DOI:
10.1039/C7RA10538J
(Paper)
RSC Adv., 2017,
7, 55012-55019
Enhanced xenon adsorption and separation with an anionic indium–organic framework by ion exchange with Co2+†
Received
23rd September 2017
, Accepted 27th November 2017
First published on 4th December 2017
Abstract
The separation of xenon/krypton is industrially significant and an environmental concern. Adsorptive capture and separation xenon from krypton using porous MOFs provides an energy and capital efficient approach compared with the current cryogenic distillation process. Herein, we investigated the adsorptive Xe/Kr separation potential of three anion In-MOFs (CPM-5, CPM-6 and the Co2+-exchanged framework analogue Co2+-CPM-6). Anionic In-MOF Co2+-CPM-6 with Co2+ ions in pore spaces has been obtained using a simple cation-exchange process and exhibits much higher Xe adsorption capacity and Xe/Kr selectivity than organic cation-analogues CPM-5 ([(CH3)2NH2]+) and CPM-6 ([CH3NH3]+), verified by single-component gas isotherms, IAST calculations and breakthrough experiments. The enhanced adsorptive Xe/Kr separation performance for Co2+-CPM-6 could be due to the increased pore size or accessible micropore volume and enhanced electric field within the pore spaces, which could induce strong interaction with Xe and simultaneously reduce the affinity with Kr.
Introduction
Rare or noble gases xenon (Xe) and krypton (Kr) are commonly encountered in low concentrations (Xe exists in the atmosphere at 0.087 ppmv, and krypton (Kr) at 1.14 ppmv) and their extraction is consequently difficult.1 However, these noble gases are industrially valuable and have many significant uses including commercial lighting, medical imaging, insulation and lasers.1,2 On the other hand, radioisotopes of xenon and krypton (85Kr and 133Xe) are released as by-products from nuclear reprocessing plants. For environmental concerns and recycling of noble gases, the long-lived and hazardous 85Kr (half life of 10.8 years) should be separated and captured from effluent gas mixtures of nuclear fuel reprocessing plants.3,4 Meanwhile, separation and capture of xenon from radioactive krypton in the off-gas stream after a short-time decay of the short half-life xenon isotope would provide a new resource of xenon for industrial use. Currently, a 20/80 molar mixture of Xe–Kr obtained as a by-product in cryogenic air separation must undergo further cryogenic distillation to produce pure xenon and krypton. And cryogenic process is also suggested for the removal of radioactive noble gases from off-gas streams in future nuclear reprocessing plants. However, this process is highly energy and capital intensive.5 Therefore, effective capture and separation of Xe–Kr at room temperature becomes an urgent and significant issue to be resolved.
Adsorptive separation of Xe–Kr using porous materials at room temperature could be a suitable alternative to cryogenic process for its easy operation and low costs. Thus, adsorbents with high Xe/Kr selectivity and capacity are essential for the design of adsorptive separation process such as pressure swing adsorption (PSA) and vacuum swing adsorption (VSA).6 In this regard, zeolites and active carbon have been used and evaluated for Xe/Kr separation at ambient conditions.7–9 However, their low Xe capacity and selectivity limits their practical use. Metal–organic frameworks (MOFs), a new kind of hybrid crystalline porous material which are hybrid lattices of organic ligands and metal nodes (metal ions or clusters), have attracted enormous attention over the past two decades for its ultrahigh specific surface area, tunable pore structure, adjustable chemical environment and high thermal stability.10–12 Owing to this feature, MOFs appear to be promising as cost-effective and efficient adsorbents for gas storage and separation.13–21 Recently, the effective separation of noble gases Xe and Kr using MOFs has received much more attention and become a new area of focus.22–36
Up to date, studies on Xe/Kr separation using MOFs are still scarce.37 Wang et al. showed that Co3(HCOO)6 can commensurately adsorb Xe and high Xe/Kr selectivity due to the unique pore size and channel shape.38 Thallapally et al. reported that NiDOBDC adsorbs more Xe compared to activated carbon and also showed that silver loading within NiDOBDC notably enhances both Xe capacity and Xe/Kr selectivity.39,40 Cooper et al. reported that a porous organic cage CC3, though not a MOF, shows significantly high Xe capacity and Xe/Kr selectivity at low concentrations.41 Xiong et al. reported a flexible zinc tetrazolate framework [Zn(mtz)2] which exhibits breathing behaviour on Xe adsorption and has a high Xe/Kr selectivity measured by breakthrough experiment using binary Xe–Kr gas mixture.42 Recently, Thallapally et al. reported that SBMOF-1 exhibits by far the highest reported xenon adsorption capacity and a remarkable Xe/Kr selectivity under conditions pertinent to nuclear fuel reprocessing.43 Computational screening studies carried by Snurr et al. suggest that MOFs with cylindrical pores just large enough to fit a single xenon atom would have the highest Xe/Kr selectivity but better materials remains to be discovered.44
Recent studies have indicated several features that are desirable for enhancing adsorption capacity and selectivity of xenon at room temperature. One feature is the suitable pore size commensurate with the size of Xe molecule (4.1 Å). Another factor currently receiving considerable attention is introducing individual localized binding sites using methods such as (1) deposition of Ag clusters in the porous MOFs and (2) creation of unsaturated metal sites (also called open-metal sites) and more polarizing organic groups (–I, –OH).40,45–47 Furthermore, the porous solid-Xe molecule interactions can also be enhanced by employing the electric field across the pore space that is capable of polarizing Xe molecules and increasing the interaction with charged internal pore surface or charged extraframework species.40 However, this approach is rarely studied because most of MOFs exhibit an electrically neutral framework. Thus, it is essential and meaningful to investigate the Xe adsorption and separation properties of anionic MOFs and the influence of cations on their separation performance. Indium(III) often exhibits an eight-coordinated central building block with four equivalent carboxylate ligands and has been frequently used to design and synthesis of anionic MOFs with low connectivity and high surface areas. Two anionic In-MOF: [(CH3)2NH2][In3O(BTC)2(H2O)3]2[In3(BTC)4]·solvent (denoted as CPM-5, CPM = crystalline porous materials) and [CH3NH3][In3O(BTC)2(H2O)3]2[In3(BTC)4]·solvent (CPM-6, BTC = 1,3,5-benzenetricarboxylate) firstly reported by Zheng et al. exhibit high surface areas, good hydrothermal stability, coupled with suitable pore size (4–6 Å) and strong charge-induced forces within the internal pore space which render them good candidates for the study of Xe selective sorption properties.
In this work, we firstly describe facile separation of Xe from Kr at room temperature using three anionic microporous MOFs based upon In(III) ions, CPM-5, CPM-6 and the Co2+-exchanged framework analogue Co2+-CPM-6. More smaller and higher polar Co2+ ions could be easily introduced into the pores of the anionic microporous framework using a simple ion-exchange with organic cations ([CH3NH3]+), which provides an approach to tune the pore volume and electric field across the pore space. Experimental adsorption isotherms and breakthrough curves under packed-bed mixture flow conditions indicates that Co2+-CPM-6 exhibits much higher Xe adsorption capacity and Xe/Kr selectivity compared with its organic cation-analogues CPM-5 ([(CH3)2NH2]+) and CPM-6 ([CH3NH3]+).
Experimental
Materials and measurements
All reagents and solvents were used as received from commercial suppliers without further purification. Thermogravimetric analyses (TGA) were performed on a TA TGA/Q500 analyzer with heating rate of 5 °C min−1 from 27 to 800 °C. Powder X-ray diffraction (PXRD) patterns were recorded by a Bruker D8-advance Powder X-ray Diffractometer Instrument operated at 40 kV and 44 mA with a scan of 1.0 deg min−1. Analyses for the concentrations of Co2+, In3+ were carried out on an Inductively Coupled Plasma-Optical Emission Spectroscopy (ICP-OES) analyzer. Calibration curves for ICP-OES were prepared by dilution of commercially available standards with the sample dissolves in concentrated HCl, and diluted to proper concentration for measurement. The verification of Co2+ in CPM-6 was also carried out on a Perkin-Elmer PHI-5702 multi-functional X-ray photoelectron spectroscopy (XPS) with Al Kα radiation.
Preparation of CPM-5 and CPM-6
CPM-5 was synthesized as a slight modification of the procedure of ref. 49, in which a mixture of 0.2 g In(NO3)3·5H2O and 0.17 g H3BTC was stirred in a mixed solution of H2O/DMF (1.0 g/4.0 g) for 1 hour, and then transferred to the Teflon-lined stainless steel autoclave (20 ml), and kept at 120 °C for 5 days. CPM-6 was synthesized using the same procedure in which the DMF was replaced by NMF. After washed by methanol several times, the colorless crystals were obtained.
Preparation of Co2+-CPM-6
The as-prepared CPM-6 sample was ion-exchanged with Co2+, immersed in methanol containing 0.1 M Co(NO3)2 and changed with fresh new Co(NO3)2 methanol solution every day for four days. And then the samples were filtered and washed with methanol several times to obtain Co2+ ions exchanged CPM-6 designated as Co2+-CPM-6.
Gas adsorption measurements
Xe, Kr, Ar, N2 adsorption and desorption isotherms were measured on the Micromeritics 3Flex Surface Characterization Analyzer. To get the solvent free framework, the fresh samples of CPM-5 and CPM-6 were guest-exchanged with dry methanol 4 times per day for 2 days, filtered and degassed at ambient temperature for 6 h, and then vacuumed at 180 °C overnight to measurements. The fresh samples of Co2+-CPM-6 were filtered and washed by methanol several times and degassed at ambient temperature for 6 h, and then vacuumed at 180 °C overnight to measurements. The adsorption isotherms at 77 K were measured upon the liquid nitrogen bath. The temperatures of adsorption isotherms at 273 K and 298 K were maintained using a Micromeritics's ISO Controller (Sub-Ambient, Thermoelectric Cooled Dewar). Ultrahigh-purity-grade (>99.999%) Xe, Kr, Ar, N2 and He gases were applied in the adsorption measurements.
Results and discussion
Characterization of CPM-5, CPM-6 and Co2+-CPM-6
CPM-5 and CPM-6 were synthesized using a slight modification of a published procedure.49 The phase purity of the sample was confirmed by PXRD. CPM-5 and CPM-6 firstly reported by Zheng et al. have the same framework structure just with different organic cations ((CH3)2NH2+ for CPM-5, CH3NH3+ for CPM-6). As shown in Fig. 1, CPM-5 and CPM-6 have a fascinating cage-within-cage architecture structure. The bigger In24 sodalite cage built with negatively charged single-metal building blocks {In(CO2)4} and BTC3− ligands with 25.7 × 25.7 × 25.7 Å3 in dimension is far too large for capture of Xe atoms. The small inner In12 cage comprised of four positively charged trimeric clusters {In3O} and three BTC3− ligands with a dimension of 8.7 Å. The In12 cage was interconnected with the In24 cage by 12 BTCs ligands which segment the pore space of the large sodalite cage into multiple domains. Drifting cations – (CH3)2NH2+ (CPM-5) and CH3NH3+ (CPM-6) are amid these networks. The whole pore space partitioned by BTC linkers and occupied by cations exhibits microporous pore network structure. The micropore size of 4–6 Å was calculated using Horvath–Kawazoe method. Additionally, removal of the three dangling water on {In3(O)(O2CR)6(H2O)3} of the inner In12 cage during the activation procedure generates In3+ sites being exposed to pore surface for Xe adsorption.48 Interestingly, the outer In24 cage composed of {In(CO2)4} units is negatively charged while the inner In12 cage made from {In3(O)(O2CR)6(H2O)3} units is positively charged, resulting in an electric field within the pore space.49 What's more, the organic cations within the pores could be easily exchanged by metal ions to tune the pore size and electric field for Xe adsorption.50 In this work, we choose Co2+ ions to exchange with [CH3NH3]+ using the as-synthesized CPM-6 samples based on several reasons: (1) bivalent Co2+ ions having higher polarizing ability compared to [CH3NH3]+ and bivalent metal ion would enhance electric field within the pore space, (2) for M-MOF-74 (M = Co2+, Mg2+, Zn2+ and Ni2+), Co2+ shows significantly stronger binding to Xe molecules than Mg2+, Zn2+ and Ni2+, which has been proved by experimental and simulated results,45,51,52 (3) Co2+ is smaller than [CH3NH3]+, consequently releasing more space volume for Xe adsorption. Co2+-exchange sample, Co2+-CPM-6 was obtained by soaking as-synthesized CPM-6 samples into a methanol solution of Co(NO3)2. In3+/Co2+ molar ratio in Co2+-CPM-6 measured by ICP-OES is 29, which means 62% [CH3NH3]+ cations has been replaced by Co2+ ions. And XPS result of Co2+-CPM-6 (see Fig. S13†) also showed that Co2+ ions were successfully introduced into the framework.
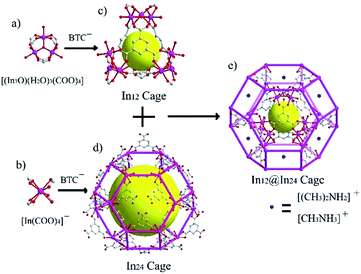 |
| Fig. 1 (a–e) Structures of trimeric {(In3O)(H2O)3} unit, monomeric In3+ ion, In12 cage, In24 cage and In12@In24 cage, respectively. The purple solid lines represent BTCs. The blue solid balls represent the cations ([(CH3)2NH2]+, [CH3NH3]+). The small yellow ball represents the In12 cage cavity. The big yellow ball represents In24 cage cavity. | |
Powder X-ray diffraction patterns indicate that pure phase samples of CPM-5 and CPM-6 have been successfully synthesized (shown in Fig. 2). Co2+-CPM-6 nearly has the same PXRD patterns with CPM-6, which shows that Co2+-CPM-6 maintains its framework structure and crystalline state well during the Co2+-exchange process. And the PXRD patterns for the activated samples illustrate that CPM-5, CPM-6 and Co2+-CPM-6 are stable enough for gas sorption measurements after their activation procedure.
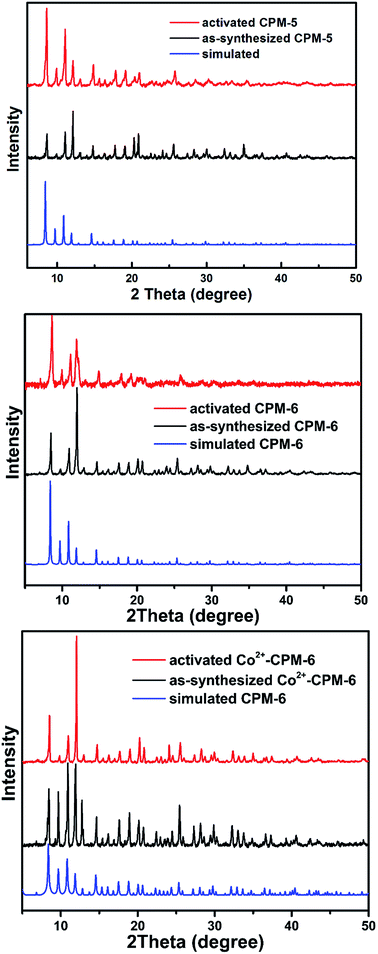 |
| Fig. 2 The PXRD patterns of CPM-5, CPM-6, and Co2+-CPM-6. | |
As shown in Fig. 3, N2 sorption isotherms of CPM-5, CPM-6 and Co2+-CPM-6 at 77 K indicate that all three samples exhibit a typical reversible type-I behavior. We adopted two models: Langmuir and BET surface area to precisely evaluate the specific surface areas of these three materials. The Langmuir and BET surface areas of CPM-5 are 768 m2 g−1 and 521 m2 g−1, respectively. And the micropore volume of CPM-5 (using t-plot method) was also calculated to be 0.242 cm3 g−1. The (Langmuir) BET surface areas and micropore volume of CPM-6 were calculated to be (871 m2 g−1) 583 m2 g−1 and 0.282 cm3 g−1, which are higher than that of CPM-5. This could be due to small cation size of [CH3NH3]+ in CPM-6 taking up less pore space compared with [(CH3)2NH2]+ in CPM-5. The surface area and micropore volume of CPM-5 and CPM-6 in this work are consistent with the reported literature.49 Co2+-CPM-6 with the small Co2+ ions for charge-compensating exhibits the highest (Langmuir) BET surface areas and micropore volume compared with CPM-5 and CPM-6 and were calculated to be (1055) 671 m2 g−1 and 0.305 cm3 g−1, respectively. Langmuir model has a relative higher value than BET model. The differences between Langmuir and BET surface areas could be attributed to the different hypothesis that Langmuir model assumes monolayer adsorption while BET model assumes multilayer adsorption. Thus it is a reasonable result that Langmuir model has a higher value than BET model. However, the specific surface areas of these two models don't differ too much, which manifests that CPM-5, CPM-6 and Co2+-CPM-6 belong to the micropore materials and the pore sizes won't exceed 7 Å (see Fig. S3†). The DFT pore sizes distribution calculated by DFT model shows that Co2+-CPM-6 exhibits a little decrease of pore space in the range of 5.4–6.4 Å and a distinct increase of pore space in the range of 6.4–8.6 Å compared with CPM-6. These results show that ion-exchange with small metal ions in anionic framework could provide an effective way to enhance the specific surface area and adjust the micropore volume or pore size (see Fig. S3†).
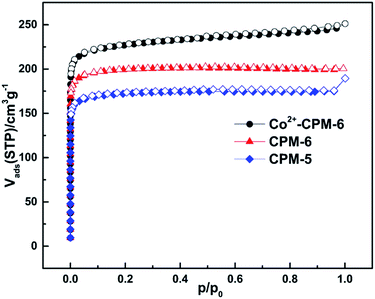 |
| Fig. 3 N2 sorption isotherms of CPM-5, CPM-6 and Co2+-CPM-6 at 77 K. | |
Single component sorption isotherms, Henry's constants and isosteric heats of adsorption
The establishment of the permanent porosity enabled us to examine their gas sorptions of Xe and Kr. As shown in Fig. 4. Pure adsorption and desorption isotherms for Xe and Kr in CPM-5, CPM-6 and Co2+-CPM-6 were measured at 298 K and 273 K up to 1 bar. Adsorption and desorption isotherms for Xe and Kr are fully reversible and the sorption of Xe exhibits type-I behavior, which was typical for strong adsorbates sorption in microporous materials. The uptake of Xe at 298 K and 1 bar for CPM-5 and CPM-6 are 2.44 mmol g−1 and 2.89 mmol g−1, 3.4 times and 3.7 times higher than their Kr uptakes (0.71 mmol g−1 for CPM-5 and 0.78 mmol g−1 for CPM-6). The Xe uptake of Co2+-CPM-6 at 298 K and 1 bar is 3.20 mmol g−1, which is 4.3 times higher than its Kr uptake (0.75 mmol g−1). It should be noted that Co2+-CPM-6 has an obvious higher Xe uptake than CPM-6 but a slightly low Kr uptake compared with CPM-6. The enhancement of Xe adsorption capacity for Co2+-CPM-6 could be due to an increase in the accessible micropore volume. The slightly lower Kr uptake of Co2+-CPM-6 may be due to the decrease of micropore volumes in the range of 5.4–6.4 Å, that are suitable for Kr adsorption.
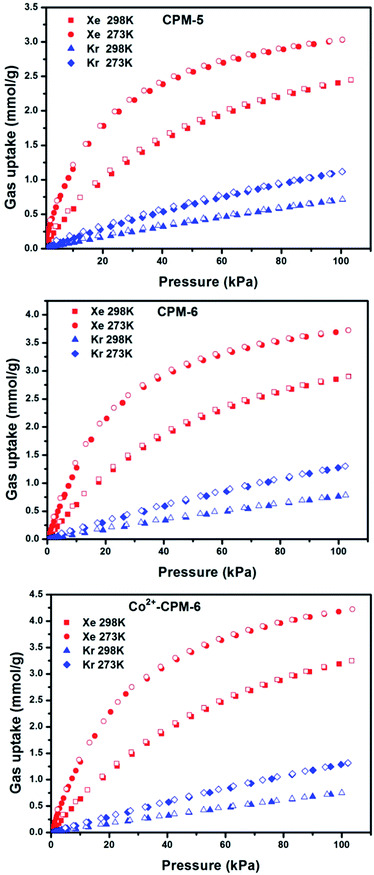 |
| Fig. 4 Xe and Kr adsorption and desorption isotherms of CPM-5, CPM-6 and Co2+-CPM-6 at 273 K and 298 K. | |
In order to understand and explain the different performances for these three MOFs on uptake of Xe over Kr, we calculated the Henry's constants based on single component sorption isotherms to evaluate the gas–adsorbent interactions. Henry's constant is a useful measure of adsorbents' affinity for adsorbates, since it represents the partition of the adsorbate between its bulk phase and adsorbed phase at very low pressures.38 The Henry's constants for Xe and Kr in CPM-5, CPM-6 and Co2+-CPM-6 were calculated using low-pressure isotherms at 298 K. We fitted the low-pressure isotherms which passed through the original point and appeared linear fit, and obtained slopes of these fitting lines which represent the Henry's constants (see Fig. S6–S11†). As shown in Table 1, the order of the calculated Henry's constants for Xe is Co2+-CPM-6 > CPM-6 > CPM-5. Interestingly, the order of the calculated Henry's constant for Kr is CPM-5 > CPM-6 > Co2+-CPM-6, which is opposite to the order of the Henry's constants for Xe. The enhancement of Henry's constant for Xe in Co2+-CPM-6 could be due to the more polarized electric field across the pore space or strong interaction between Co2+ ions and Xe molecules. The lower Henry's constant for Kr in Co2+-CPM-6 is main due to the decreased pore volumes in the range of 5.4–6.4 Å that are just fit for adsorption of Kr atoms. To verify adsorption strength of Xe and Kr in CPM-5, CPM-6 and Co2+-CPM-6, we calculated the isosteric heats of adsorption (Qst) from single component adsorption isotherms as a function of quantity adsorbed at different temperatures (273 K and 298 K) using the Clausius–Clapeyron equation. As shown in Fig. 5, Xe Qst values of CPM-5 and CPM-6 at low loadings are 25.0 kJ mol−1 and 25.1 kJ mol−1, which are nearly the same. Co2+-CPM-6 has a higher Xe Qst value (25.9 kJ mol−1) than CPM-5 and CPM-6. The Kr Qst values of these three MOFs at low loading are as follows: CPM-5 (17.3 kJ mol−1) > CPM-6 (17.1 kJ mol−1) > Co2+-CPM-6 (16.6 kJ mol−1), which matches well with the order of the Henry's constants for Kr. All these results indicates that introduce of small and polar Co2+ ions could enhance the Xe interaction with pore surface and reduce affinity of Kr. The Xe/Kr selectivity calculated by the radio of Henry's constants follows the order: Co2+-CPM-6 (7.8) > CPM-6 (7.2) > CPM-5 (6.8), which indicates that Co2+-CPM-6 has the strongest interaction with Xe molecules of these three MOFs.
Table 1 Gas uptake (mmol g−1) at 298 K and 1 bar, Henry constants (mmol g−1 bar−1) and Qst (kJ mol−1) on CPM-5, CPM-6 and Co2+-CPM-6a
|
NXe (mmol g−1) |
NKr (mmol g−1) |
NXe/NKr |
HXe (mmol g−1 bar−1) |
HKr (mmol g−1 bar−1) |
HXe/HKr |
Qst(Xe) |
Qst(Kr) |
NXe and NKr represent Xe and Kr uptake, respectively; HXe and HKr represent Henry's constant of Xe and Kr. |
CPM-5 |
2.44 |
0.71 |
3.1 |
6.06 |
0.89 |
6.8 |
25.0 |
17.3 |
CPM-6 |
2.89 |
0.78 |
3.7 |
6.15 |
0.85 |
7.2 |
25.1 |
17.1 |
Co2+-CPM-6 |
3.20 |
0.75 |
4.3 |
6.28 |
0.81 |
7.8 |
25.9 |
16.6 |
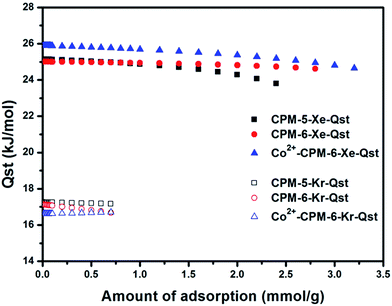 |
| Fig. 5 The isosteric heats of adsorption (Qst) for Xe and Kr in CPM-5, CPM-6 and Co2+-CPM-6. | |
IAST selectivity
Ideal adsorbed solution theory (IAST) is a thermodynamic method which can predict the selectivity of gas mixtures based on the experimental single component adsorption isotherms and has been proved to be an effective approach to evaluate the separation ability of various materials.53–55 In order to obtain good prediction by IAST, pure isotherms of Xe and Kr were fitted using the dual-site Langmuir–Freundlich (DSLF) model (see Table S1†).56 In this work, we predicted the Xe/Kr selectivity by IAST for 20/80 Xe/Kr gas mixture, which is an industrially relevant composition for Xe–Kr gas mixture to assess the potential of CPM-5, CPM-6 and Co2+-CPM-6 on Xe/Kr separation. As shown in Fig. 6, Co2+-CPM-6 exhibits a distinct enhancement on Xe/Kr selectivity compared with CPM-6 after the Co2+-exchange process. The IAST Xe/Kr selectivities of these three materials follow the order of Co2+-CPM-6 (9.3) > CPM-6 (7.3) > CPM-5 (7.1) at 100 kPa and 298 K, which agrees with the results from Henry's constants. This could be attributed to the stronger electric field across the pore space with Co2+ ions as compensating cations in Co2+-CPM-6, that is capable of polarizing Xe atoms and increasing its interaction with charged pore surface or Co2+ ions. The IAST Xe/Kr selectivity of Co2+-CPM-6 is among the reported high Xe/Kr selectivity porous materials: SBMOF-1 (16), SBMOF-2 (10), Co3(HCOO)6 (11), CC3 (12.5), Ag@MOF-74Ni (11.5), Co-MOF-74 (12).43,46
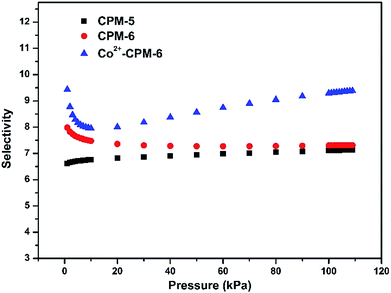 |
| Fig. 6 IAST-predicted Xe/Kr selectivities of CPM-5, CPM-6 and Co2+-CPM-6 for 20/80 Xe/Kr gas mixture at 298 K. | |
Dynamic breakthrough experiments
We also carried out the breakthrough experiments at room temperature to evaluate the potential of these three MOFs for the adsorptive separation of Xe/Kr mixtures, under the real world conditions (see Fig. S12†). Fig. 7 shows the breakthrough curves of Xe and Kr upon separation of a 20/80 Xe/Kr mixture on the column packed with CPM-5, CPM-6 and Co2+-CPM-6 at 298 K and 1 bar. For all three MOFs, Kr firstly elutes from the column, and was detected at ∼9 min g−1 after the gas mixture was introduced into the column. While Xe retains strongly in the column and was detected at a breakthrough time of ∼15 min g−1 for CPM-5, 24 min g−1 for CPM-6 and 35 min g−1 for Co2+-CPM-6, respectively. The Kr concentration at the column outlet exceeding the feed concentration indicates a significantly stronger interaction for Xe molecules than Kr molecules with the adsorbents during the breakthrough process. The Xe/Kr selectivity of CPM-5, CPM-6 and Co2+-CPM-6 was calculated to be 6.2, 6.6 and 8.9 respectively, based on the adsorption capacity of the two gases, agreeable with the IAST selectivity derived from the single gas adsorption isotherms. These results indicate that after Co2+-exchange process, Co2+-CPM-6 has a remarkable higher Xe/Kr selectivity than CPM-5 and CPM-6 not only at equilibrium, but also under kinetic flow conditions. What's more, Co2+-CPM-6 exhibits less diffusion effect for Xe than CPM-5 and CPM-6 in the breakthrough process and would provide better Xe/Kr separation performance in the practical application. This could be due to the improvement of pore size and micropore volumes in the framework during the Co2+-exchange process. The high Xe capacity and Xe/Kr selectivity suggests Co2+-CPM-6 a promising candidate for Xe/Kr adsorptive separation.
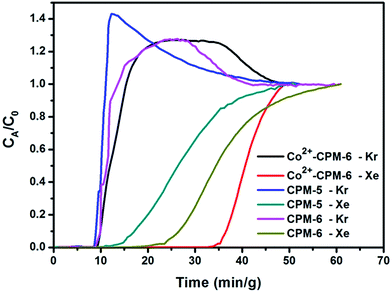 |
| Fig. 7 The breakthrough curves of CPM-5, CPM-6 and Co2+-CPM-6 for a 20 : 80 Xe/Kr gas mixture at 298 K and 1 bar. The flow rate of He and Xe/Kr gas mixture is 5 ml min−1. | |
Conclusions
In this work, we have investigated adsorptive Xe/Kr separation potential of three anionic porous indium–organic frameworks (CPM-5, CPM-6 and the Co2+-exchanged framework analogue Co2+-CPM-6). A new anionic In-MOF Co2+-CPM-6 with Co2+ ions in pore spaces has been obtained using a simple cation-exchange process and exhibits much higher Xe adsorption capacity and Xe/Kr selectivity than CPM-5 and CPM-6, verified by the single-component gas isotherms, IAST calculations and breakthrough experiments. Small and polarized Co2+ ions could increase the pore size or accessible micropore volumes and enhance the electric field within pore space, which could induce strong interaction with Xe and simultaneously reduce the affinity with Kr, suggesting the potential of Co2+-CPM-6 to be a promising candidate material for Xe/Kr adsorptive separation. The introduction of small and polarized metal ions such as Co2+ ions into an anionic MOF to tune its pore size or pore volume and electric field across pore space may be a promising strategy to enhance Xe adsorption capacity and Xe/Kr separation performance of MOFs.
Conflicts of interest
Authors declare no conflicts of interest and have reached an agreement of the final version of manuscript.
Acknowledgements
This work was supported by “Radiochemistry 909 program” in China Academy of Engineering Physics (CAEP), the National Natural Science Foundation of China (Grants 21501158).
Notes and references
- F. G. Kerry, Industrial Gas Handbook: Gas Separation and Purification, CRC Press, Boca Raton, Florida, 2007 Search PubMed.
- S. C. Cullen and E. G. Gross, Science, 1951, 113, 580–582 CAS.
- A. Turkevich, L. Winsberg, H. Flotow and R. M. Adams, Proc. Natl. Acad. Sci. U. S. A., 1997, 94, 7807–7810 CrossRef CAS.
- N. R. Soelberg, T. G. Garn, M. R. Greenhalgh, J. D. Law, R. Jubin, D. M. Strachan and P. K. Thallapally, Sci. Technol. Nucl. Install., 2013, 72496 Search PubMed.
- J. Izumi, Waste Gas Treatment using Zeolites in Nuclear-Related Industries, in Handbook of Zeolites Science and Technology, Marcel Dekker, Inc., New York, 2003 Search PubMed.
- R. T. Yang, Adsorbents: Fundamentals and Applications, John Wiley & Sons. Inc., Hoboken, 2003 Search PubMed.
- C. G. Saxton, A. Kruth, M. Castro, P. A. Wright and R. F. Howe, Microporous Mesoporous Mater., 2010, 129, 68–73 CrossRef CAS.
- R. E. Bazan, M. Bastos-Neto, A. Moeller, F. Dreisbach and R. Staudt, Adsorption, 2011, 17, 371–383 CrossRef CAS.
- X. Feng, Z. Zong, S. K. Elsaidi, J. B. Jasinski, R. Krishna, P. K. Thallapally and M. A. Carreon, J. Am. Chem. Soc., 2016, 138, 9791–9794 CrossRef CAS PubMed.
- G. Férey, Chem. Soc. Rev., 2008, 37, 191–214 RSC.
- S. Kitagawa, R. Kitaura and S. Noro, Angew. Chem., Int. Ed., 2004, 43, 2334–2375 CrossRef CAS PubMed.
- O. M. Yaghi, M. O'Keeffe, N. W. Ockwig, H. K. Chae, M. Eddaoudi and J. Kim, Nature, 2003, 423, 705–714 CrossRef CAS PubMed.
- D. M. D'Alessandro, B. Smit and J. R. Long, Angew. Chem., Int. Ed., 2010, 49, 6058–6082 CrossRef PubMed.
- R. Matsuda, R. Kitaura, S. Kitagawa, Y. Kubota, R. V. Belosludov, T. C. Kobayashi, H. Sakamoto, T. Chiba, M. Tkata, Y. Kawazoe and Y. Mita, Nature, 2005, 436, 238–241 CrossRef CAS PubMed.
- Z. Zhang, Z. Z. Yao, S. Xiang and B. Chen, Energy Environ. Sci., 2014, 7, 2868–2899 CAS.
- S. Xiang, Y. He, Z. Zhang, H. Wu, W. Zhou, R. Krishna and B. Chen, Nat. Commun., 2012, 3, 954–960 CrossRef PubMed.
- J. R. Li, R. J. Kuppler and H. C. Zhou, Chem. Soc. Rev., 2009, 38, 1477–1504 RSC.
- P. Nugent, Y. Belmabkhout, S. D. Burd, A. J. Cairns, R. Luebke, K. Forrest, T. Pham, S. Ma, B. Space, L. Wojtas, M. Eddaoudi and M. J. Zaworotko, Nature, 2013, 495, 80–84 CrossRef CAS PubMed.
- S. S. Xiong, Y. J. Gong, H. X. Wang, H. L. Wang, Q. Liu, M. Cu, X. L. Wang, B. L. Chen and Z. Y. Wang, Chem. Commun., 2014, 50, 12101–12104 RSC.
- R. E. Morris and P. S. Wheatley, Angew. Chem., Int. Ed., 2008, 47, 4966–4981 CrossRef CAS PubMed.
- J. R. Li, Y. G. Ma, M. C. McCarthy, J. Sculley, J. M. Yu, H. K. Jeong, P. B. Balbuena and H. C. Zhou, Coord. Chem. Rev., 2011, 255, 1791–1823 CrossRef CAS.
- M. V. Parkes, C. L. Staiger, J. J. Perry IV, M. D. Allendorf and J. A. Greathouse, Phys. Chem. Chem. Phys., 2013, 15, 9093–9106 RSC.
- T. Van Heest, S. L. Teich-McGoldrick, J. A. Greathouse, M. D. Allendorf and D. S. Sholl, J. Phys. Chem. C, 2012, 116, 13183–13195 CAS.
- Y. S. Bae, B. G. Hauser, Y. J. Colon, J. T. Hupp, O. K. Farha and R. Q. Snurr, Microporous Mesoporous Mater., 2013, 169, 176–179 CrossRef CAS.
- K. V. Lawler, Z. Hulvey and P. M. Forster, Chem. Commun., 2013, 49, 10959–10961 RSC.
- C. A. Fernandez, J. Liu, P. K. Thallapally and D. M. Strachan, J. Am. Chem. Soc., 2012, 134, 9046–9049 CrossRef CAS PubMed.
- C. M. Simon, R. Mercado, S. K. Schnell, B. Smit and M. Haranczyk, Chem. Mater., 2015, 27, 4459–4475 CrossRef CAS.
- S. J. Lee, T. U. Yoon, A. R. Kim, S. Y. Kim, K. H. Cho, Y. K. Hwang, J. W. Yeon and Y. S. Bae, J. Hazard. Mater., 2016, 320, 513–520 CrossRef CAS PubMed.
- J. Liu, P. K. Thallapally and D. Strachan, Langmuir, 2012, 28, 11584–11589 CrossRef CAS PubMed.
- M. H. Mohamed, S. K. Elsaidi, T. Pham, K. A. Forrest, H. T. Schaef, A. Hogan, L. Wojtas, W. Xu, B. Space, M. J. Zaworotko and P. K. Thallapally, Angew. Chem., Int. Ed., 2016, 55, 8285–8289 CrossRef CAS PubMed.
- Q. Wang, S. S. Xiong, Z. H. Xiang, S. M. Peng and D. P. Cao, Sci. China: Chem., 2016, 59, 643–650 CrossRef CAS.
- Q. Wang, H. Wang, S. M. Peng, X. Peng and D. P. Cao, J. Phys. Chem. C, 2014, 118, 10221–10229 CAS.
- S. K. Elsaidi, M. H. Mohamed, C. M. Simon, E. Braun, T. Pham, K. A. Forrest, W. Xu, D. Banerjeer, B. Space, M. J. Zaworotko and P. K. Thallapally, Chem. Sci., 2017, 8, 2373–2380 RSC.
- R. S. Patil, D. Banerjee, C. M. Simon, J. L. Atwood and P. K. Thallapally, Chem.–Eur. J., 2016, 22, 12618–12623 CrossRef CAS PubMed.
- S. K. Ghose, Y. Li, A. Yakovenko, A. Yakovenko, E. Dooryhee, L. Ehm, L. E. Ecker, A. Dippel, G. J. Halder, D. M. Strachan and P. K. Thallapally, J. Phys. Chem. Lett., 2015, 6, 1790–1794 CrossRef CAS PubMed.
- J. Liu, C. A. Fernandez, P. F. Martin, P. K. Thallapally and D. M. Strachan, Ind. Eng. Chem. Res., 2014, 53, 12893–12899 CrossRef CAS.
- D. Banerjee, A. J. Cairns, J. Liu, R. K. Motkuri, S. K. Nune, C. A. Fernandez, R. Krishna, D. M. Strachan and P. K. Thallapally, Acc. Chem. Res., 2015, 48, 211–219 CrossRef CAS PubMed.
- H. Wang, K. Yao, Z. Zhang, J. Jagiello, Q. Gong, Y. Han and J. Li, Chem. Sci., 2014, 5, 620–624 RSC.
- P. K. Thallapally, J. W. Grate and R. K. Motkuri, Chem. Commun., 2012, 48, 347–349 RSC.
- J. Liu, D. M. Strachan and P. K. Thallapally, Chem. Commun., 2014, 50, 466–468 RSC.
- L. Chen, P. S. Reiss, S. Y. Chong, D. Holden, K. E. Jelfs, T. Hasell, M. A. Little, A. Kewley, M. E. Briggs, A. Stephenson, K. M. Thomas, J. A. Armstrong, J. Bell, J. Busto, R. Boel, J. Liu, D. M. Strachan, P. K. Thallapally and A. I. Cooper, Nat. Mater., 2014, 134, 18892–18895 Search PubMed.
- S. S. Xiong, Q. Liu, Q. Wang, W. Li, Y. Tang, X. L. Wang, S. Hu and B. L. Chen, J. Mater. Chem. A, 2015, 3, 10747–10752 CAS.
- D. Banerjee, C. M. Simon, A. M. Plonka, R. K. Motkuri, J. Liu, X. Chen, B. Smit, J. B. Parise, M. Haranczyk and P. K. Thallapally, Nat. Commun., 2016, 7, 11831 CrossRef PubMed.
- B. J. Sikora, C. E. Wilmer, M. L. Greenfield and R. Q. Snurr, Chem. Sci., 2012, 3, 2217–2223 RSC.
- J. J. Perry, S. L. Teich-McGoldrick, S. T. Meek, J. A. Greathouse, M. Haranczyk and M. D. Allendorf, J. Phys. Chem. C, 2014, 118, 11685–11698 CAS.
- X. Chen, A. M. Plonka, D. Banerjee, R. Krishna, H. T. Schaef, S. Ghose, P. K. Thallapally and J. B. Parise, J. Am. Chem. Soc., 2015, 137, 7007–7010 CrossRef CAS PubMed.
- S. T. Meek, S. L. Teich-McGoldrick, J. J. Perry, J. A. Greathouse and M. D. Allendorf, J. Phys. Chem. C, 2012, 116, 19765–19772 CAS.
- S. K. Ghose, Y. Li, A. Yakovenko, E. Dooryhee, L. Ehm, L. Ecker, A.-C. Dippel, G. J. Halder, D. M. Strachan and P. K. Thallapally, J. Phys. Chem. Lett., 2015, 6, 1790–1794 CrossRef CAS PubMed.
- S.-T. Zheng, J. T. Bu, Y. F. Li, T. Wu, F. Zuo, P. Y. Feng and X. H. Bu, J. Am. Chem. Soc., 2010, 132, 17062–17064 CrossRef CAS PubMed.
- F. Nouar, J. Eckert, J. F. Eubank, P. Forster and M. Eddaoudi, J. Am. Chem. Soc., 2009, 131, 2864–2870 CrossRef CAS PubMed.
- T. Vazhappilly, T. K. Ghanty and B. N. Jagatap, J. Phys. Chem. C, 2016, 120, 10968–10974 CAS.
- S. J. Lee, K. C. Kim, T. U. Yoon, M. B. Kim and Y. S. Bae, Microporous Mesoporous Mater., 2016, 236, 284–291 CrossRef CAS.
- A. L. Myers and J. M. Prausnitz, AIChE J., 1965, 11, 121–127 CrossRef CAS.
- R. Babarao, Z. Q. Hu, J. W. Jiang, S. Chempath and S. I. Sandler, Langmuir, 2007, 23, 659–666 CrossRef CAS PubMed.
- S. R. Challa, D. S. Sholl and J. K. Johnson, J. Chem. Phys., 2002, 116, 814–824 CrossRef CAS.
- L. Czepirski and J. Jagiello, Chem. Eng. Sci., 1989, 44, 797–801 CrossRef CAS.
Footnote |
† Electronic supplementary information (ESI) available. See DOI: 10.1039/c7ra10538j |
|
This journal is © The Royal Society of Chemistry 2017 |
Click here to see how this site uses Cookies. View our privacy policy here.