with a rhodamine moiety (acceptor). We evaluated its properties for recognizing −OCl and the results implied that IRP possessed high selectivity, a real-time detection possibility and brilliant photostability. The fluorescence intensity ratios (I575/I467) of IRP displayed desirable −OCl-dependent performance and responded linearly to −OCl in the concentration range of 0.5–3.5 μM. Also, IRP was applied to image endogenous −OCl with a mitochondria-targeting ability, due to the introduction of the quaternized pyridine moiety. Therefore, IRP may be used for further mitochondrial −OCl-related studies.
Introduction
Reactive oxygen species (ROS) are of scientific interest since they could mediate a number of biological processes. Hypochlorous acid (HOCl)/hypochlorite (−OCl), one of the most important ROS, has received increasing attention recently. −OCl is synthesized from hydrogen peroxide and chloride with the aid of myeloperoxidase (MPO) in immunological cells.1 −OCl is a powerful oxidizer with effective antibacterial properties when microorganism infections occur.2 Despite that, the abnormal generation of −OCl in vivo may be harmful and is implicated in various diseases such as rheumatoid arthritis, atherosclerosis, osteoarthritis and lung injury.3–6 Thus, scientists have devoted effort to developing sensitive and accurate methods to detect −OCl. Among the analytical methods, fluorescent probes show many advantages in terms of sensitivity and selectivity as well as spectral resolution.7–18 Single emission-based probes are flawed in quantitative assays, because the results may be perturbed by environmental factors. Ratiometric probes, which can normalize the interference, have been a powerful approach for recognizing and detecting −OCl.19,20
Fluorescence resonance energy transfer (FRET) strategy is an effective approach to constructing ratiometric probes. FRET-based probes for −OCl display two well-resolved emission peaks before and after reaction with −OCl.21–23 However, despite the good performance, it is difficult to construct such an FRET-based system on account of the prerequisite of substantial spectral overlap between donor emission and acceptor absorption. Hence, it is highly desirable to develop novel fluorophores for the fabrication of FRET-based probes.
By far, clarifying the intracellular functions of −OCl is still a challenge since the subcellular distribution of −OCl is uncertain. So it is highly urgent to fabricate fluorescent probes for −OCl possessing organelle-targeting ability. To the best of our knowledge, some organelle-targeting probes for −OCl have been developed.24–28 However, −OCl ratiometric probes based on FRET that can be applied especially in mitochondria are still in great demand.23,29
Previously we designed a ratiometric probe for sensing copper ion based on imidazo[1,5-a]pyridine-rhodamine FRET system.30 The data indicated that emission spectrum of imidazo[1,5-a]pyridine and absorption spectrum of rhodamine largely overlapped, thus giving efficient FRET process. Inspired by this, we chose imidazo[1,5-a]pyridine as FRET donor and rhodamine as FRET acceptor to produce a −OCl ratiometric probe (IRP). IRP exhibited high selectivity and sensitivity toward −OCl. In comparison with other −OCl probes, probe IRP showed much lower detection limit (10.2 nM).31–34 Moreover, quaternized pyridine moiety, which was a mitochondria-targeting group, was introduced into the probe to achieve mitochondria-targeting ability.6,23,29 IRP has also been applied for fluorescence imaging of endogenous −OCl with excellent membrane permeability and stability.
Results and discussion
Design and synthesis of probe IRP
The imidazo[1,5-a]pyridine fluorophore was chosen as the FRET donor due to its outstanding spectroscopic properties.35–37 And rhodamine moiety was selected as FRET acceptor, because the emission spectra of imidazo[1,5-a]pyridine and the excitation spectra of rhodamine largely overlapped, which was essential for an efficient energy transfer process. Importantly, the quaternized pyridine moiety was introduced into probe IRP to realize the mitochondria-targeting capability. We expected that the quaternized pyridine moiety in probe IRP would enable the probe to be suitable for the fluorescence imaging of endogenous −OCl in mitochondria.
Probe IRP was synthesized via a simple process (Scheme 1) and characterized by 1H NMR, 13C NMR and HRMS (Fig. S1–S3, ESI†).
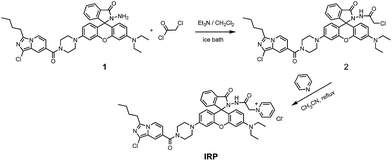 |
| Scheme 1 Synthesis of probe IRP. | |
Spectroscopic response of probe IRP to −OCl
The absorption spectra of IRP were investigated in EtOH-PBS (3/7, v/v, pH 7.4). As shown in Fig. 1, IRP exhibited a maximal absorption band at 365 nm, which was assigned to the imidazo[1,5-a]pyridine moiety. And the absorbance was almost unchanged along with −OCl addition. Upon addition of −OCl, a new absorption peak appeared at 555 nm and the absorbance increased remarkably. The spectra changes could be attributed to the structural formation of ring-opened form of rhodamine moiety, induced by the reaction of IRP and −OCl.
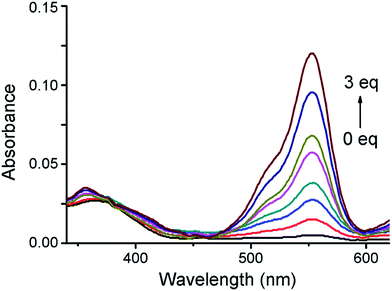 |
| Fig. 1 Absorption spectra of probe IRP (5 μM) upon addition of −OCl. | |
To explore the fluorescence response of IRP to −OCl, probe IRP was titrated with various concentrations of −OCl (Fig. 2a). Upon excitation at 370 nm, the probe only emitted the fluorescence of imidazo[1,5-a]pyridine moiety (467 nm). When −OCl was titrated from 0 to 5 μM, the emission peak of imidazo[1,5-a]pyridine decreased. Meanwhile, a typical emission peak of rhodamine moiety emerged at 575 nm and the fluorescence intensity increased gradually, which was in perfect agreement with the absorption spectra changes. The phenomena could be ascribed to the energy transfer between imidazo[1,5-a]pyridine donor and rhodamine acceptor (Scheme 2). The rhodamine moiety was in the ring-closed form in the absence of −OCl, and no FRET was found in probe IRP at this stage. However, the titration of −OCl resulted in the rhodamine structural transformation from spirolactam form (non-fluorescent) to ring-opened form (fluorescent). The HRMS spectra of the reaction mixture of IRP with −OCl (Fig. S4†) verified the structure change of rhodamine moiety. And the ring-opened rhodamine moiety was an efficient energy acceptor. FRET process between imidazo[1,5-a]pyridine donor and rhodamine acceptor occurred accordingly. The energy transfer efficiency was determined to be 45%.
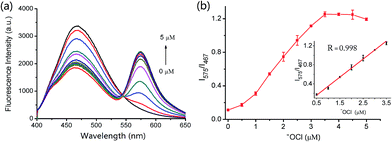 |
| Fig. 2 (a) Fluorescence titration spectra and (b) fluorescence intensity ratios (I575/I467) of probe IRP (2 μM) upon addition of −OCl. The inset showed the linear relationship of intensity ratios (I575/I467) along with −OCl concentration (R = 0.998). | |
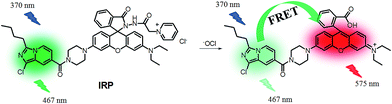 |
| Scheme 2 Proposed mechanism of IRP for sensing −OCl. | |
Fluorescence intensity ratios (I575/I467) as a function of −OCl concentration were illustrated in Fig. 2b. When the −OCl concentration increased from 0 to 5 μM, I575/I467 exhibited a significant enhancement. More importantly, the inset indicated that there was a linear correlation between the fluorescence intensity ratio (I575/I467) and the −OCl concentration from 0.5 to 3.5 μM (R = 0.998). In addition, the limit of detection was calculated to be 10.2 nM, which demonstrated that probe IRP may be able to monitor −OCl changes at trace levels.
In order to investigate whether the probe could selectively respond to −OCl, we evaluated the influence of potential interferents, including ROS/RNS and common ions. As shown in Fig. 3, compared to other tested analytes, only the presence of −OCl caused a dramatic increase of I575/I467 for the probe IRP. The results indicated that the probe possessed excellent selectivity for −OCl even in the complicated intracellular environment.
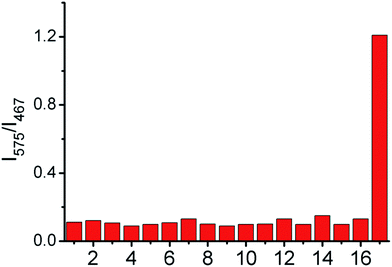 |
| Fig. 3 Fluorescence intensity ratios (I575/I467) of probe IRP (2 μM) containing other ROS/RNS, ions and −OCl. (1): blank, (2): H2O2, (3): t-BuOOH, (4): t-BuO˙, (5): 1O2, (6): −O2, (7): ON, (8): ˙OH, (9): ONOO−, (10): NO2−, (11): AcO−, (12): Br−, (13): Cl−, (14): I−, (15): CO32−, (16): SO42−, (17): −OCl. Concentration: 40 μM for (2)–(9); 4 μM for (17), 1 mM for others. | |
The effect of solution pH on the fluorescence response of IRP toward −OCl was also studied (Fig. 4). Probe IRP itself was stable in the pH range of 4.0–10.0. After −OCl (2 μM) was added to the IRP solution, the fluorescence intensity ratio (I575/I467) increased notably and peaked at pH 7.4, implying that IRP could be used to detect −OCl in neutral and slightly basic conditions. Since the pKa of HOCl was 7.6, we speculated that the probe could sense −OCl rather than HOCl. In consideration of the weakly basic pH conditions in mitochondria, we anticipated that IRP may be suitable for mitochondrial −OCl measurement.
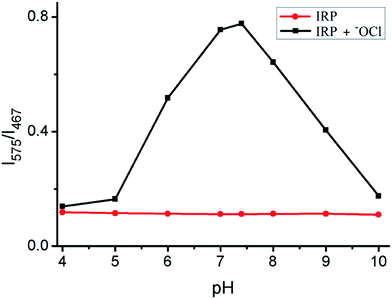 |
| Fig. 4 Fluorescence intensity ratios (I575/I467) of IRP (2 μM) versus pH in the absence (●) or presence (■) of −OCl (2 μM). | |
Furthermore, the time courses of IRP were recorded upon addition of −OCl (3 μM). As displayed in Fig. S5,† the fluorescence intensity ratios (I575/I467) of IRP instantly increased and reached a plateau after −OCl was added to the solution. Hence, the probe was able to sense −OCl rapidly, which was pivotal for the real-time detection of −OCl. The high specificity of IRP for −OCl over other species, fast response, as well as the satisfactory −OCl-dependent performance further confirmed that probe IRP had great potential as a practical tool for −OCl detection in vivo.
Cell imaging of probe IRP
In light of the excellent spectroscopic response of probe IRP toward −OCl in vitro, we proceeded to explore the biological application of cell imaging. Murine RAW264.7 cells were selected to image endogenous HOCl in living cells, since RAW264.7 cells would produce HOCl after the stimulation with lipopolysaccharide (LPS).23 After RAW264.7 cells were incubated with IRP (1 μM) for 30 min, bright blue fluorescence and weak red fluorescence were noticed, as shown in Fig. 5a. Whereas in the experiment group, RAW264.7 cells were incubated with LPS for 6 h and then with phorbol 12-myristate 13-acetate (PMA) for 30 min, followed by incubation with probe IRP (1 μM) for 30 min. According to the previous papers, the synergistic effect of LPS and PMA could stimulate RAW264.7 cells to produce HOCl.38–41 The blue fluorescence turned weaker and the red fluorescence became stronger, compared to the control group without LPS. Moreover, the fluorescence intensity ratio (blue to red) declined obviously in the presence of LPS (Fig. 5b). The results suggested that probe IRP could sense endogenous −OCl in living cells.
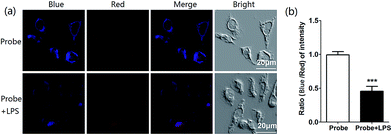 |
| Fig. 5 (a) Fluorescence images of RAW264.7 cells. First line: the cells incubated with IRP (1 μM) for 30 min, second line: the cells treated with LPS (1 μg mL−1) for 6 h, then with PMA (1 μg mL−1) for 30 min and with IRP (1 μM) for 30 min. First column: imidazo[1,5-a]pyridine fluorescence (410–520 nm), second column: rhodamine fluorescence (560–700 nm), third column: overlap images of the first and second columns, fourth column: bright field images. (b) Fluorescence intensity quantitation was analyzed using the Image J. The results were presented as mean ± SE with replicates n = 3. | |
To further determine the subcellular localization of IRP, the commercially available mitochondrial dye, Mito Tracker Deep Red, was utilized for the co-localization study (Fig. 6). RAW264.7 cells were incubated with the probe (1 μM) for 30 min and then with Mito Tracker Deep Red (0.2 μM) for 30 min. The imidazo[1,5-a]pyridine signals in IRP-loaded cells overlapped significantly with the signals of Mito Tracker Deep Red (Fig. 6c) and the co-localization coefficient was calculated to be 0.95. The results indicated that IRP could selectively stain mitochondria. In addition, we also conducted the co-localization assays of IRP with Lyso Tracker Deep Red. After RAW264.7 cells were incubated with IRP (1 μM) for 30 min and then with Lyso Tracker Deep Red (0.2 μM) for 30 min, the cells were imaged. As depicted in Fig. S6,† the probe was not site-specifically internalized in lysosomes (co-localization coefficient: 0.20). Therefore, the co-localization data confirmed that probe IRP could target mitochondria.
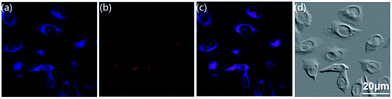 |
| Fig. 6 Fluorescence images of RAW264.7 cells co-stained with IRP (1 μM) and Mito Tracker Deep Red (0.2 μM). (a) Imidazo[1,5-a]pyridine fluorescence of IRP. (b) Fluorescence of Mito Tracker Deep Red. (c) Overlay of (a) and (b). (d) Bright field images. | |
Considering that a probe's cytotoxicity and photostability were vital parameters for the cellular bio-imaging, so we next evaluated the cytotoxicity of IRP by standard SRB assays. Fig. S7† revealed that the cell viability was affected little after incubation with IRP (1, 2, 4, 6 μM) for 12 h. Furthermore, the photostablity property of the probe was inspected and the results implied that probe IRP exhibited satisfactory photostablity within 120 s under the experimental conditions (Fig. S8†).
Experimental
Apparatus and chemicals
1H NMR (400 MHz) and 13C NMR (100 MHz) spectra were acquired on a Bruker Avance spectrometer, with d6-DMSO used as a solvent and tetramethylsilane (TMS) as an internal standard. High-resolution mass spectroscopy (HRMS) was collected on a Q-TOF6510 spectrograph (Agilent). UV-vis spectra were measured by a Hitachi U-4100 spectrophotometer. Fluorescent measurements were performed on a Perkin-Elmer LS-55 luminescence spectrophotometer. The pH was determined with a model PHS-3C pH meter (YouKe, Shanghai, China).
−OCl solution was prepared by diluting the commercially available NaOCl solution. ROS and reactive nitrogen species (RNS) solutions were prepared by the literature.42–44 Unless otherwise stated, all reagents were used without further purification from merchants. Twice-distilled water was used throughout all experiments.
Preparation of test solutions
Probe IRP was dissolved in ethanol to afford the stock solution (10−4 M). All analysis samples were prepared by displacing the stock solution into a 10 mL volumetric flask. The solution was diluted to 10 mL in a mixture solution of EtOH-PBS (pH 7.4) = 3
:
7, v/v. Appropriate aliquots of each testing species solution were then added. The resulting solutions were shaken well and incubated for 30 min before measurements.
Synthesis of probe IRP
The synthetic route of probe IRP was displayed in Scheme 1, in which compound 1 was prepared according to our previous work.30 Compound 1 (1.0 mmol) was dissolved in dry dichloromethane (20 mL) and triethylamine (3.3 mmol). The solution was cooled to 0 °C with stirring under nitrogen atmosphere. Then, chloroacetyl chloride (3.0 mmol) was added dropwise to the solution. After addition, the reaction was stirred at 0 °C for 4 h. Subsequently, the solvent was removed under reduced pressure to give compound 2. The crude product was used for next step without purification.
Compound 2 (1.0 mmol) and pyridine (1 mL) were dissolved in anhydrous acetonitrile (30 mL). The solution was refluxed overnight. Then the solvent was removed under reduced pressure and the crude product was purified by column chromatography on silica gel using dichloromethane/methanol (10/1, v/v) as the eluent to obtain IRP as a faint yellow solid (yield: 49.6%). 1H NMR (400 MHz, d6-DMSO), δ (ppm): 0.92 (t, 3H, J = 7.6 Hz, CH3), 1.09 (t, 6H, J = 6.8 Hz, CH3), 1.32 (q, 2H, J = 7.6 Hz, CH2), 1.68–1.73 (m, 2H, CH2), 2.92 (t, 2H, J = 7.4 Hz, CH2), 3.26 (br, 4H, CH2), 3.32 (br, 4H, CH2), 3.68 (br, 4H, CH2), 5.48 (s, 2H, CH2), 6.34 (d, 2H, J = 9.2 Hz, ArH), 6.49 (d, 1H, J = 8.8 Hz, ArH), 6.56–6.61 (m, 2H, ArH), 6.68 (d, 1H, J = 2.0 Hz, ArH), 6.75 (d, 1H, J = 7.2 Hz, ArH), 7.05 (d, 1H, J = 6.8 Hz, ArH), 7.52 (s, 1H, ArH), 7.54–7.62 (m, 2H, ArH), 7.85 (d, 1H, J = 6.8 Hz, ArH), 8.14–8.17 (m, 2H, ArH), 8.29 (d, 1H, J = 7.2 Hz, ArH), 8.70 (t, 1H, J = 8.0 Hz, ArH), 8.74 (d, 2H, J = 5.6 Hz, ArH), 10.60 (s, 1H, NH). 13C NMR (100 MHz, d6-DMSO), δ (ppm): 167.6, 164.2, 163.8, 153.4, 153.1, 151.8, 151.7, 149.0, 146.9, 146.1, 138.9, 134.1, 129.5, 129.4, 129.2, 128.5, 128.2, 126.4, 124.3, 123.7, 123.3, 122.8, 118.7, 115.9, 112.4, 111.7, 108.5, 108.4, 104.1, 101.9, 97.5, 65.5, 60.6, 47.9, 44.1, 29.4, 29.1, 25.6, 22.1, 14.1, 12.9. HRMS m/z: calcd for C47H48ClN8O4S [M]+: 823.3487; found: 823.3552. m/z: calcd for C47H49ClN8O4S [M + H]2+/2: 412.1782; found: 412.1790.
Cell culture and imaging
RAW264.7 cells (ATCC® TIB-71™) were cultured in Dulbecco's modified Eagle's medium (DMEM) supplemented with 10% (v/v) fetal bovine serum in an atmosphere containing 5% CO2 and 95% air at 37 °C. The cells were imaged using confocal fluorescence microscopy (Leica SP8).
Conclusion
In summary, we employed imidazo[1,5-a]pyridine-rhodamine FRET platform to develop a ratiometric fluorescent probe for −OCl. The probe was able to detect −OCl selectively and function well in mitochondrial physiological pH conditions. I575/I467 ratios of the probe responded linearly to minor fluctuations of −OCl concentration (0.5–3.5 μM). Moreover, the probe was applied to sense endogenous −OCl in RAW264.7 cells with negligible toxicity. In particular, the probe could target mitochondria due to the introduction of the quaternized pyridine moiety. Hence, we expected that the probe would be a promising candidate for highly selective and sensitive sensing of −OCl in mitochondria.
Conflicts of interest
There are no conflicts to declare.
Acknowledgements
This study was supported by the Natural Science Foundation of Shandong Province (ZR2016BB35), Foundation of Taishan Medical University (2016GCC08), National Natural Science Foundation of China (21602153 and 11574009).
Notes and references
- J. E. Harrison and J. Schultz, J. Biol. Chem., 1976, 251, 1371–1374 CAS.
- Z. M. Prokopowicz, F. Arce, R. Biedron, C. L. Chiang, M. Ciszek, D. R. Katz, M. Nowakowska, S. Zapotoczny, J. Marcinkiewicz and B. M. Chain, J. Immunol., 2010, 184, 824–835 CrossRef CAS PubMed.
- E. Hidalgo, R. Bartolome and C. Dominguez, Chem.-Biol. Interact., 2002, 139, 265–282 CrossRef CAS PubMed.
- A. Daugherty, J. L. Dunn, D. L. Rateri and J. W. Heinecke, J. Clin. Invest., 1994, 94, 437–444 CrossRef CAS PubMed.
- S. M. Wu and S. V. Pizzo, Arch. Biochem. Biophys., 2001, 391, 119–126 CrossRef CAS PubMed.
- J. T. Hou, M. Y. Wu, K. Li, J. Yang, K. K. Yu, Y. M. Xie and X. Q. Yu, Chem. Commun., 2014, 50, 8640–8643 RSC.
- K. M. Xiong, F. J. Huo, C. X. Yin, Y. Y. Chu, Y. T. Yang, J. B. Chao and A. M. Zheng, Sens. Actuators, B, 2016, 224, 307–314 CrossRef CAS.
- S. M. Chen, J. X. Lu, C. D. Sun and H. M. Ma, Analyst, 2010, 135, 577–582 RSC.
- Z. Yang, M. J. Wang, M. Y. She, B. Yin, Y. Y. Huang, P. Liu, J. L. Li and S. Y. Zhang, Sens. Actuators, B, 2014, 202, 656–662 CrossRef CAS.
- L. Yuan, W. Y. Lin and H. Chen, Biomaterials, 2013, 34, 9566–9571 CrossRef CAS PubMed.
- H. Zhu, J. L. Fan, J. Y. Wang, H. Y. Mu and X. J. Peng, J. Am. Chem. Soc., 2014, 136, 12820–12823 CrossRef CAS PubMed.
- J. L. Fan, H. Y. Mu, H. Zhu, J. Y. Wang and X. J. Peng, Analyst, 2015, 140, 4594–4598 RSC.
- Q. A. Best, N. Sattenapally, D. J. Dyer, C. N. Scott and M. E. McCarroll, J. Am. Chem. Soc., 2013, 135, 13365–13370 CrossRef CAS PubMed.
- S. Goswami, S. Paul and A. Manna, Dalton Trans., 2013, 42, 10097–10101 RSC.
- S. Goswami, A. K. Das, A. Manna, A. K. Maity, P. Saha, C. K. Quah, H. K. Fun and H. A. Abdel-Aziz, Anal. Chem., 2014, 86, 6315–6322 CrossRef CAS PubMed.
- S. Goswami, A. Manna, S. Paul, C. K. Quah and H. K. Fun, Chem. Commun., 2013, 49, 11656–11658 RSC.
- A. Manna and S. Goswami, New J. Chem., 2015, 39, 4424–4429 RSC.
- A. Manna, D. Sarkar, S. Goswami, C. K. Quah and H. K. Fun, RSC Adv., 2016, 6, 57417–57423 RSC.
- S. L. Shen, J. Y. Ning, X. F. Zhang, J. Y. Miao and B. X. Zhao, Sens. Actuators, B, 2017, 244, 907–913 CrossRef CAS.
- M. G. Ren, B. B. Deng, K. Zhou, X. Q. Kong, J. Y. Wang, G. P. Xu and W. Y. Lin, J. Mater. Chem. B, 2016, 4, 4739–4745 RSC.
- Y. R. Zhang, X. P. Chen, J. Shao, J. Y. Zhang, Q. Yuan, J. Y. Miao and B. X. Zhao, Chem. Commun., 2014, 50, 14241–14244 CAS.
- G. W. Chen, F. L. Song, J. Y. Wang, Z. G. Yang, S. G. Sun, J. L. Fan, X. X. Qiang, X. Wang, B. R. Dou and X. J. Peng, Chem. Commun., 2012, 48, 2949–2951 RSC.
- S. L. Shen, X. Zhao, X. F. Zhang, X. L. Liu, H. Wang, Y. Y. Dai, J. Y. Miao and B. X. Zhao, J. Mater. Chem. B, 2017, 5, 289–295 RSC.
- D. X. Li, Y. Feng, J. Z. Lin, M. Chen, S. X. Wang, X. Wang, H. T. Sheng, Z. L. Shao, M. Z. Zhu and X. M. Meng, Sens. Actuators, B, 2016, 222, 483–491 CrossRef CAS.
- Z. Xu and L. Xu, Chem. Commun., 2016, 52, 1094–1119 RSC.
- L. Yuan, L. Wang, B. K. Agrawalla, S. J. Park, H. Zhu, B. Sivaraman, J. J. Peng, Q. H. Xu and Y. T. Chang, J. Am. Chem. Soc., 2015, 137, 5930–5938 CrossRef CAS PubMed.
- W. Xu, Z. B. Zeng, J. H. Jiang, Y. T. Chang and L. Yuan, Angew. Chem., Int. Ed., 2016, 55, 13658–13699 CrossRef CAS PubMed.
- H. D. Xiao, K. Xin, H. F. Dou, G. Yin, Y. W. Quan and R. R. Wang, Chem. Commun., 2015, 51, 1442–1445 RSC.
- J. T. Hou, K. Li, J. Yang, K. K. Yu, Y. X. Liao, Y. Z. Ran, Y. H. Liu, X. D. Zhou and X. Q. Yu, Chem. Commun., 2015, 51, 6781–6784 RSC.
- Y. Q. Ge, R. X. Ji, S. L. Shen, X. Q. Cao and F. Y. Li, Sens. Actuators, B, 2017, 245, 875–881 CrossRef CAS.
- S. L. Shen, X. F. Zhang, Y. Q. Ge, Y. Zhu and X. Q. Cao, Sens. Actuators, B, 2018, 254, 736–741 CrossRef CAS.
- H. Ma, B. Song, Y. X. Wang, C. L. Liu, X. Wang and J. L. Yuan, Dyes Pigm., 2017, 140, 407–416 CrossRef CAS.
- Z. Zhang, J. L. Fan, G. H. Cheng, S. Ghazali, J. J. Du and X. J. Peng, Sens. Actuators, B, 2017, 246, 293–299 CrossRef CAS.
- J. Kang, F. J. Huo, Y. K. Yue, Y. Wen, J. B. Chao, Y. B. Zhang and C. X. Yin, Dyes Pigm., 2017, 136, 852–858 CrossRef CAS.
- G. J. Song, S. Y. Bai, X. Dai, X. Q. Cao and B. X. Zhao, RSC Adv., 2016, 6, 41317–41322 RSC.
- X. Zhang, G. J. Song, X. J. Cao, J. T. Liu, M. Y. Chen, X. Q. Cao and B. X. Zhao, RSC Adv., 2015, 5, 89827–89832 RSC.
- Y. Q. Ge, X. J. Xing, A. K. Liu, R. X. Ji, S. L. Shen and X. Q. Cao, Dyes Pigm., 2017, 146, 136–142 CrossRef CAS.
- Q. L. Xu, C. H. Heo, J. A. Kim, H. S. Lee, Y. Hu, D. Y. Kim, K. M. K. Swamy, G. Kim, S. J. Nam, H. M. Kim and J. Y. Yoon, Anal. Chem., 2016, 88, 6615–6620 CrossRef CAS PubMed.
- X. Z. Wang, L. Zhou, F. Qiang, F. Y. Wang, R. Wang and C. C. Zhao, Anal. Chim. Acta, 2016, 911, 114–120 CrossRef CAS PubMed.
- S. V. Mulay, M. Choi, Y. J. Jang, Y. Kim, S. Jon and D. G. Churchill, Chem.–Eur. J., 2016, 22, 9642–9648 CrossRef CAS PubMed.
- G. H. Cheng, J. L. Fan, W. Sun, J. F. Cao, C. Hu and X. J. Peng, Chem. Commun., 2014, 50, 1018–1020 RSC.
- W. L. Wu, Z. M. Zhao, X. Dai, L. Su and B. X. Zhao, Sens. Actuators, B, 2016, 232, 390–395 CrossRef CAS.
- X. H. Li, G. X. Zhang, H. M. Ma, D. Q. Zhang, J. Li and D. B. Zhu, J. Am. Chem. Soc., 2004, 126, 11543–11548 CrossRef CAS PubMed.
- Z. Ma, W. Sun, L. Z. Chen, J. Li, Z. Z. Liu, H. X. Bai, M. Y. Zhu, L. P. Du, X. D. Shi and M. Y. Li, Chem. Commun., 2013, 49, 6295–6297 RSC.
Footnote |
† Electronic supplementary information (ESI) available. See DOI: 10.1039/c7ra11086c |
|
This journal is © The Royal Society of Chemistry 2017 |
Click here to see how this site uses Cookies. View our privacy policy here.