DOI:
10.1039/C7RA10438C
(Paper)
RSC Adv., 2017,
7, 51670-51677
Li4x/3Co2−2xTi1+2x/3O4 spinel solid solutions: order and disorder phase transition, cations distribution and adjustable microwave dielectric properties
Received
20th September 2017
, Accepted 28th October 2017
First published on 8th November 2017
Abstract
A series of spinel solid solutions Li4x/3Co2−2xTi1+2x/3O4 (LCT, 0.2 ≤ x ≤ 0.8) were synthesized and their structures and microwave dielectric properties were characterized in detail. The distribution of cations at A and B sites in the lattice has been analyzed by using Rietveld refinement. The microstructure and dielectric properties were studied by scanning electron microscope and microwave network analyzer. The solid solutions undergo a discontinuous B-site, Li/Ti order–disorder phase transition from a disordered cubic phase to an ordered cubic phase with increasing x values from 0.4 to 0.6. With increasing the A-site lithium content, the LCT ceramics exhibit improved dielectric permittivity εr (20.3–26.5), high Q × f value (≥29
400 GHz) and a tailored temperature coefficient of resonant frequency τf from −40 to 10 ppm per °C. Optimized microwave dielectric properties were achieved for the composition with x = 0.8: εr = 26.5, Q × f ≈ 29
400 GHz and τf ≈ 10 ppm per °C. A correlation between the cation distribution and microwave dielectric loss is discussed in detail.
1. Introduction
The development of miniature, lightweight and low cost microwave devices advanced dielectric materials with excellent dielectric properties (high quality factor (Q × f), near-zero temperature coefficient of resonant frequency (τf) and moderate relative permittivity), and low bulk density.1–3 Some commercial materials, such as Ba(Mg1/3Ta2/3)O3 (BMT) and Ba(Zn1/3Ta2/3)O3 (BZT),4,5 possess a high quality factor (Q × f > 150
000), but high density and expensive cost restrict their applications in microwave devices. Other materials, such as (Zr1−xSnx)TiO4, BaTi4O9, Ba2Ti9O20, are low cost materials,6–8 however, a high sintering temperature together with a long annealing time is necessary to obtain densified ceramics. So, the search for new microwave dielectric materials with excellent properties, low cost and bulk density is in rapid progress owing to the drive for further system miniaturization.9–11
The spinel compounds have a general formulation of AB2O4 which crystallize in the cubic (isometric) crystal system. For this structure, the oxide anions are arranged in a cubic close-packed lattice and the cations A and B occupy some or all of the octahedral and tetrahedral sites in the lattice. A and B can be divalent, trivalent, or quadrivalent cations, including Mg, Zn, Fe, Mn, Al, Cr, Ti, and Si. Many spinel compounds have been widely investigated due to their outstanding magnetism (such as CoFe2O4, Ni0.5Zn0.5Fe2O4, ZnFe2O4, etc.)12–14 and electrochemical properties (such as LiMn2O4, LiCo0.5Mn1.5O4, Li4Ti5O12, etc.).15–17 Some compounds, such as ZnAl2O4, MgAl2O4, Mg2TiO4, etc. also exhibited perfect microwave dielectric performance,18–20 however, high sintering temperature restricted their further applications. Recently, the microwave dielectric properties of Li-based spinel compounds Li2MTi3O8 (M = Zn, Mg) have been reported by Sebastian et al.,21 Zhou et al.22 These materials not only showed excellent microwave properties, but also exhibited low sintering temperature, cheap raw materials cost and low bulk density. Antic et al.23 reported that a complete range of spinel-like solid solutions Li4x/3Co2−2xTi1+2x/3O4 (0 ≤ x ≤ 1) forms between Li4Ti5O12 and Co2TiO4. Fang and Zhou24,25 reported that two intermediate compounds LiCo0.5Ti1.5O4 and Li2/3CoTi4/3O4 exhibit good microwave dielectric properties, cheap cost and bulk density. However, the relationship between the structure and microwave dielectric properties of Li4x/3Co2−2xTi1+2x/3O4 solid solutions has not been well-established. In this work, Li4x/3Co2−2xTi1+2x/3O4 spinel series compounds were synthesized. The phase structure, cation distribution, order–disorder phase transition, microstructure and dielectric properties of these ceramics have also been investigated. Finally, correlations between the cation distribution and microwave dielectric loss are also addressed.
2. Experimental procedure
Specimens of the Li4x/3Co2−2xTi1+2x/3O4 (LCT, 0.2 ≤ x ≤ 0.8) ceramics were prepared by the conventional mixed oxide route from the high-purity raw powders (≥99%, Sinopharm Chemical Reagent Co. Ltd, Shanghai, China) of Li2CO3, Co2O3 and TiO2. The stoichiometric proportions of the above raw materials were mixed in the high-purity alcohol medium using zirconia balls for 4 h. The mixtures were dried and calcined at 900 °C for 6 h in air. With subsequent ball-milling, the powders were mixed with 5 wt% polyvinyl alcohol and pressed in to pellets of 12 mm in diameter and 6–7 mm in height by uniaxial pressing under a pressure of 200 MPa. The pellets were heat-treated at 550 °C for 4 h to remove the organic binder and then sintered at 1050–1150 °C for 2 h with a heating rate of 5 °C min−1 in air.
The crystal structures of the specimens were analyzed by an X-ray diffractometer (CuKα1, 1.54059 Å, Model X'Pert PRO, PANalytical, Almelo, Holland) with CuKα radiation generated at 40 kV and 100 mA. Data of a quality suitable for Rietveld refinement were collected over a 3 h range of 10–120°. Structural analysis has been done using Rietveld refinement program Topas 4.1. As 0.2 ≤ x ≤ 0.4, the parameters of Co2TiO4 were chose as a starting model. But when 0.6 ≤ x ≤ 0.8, the starting model was Li2CoTi3O8. It should be noted here that in order to avoid changing the multiplicity, the Wyckoff sites 8a and 16d with specific site symmetry in the structure with space group Fd
m were fixed and not refined, neither for the sites 4b, 8c, 12d, and 24e sites with specific symmetry in the P4332 space group. The bulk densities of the sintered samples were measured by the Archimedes method. After polishing and thermally etching, the samples were coated with gold and performed using a field emission scanning electron microscope (FESEM, JEOL JSM-7600F). Raman spectra were collected with a WITEC CRM200 Raman System (488 nm laser, 2.54 eV, WITec, Germany). Dielectric behaviors in microwave frequency were measured by the TE01δ shielded cavity method using a Network Analyzer (Model N5230A, Agilent Co., Palo Alto, California) and a temperature chamber (Delta 9039, Delta Design, San Diego, California). The temperature coefficient of resonant frequency (τf) was calculated by the formula as following:
|
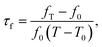 | (1) |
where
fT,
f0 were the resonant frequencies at the measuring temperature
T (85 °C) and
T0 (25 °C) respectively.
3. Results
3.1 Cations distribution and crystal structure
The phase structure and cation distribution of Li4x/3Co2−2xTi1+2x/3O4 (LCT) ceramics have been investigated by XRD refinement. Some of the intensities are not fit particularly well, this may mainly results from the deviation between the nominal and refined composition, as it is indeed difficult to get an accurate position and ideal occupancy for Li atoms from XRD data due to its low scattering factor to the X-ray. As to a Rietveld refinement based on powder XRD data (not single crystal refinements), several R-indices, such as Rwp, Rp, Rexp et al., are used to check on the quality of the fit, and refinement Rwp is statistically the most meaningful indicator of the overall fit since the numerator is the residual that is minimized in the least squares procedure. Here, although the GOF (= Rwp/Rexp) value seems a bit high, the considerable low Rwp values for all these Rietveld refinements make the fitting results acceptable and reliable. Fig. 1 shows the XRD patterns of all the products in Li4x/3Co2−2xTi1+2x/3O4 series. It can be seen that as the x values increased from 0.4 to 0.6, additional superstructural peaks appeared in the XRD patterns. All these reflections were indexed satisfactorily on the basis of a cubic unit cell [Co2TiO4 (PDF: 00-018-0428)] (Fig. 1(b)). The appearance of extra peaks agreed well with the previous report.22 In Leonidov's work,26 the reflections viz. (110), (210), (211), (221), and (310) which belong to superstructural peaks, appeared in XRD pattern due to the ordering of Li ion and Ti ion at octahedral site. Therefore, the sample, x = 0.6 exhibits cation ordering (Li
:
Ti = 1
:
3) on the octahedral sites, resulting in a superstructure that could be refined in the P4332 space group [Li2CoTi3O8 (PDF: 01-0891309)]. The crystallographic data for Li4x/3Co2−2xTi1+2x/3O4 compounds obtained by the Rietveld refinement are listed in Table 1. All the Bragg reflections were indexed on the basis of a cubic symmetry. With increasing the x values, the LCT samples undergo a disorder–order phase transition from the space group Fd
m to P4332. Antic et al.23 reported that Li4x/3Co2−2xTi1+2x/3O4 (0.5 ≤ x ≤ 0.875) exhibited 3
:
1 cation ordering with an ideal composition x = 0.75, i.e., Li2CoTi3O8. In our work, as the x value exceeds 0.6, the 1
:
3 ordered structure at the octahedral site is represented by lithium (4b) and titanium (12d), although the titanium sublattice contains some Co. The crystal structures of Li4x/3Co2−2xTi1+2x/3O4 series for x = 0.4 and x = 0.6, obtained from the Rietveld refinement data, are demonstrated in Fig. 2. It can be seen that as x = 0.4, the crystal was a disordered phase in which cations occupied 8a and 16d Wyckoff's positions. When the x = 0.6, the crystal became ordered phase and the cations occupied 8c, 4b, and 12d Wyckoff's positions. The Li at 4b position and Ti at 12d position formed a 1
:
3 order in the octahedral.
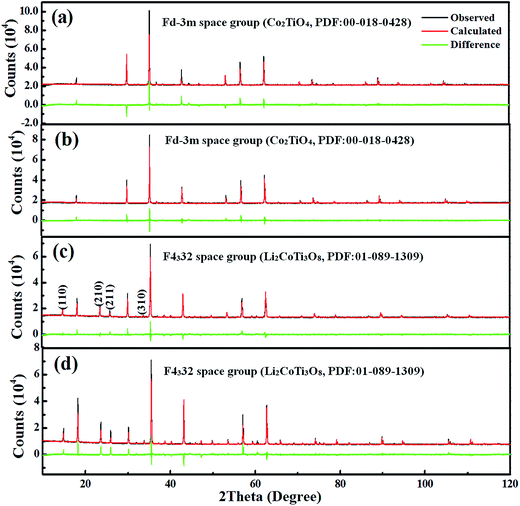 |
| Fig. 1 XRD refinement of Li4x/3Co2−2xTi1+2x/3O4 series: (a) x = 0.2 and (b) x = 0.4, were disordered phases, (c) x = 0.6 and (d) x = 0.8 were ordered phases. Indexed peaks are the superstructure of the ordered spinel phase. | |
Table 1 Crystal structure data and cation composition of Li4x/3Co2−2xTi1+2x/3O4 series
Atom |
Site |
x/a |
y/b |
z/c |
Occ |
Beq |
x = 0.2, (Li0.12Co0.87Ti0.01)(Li0.15Co0.73Ti1.12)O4, space group Fd m, disordered phase |
Co |
8a |
0 |
0 |
0 |
0.8738(3) |
0.6727(1) |
Li |
8a |
0 |
0 |
0 |
0.1162(3) |
0.6727(1) |
Ti |
8a |
0 |
0 |
0 |
0.01(1) |
0.6727(1) |
Co |
16d |
5/8 |
5/8 |
5/8 |
0.3832(2) |
0.6727(1) |
Li |
16d |
5/8 |
5/8 |
5/8 |
0.1508(3) |
0.6727(1) |
Ti |
16d |
5/8 |
5/8 |
5/8 |
1/2 |
0.6727(1) |
O |
32e |
0.3802(2) |
0.3802(2) |
0.3802(2) |
1 |
1 |
Rexp = 0.92, Rwp = 4.17, Rp = 1.74, GOF = 4.52, DW = 0.39, a = 8.4308(3) |
![[thin space (1/6-em)]](https://www.rsc.org/images/entities/char_2009.gif) |
x = 0.4, (Li0.16Co0.83Ti0.01)(Li0.37Co0.37Ti1.26)O4, space group Fd m, disordered phase |
Co |
8a |
0 |
0 |
0 |
0.8299(1) |
0.759(4) |
Li |
8a |
0 |
0 |
0 |
0.1601(5) |
0.759(4) |
Ti |
8a |
0 |
0 |
0 |
0.01(1) |
0.759(4) |
Co |
16d |
5/8 |
5/8 |
5/8 |
1/2 |
0.759(4) |
Ti |
16d |
5/8 |
5/8 |
5/8 |
0.3663(3) |
0.759(4) |
Li |
16d |
5/8 |
5/8 |
5/8 |
0.1337(2) |
0.759(4) |
O |
32e |
0.3802(2) |
0.3802(2) |
0.3802(2) |
1 |
1 |
Rexp = 0.75, Rwp = 1.62, Rp = 0.83, GOF = 2.15, DW = 0.95, a = 8.4137(4) |
![[thin space (1/6-em)]](https://www.rsc.org/images/entities/char_2009.gif) |
x = 0.6, (Li0.31Co0.69)(Li0.49Co0.11Ti1.4)O4, space group P4332, ordered phase |
Li |
4b |
5/8 |
5/8 |
5/8 |
0.9797(6) |
0.8899(2) |
Co |
8c |
0 |
0 |
0 |
0.6898(1) |
0.8899(2) |
Li |
8c |
0 |
0 |
0 |
0.3102(5) |
0.8899(2) |
Ti |
12d |
1/8 |
3/8 |
−1/8 |
0.952(3) |
0.8899(2) |
Co |
12d |
1/8 |
3/8 |
−1/8 |
0.048(2) |
0.8899(2) |
O |
24e |
1/8 |
1/8 |
3/8 |
1 |
1 |
O |
8c |
3/8 |
3/8 |
3/8 |
1 |
1 |
Rexp = 0.85, Rwp = 1.66, Rp = 0.98, GOF = 1.95, DW = 0.51, a = 8.3898(2) |
![[thin space (1/6-em)]](https://www.rsc.org/images/entities/char_2009.gif) |
x = 0.8, (Li0.6Co0.4)(Li0.47Ti1.53)O4, space group P4332, ordered phase |
Li |
4b |
5/8 |
5/8 |
5/8 |
0.8(1) |
1.239(6) |
Co |
8c |
0 |
0 |
0 |
0.4(1) |
1.239(6) |
Li |
8c |
0 |
0 |
0 |
0.6(1) |
1.239(6) |
Ti |
12d |
1/8 |
3/8 |
−1/8 |
1 |
1.239(6) |
O |
24e |
1/8 |
1/8 |
3/8 |
1 |
1 |
O |
8c |
3/8 |
3/8 |
3/8 |
1 |
1 |
Rexp = 1.09, Rwp = 3.86, Rp = 2.01, GOF = 3.55, DW = 0.38, a = 8.3748(3) |
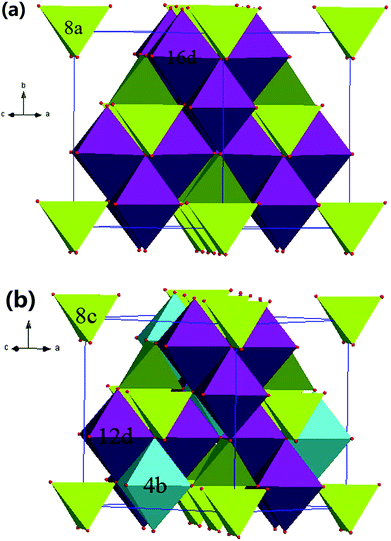 |
| Fig. 2 (a) Crystal structure of Li4x/3Co2−2xTi1+2x/3O4 series for x = 0.4, where 8a is the (Li0.16Co0.83Ti0.01)O4, 16d is the (Li0.37Co0.37Ti1.26)O6; (b) x = 0.6, where 8c is (Li0.31Co0.69)O4, 12d and 4b are the (Li0.49Co0.11Ti1.4)O6. | |
3.2 Raman analysis
Fig. 3 illustrates the Raman spectroscopy of Li4x/3Co2−2xTi1+2x/3O4 ceramics. Comparing with the powder XRD techniques, Raman spectroscopy reflects a short-range environment of the oxygen coordination around the cations in oxides or compounds. The positions and relative intensities of the Raman modes are sensitive to coordination geometry, oxidation states, and Jahn–Teller distortions.27,28 For the disordered spinel-type compounds (Fd
m space group), five optic modes are Raman active: 32e (O): A1g + Eg + 2F2g, 8a (cations): 1F2g. Ordering of the cations on octahedral sites will reduce the crystal symmetry from Fd
m to P4332. As a result, the number of Raman active modes will increase dramatically (5A1 + 12E + 17F2). Accordingly, atoms in all Wyckoff's position are Raman active: 8c: (1A1 + 2E + 3F2), 4b: (1E + 1F2), 24e: (3A1 + 6E + 9F2), 12d: (1A1 + 3E + 4F2). Therefore, the phase transition in Li4x/3Co2−2xTi1+2x/3O4 can be easily recognized from Raman spectra. As the x ≤ 0.4, three weak and broad bands around 350, 530, and 700 cm−1 could be observed. When the x value reaches 0.6, the Raman spectrum shows stronger and intense Raman bands at 710, 526, 404, 356 and 264 cm−1, which is probably the consequence of the 1
:
3 ordering of lithium and titanium in the octahedral sublattice. In Bouchard's work,29 Raman spectra of LiCo0.5Ti1.5O4 (x = 0.75) ordered spinel compound exhibit intense, broad bands at 700 and 400 cm−1 and minor bands at 528, 349 and 262 cm−1, which agree well with the results of the ordered samples for x = 0.6 and 0.8. The Raman active modes were assigned and the results were listed in Table 2.
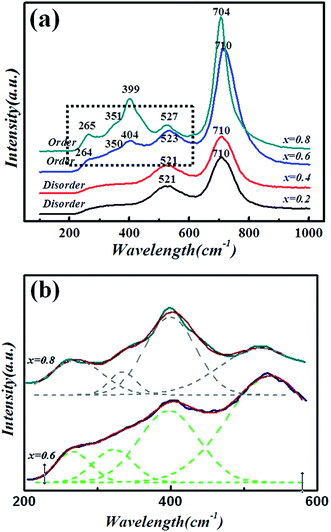 |
| Fig. 3 (a) Raman spectra of the Li4x/3Co2−2xTi1+2x/3O4 series, (b) high-resolution Roman spectra of Li4x/3Co2−2xTi1+2x/3O4 (x = 0.6 and 0.8) ceramics in the range of 200–600 cm−1. | |
Table 2 Raman active modes of Li4x/3Co2−2xTi1+2x/3O4 compounds
Li4x/3Co2−2xTi1+2x/3O4 |
Disordered cubic phase |
Ordered cubic phase |
Raman mode (cm−1) |
x = 0.2 |
x = 0.4 |
x = 0.6 |
x = 0.8 |
A1g |
710 |
710 |
710 |
704 |
F2g |
521 |
521 |
523 |
527 |
Eg |
— |
— |
404 |
399 |
F2g |
346 |
348 |
350 |
351 |
F2g |
— |
— |
264 |
265 |
3.3 Microstructure analysis
The surface images of the Li4x/3Co2−2xTi1+2x/3O4 ceramics have been examined by SEM and showed in Fig. 4. All samples exhibited uniform grain growth and few pores can be observed. The average grain size is about 50 μm, with reasonably good close packing of grains. Through enlarging some part of the grains, the step growth mechanism will be revealed [as seen in the inset of Fig. 4(a)].
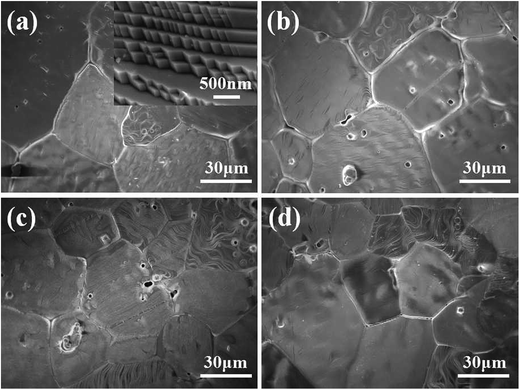 |
| Fig. 4 SEM of Li4x/3Co2−2xTi1+2x/3O4 ceramics: (a) x = 0.2, (b) x = 0.4, (c) x = 0.6 and (d) x = 0.8. Inset shows the enlarged SEM of (a). | |
3.4 Microwave dielectric properties
The microwave dielectric properties of Li4x/3Co2−2xTi1+2x/3O4 series compounds sintered at their optimized temperatures are listed in Table 3. With increasing x values, the optimized sintering temperature of solution decreased from 1125 to 1075 °C. The ceramics obtained a high relative density (over 95%). The permittivity was corrected by the Rushman–Strivens formula:30 εr.c. = εr.m. × (2 + P)/2(1 − P), where P is the porosity, εr.c. and εr.m. are the corrected permittivity and measured permittivity, respectively. The measured permittivity increased from 20.3 to 26.5, τf value improved from −40.1 to 10 ppm per °C and Q × f value deceased from 67
400 to 29
400 GHz. In a word, the ceramic for x = 0.8 exhibits good microwave dielectric properties with: εr.m. = 26.5, Q × f = 29
400 GHz, and τf = 10 ppm per °C (Table 3).
Table 3 The relative permittivity, Q × f and τf values of Li4x/3Co2−2xTi1+2x/3O4 series sintered their optimized temperature
Li4x/3Co2−2xTi1+2x/3O4 |
x = 0.2 |
x = 0.4 |
x = 0.6 |
x = 0.8 |
Sintering temperature (°C) |
1125 |
1100 |
1075 |
1075 |
Relative density (%) |
96 |
95.4 |
95.8 |
95.7 |
εr.m. |
20.3 |
20.9 |
24.8 |
26.5 |
εr.c. |
21.6 |
22.4 |
26.4 |
28.3 |
Q × f (GHz) |
67 400 |
65 300 |
43 300 |
29 400 |
f (GHz) |
7.81 |
7.32 |
7.08 |
6.27 |
τf (ppm per °C) |
−40.1 |
−32 |
−15.8 |
10 |
3.5 Infrared reflectivity
Fig. 5 presents the IR reflectivity spectra of Li4x/3Co2−2xTi1+2x/3O4 (x = 0.2 and 0.8) ceramics ranging from 50 to 1000 cm−1. It is seen that the infrared spectra were fitted by 7 resonant modes corresponding with the infrared reflectivity peaks and the reflectivity intensities of bands below 300 cm−1 become weaker for x = 0.2. For x = 0.8, the infrared spectra were fitted by 13 resonant modes and the bands above 700 cm−1 become weaker.
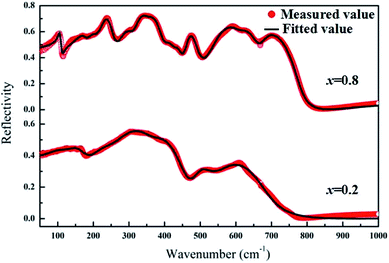 |
| Fig. 5 IR reflectivity spectra of Li4x/3Co2−2xTi1+2x/3O4 (x = 0.2 and 0.8) ceramics ranging from 50 to 1000 cm−1. | |
There spectra have been analyzed by using the classical harmonic oscillator model based on the standard Lorentzian formula [eqn (2)] and the Fresnel formula [eqn (3)]:
|
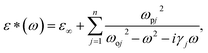 | (2) |
|
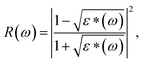 | (3) |
where
ε*(
ω) is complex dielectric function.
ε∞ is the dielectric constant caused by the electronic polarization at high frequencies.
ωpj,
ωoj and
γj are the plasma frequency, the transverse frequency, and damping factor of the
j-th Lorentz oscillator, respectively.
n is the number of transverse phonon modes.
R(
ω) is the IR reflectivity.
Fig. 6 shows the calculated permittivity ε′(ω) and loss ε′′(ω) obtained from the fits of the infrared reflectivity together with the experimental microwave data. It is seen that the calculated permittivities are a little smaller than the measured ones in the microwave range. Meanwhile, the calculated and measured dielectric losses have the same order of magnitudes. Therefore, it can be concluded that the microwave dielectric properties of ceramics are mainly caused by the polar optical phonons.
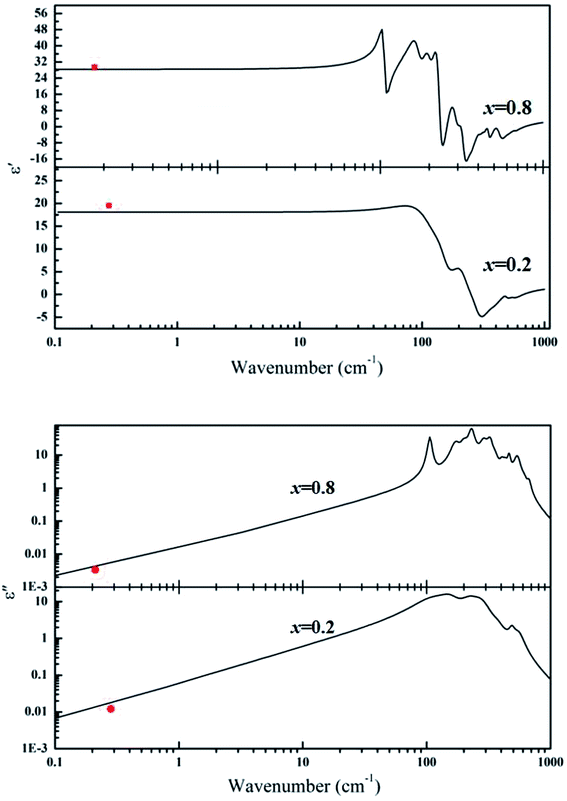 |
| Fig. 6 The calculated permittivity ε′(ω) and loss ε′′(ω) obtained from the fits of the infrared reflectivity together with the experimental microwave data (the red dots represent the experimental values that measured by using TE01δ method). | |
4. Discussion
For the Li-based spinel compounds, the cations distribution in the octahedral and tetrahedral plays an important role on tailoring the properties. Fig. 7(a) illustrates the relationship between the ionic polarizability per unit cell volume (αv) and relative permittivity. The variation of relative permittivity through the compositional evolution is dependent on the ionic polarizability per unit cell volume (αv).31 The αv increased from 0.1488 to 0.1536 with increasing x values, which results the increase of relative permittivity. The τf values increased from −40 to 10 ppm per °C with increasing x values. The behavior of the change of τf in this solid solution is similar to K2NiF4 type ceramic SrLaAlO4–Sr2TiO4 system.31 The origin of τf is related to linear expansion coefficient αL and dielectric constant variation with temperature (τε). Mathematically, the relationship is: |
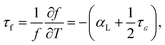 | (4) |
where τε is the temperature coefficient of the permittivity and αL is the linear thermal expansion coefficient of the dielectric material which is usually positive. For most of the electronic ceramic materials, αL is about +10 ppm per °C, indicating the significant influence of τε on τf. εr increases with increasing x value, the polarizability per unit cell volume increases. Meanwhile, the long range coupling of Ti4+ is improved through decreasing the Co2+ concentration. These effects drive τε more negatively, which in turn makes τf increase toward zero and positive further.
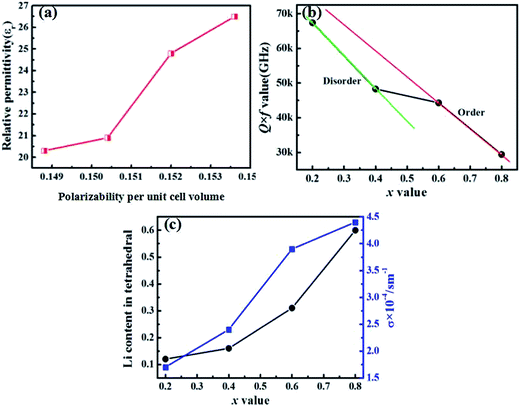 |
| Fig. 7 (a) Relative permittivity vs. polarizability per unit volume, (b) Q × f as a function of x value, and (c) σ and Li occupation in tetrahedral as a function of x value. | |
The Q × f values of Li4x/3Co2−2xTi1+2x/3O4 series ceramics decreased with increasing the x values [as shown in Fig. 7(b)]. The microwave dielectric losses included intrinsic loss which were mainly contributed by the lattice vibrational modes and extrinsic loss caused by densification/porosity, grain sizes, secondary phases and oxygen vacancies. For the Li4x/3Co2−2xTi1+2x/3O4 series ceramics, the changing Q × f values were related to the lattice vibrational loss and conductivity loss. From the XRD analysis, as the x values increase from 0.4 to 0.6, the crystal system changed from a disordered to ordered phase, indicating the increase of Q × f values. For the same composition for LCT compounds, an ordered phase exhibits a higher Q × f value than the disordered phase. The similar phenomenon was observed in Ba3MM′2O9 (M = Mg, Ni, Zn; M′ = Nb, Ta) perovskite compounds.5,32 Fig. 7(c) illustrated the relationship between the conductivity, lithium content in tetrahedral and x values. It can be seen that the conductivity and lithium content in tetrahedral increased with increasing x values. Higher lithium content in tetrahedral induced a higher conductivity loss. As a result, the Q × f values decreased. Besides, the atoms must overcome much force because of the shrinkage of the cell. These two factors induced the higher dielectric loss for the Li4x/3Co2−2xTi1+2x/3O4 series compounds. Li4x/3Co2−2xTi1+2x/3O4 exhibited good microwave dielectric performances, but high sintering temperature (1075–1125 °C) limit their further industrial applications, we will try to reduce the sintering temperature of Li4x/3Co2−2xTi1+2x/3O4 ceramics in the next work.
5. Conclusions
In this paper, Li4x/3Co2−2xTi1+2x/3O4 spinel series compounds were synthesized. The phase structure, cation distribution, order–disorder phase transition, microstructure and dielectric properties of these ceramics have also been investigated. Structural analysis showed that the Li4x/3Co2−2xTi1+2x/3O4 series undergo a discontinuous phase transition from a disordered cubic phase to an ordered cubic phase with the changing x value. Li4x/3Co2−2xTi1+2x/3O4 ceramics exhibit relatively high dielectric permittivity εr of 20.3–26.5, modest Q × f values of 29
400–67
400 GHz and temperature coefficient of resonant frequency τf from −40 ppm per °C to 10 ppm per °C. Finally, correlations between the cation distribution and microwave dielectric loss are also addressed.
Conflicts of interest
There are no conflicts to declare.
Acknowledgements
This work was supported by the Natural Science Foundation of China (No. 11464009, 61761015, 11664008 and 11364012), Guangxi Natural Science Foundation for Distinguished Young Scholars (Basic research on microwave dielectric ceramics and devices for novel nonferrous metal oxides), Guangxi Natural Science Foundation (No. 2015GXNSFDA139033) and Research Start-up Funds Doctor of Guilin University of Technology (No. 002401003281 and 002401003282).
References
- D. Zhou, D. Guo, W. B. Li, L. X. Pang, X. Yao, D. W. Wang and I. M. Reaney, J. Mater. Chem. C, 2016, 4, 5357 RSC.
- B. Liu, L. Li, X. Q. Liu and X. M. Chen, J. Mater. Chem. C, 2016, 4, 4684 RSC.
- J. Guo, C. A. Randall, G. Q. Zhang, D. Zhou, Y. Y. Chen and H. Wang, J. Mater. Chem. C, 2014, 2, 7364 RSC.
- K. P. Surendran, M. T. Sebastian, P. Mohanan, R. L. Moreira and A. Dias, Chem. Mater., 2005, 17, 142 CrossRef CAS.
- M. W. Lufaso, Chem. Mater., 2004, 16, 2148 CrossRef CAS.
- K. Wakino, K. Minai and H. Tamura, J. Am. Ceram. Soc., 1984, 67, 278 CrossRef CAS.
- S. G. Mhaisalker, D. W. Readey and S. A. Akbar, J. Am. Ceram. Soc., 1991, 74, 1894 CrossRef.
- S. F. Wang, Y. F. Hsu, T. H. Weng, C. C. Chiang, J. P. Chu and C. Y. Huang, J. Mater. Res., 2003, 18, 1179 CrossRef CAS.
- D. Zhou, L. X. Pang, J. Guo, H. Wang, X. Yao and C. Randall, Inorg. Chem., 2011, 50, 12733 CrossRef CAS PubMed.
- J. J. Bian and J. Y. Wu, J. Am. Ceram. Soc., 2012, 95, 318 CrossRef CAS.
- C. L. Huang, Y. W. Tseng and J. Y. Chen, J. Eur. Ceram. Soc., 2012, 32, 3287 CrossRef CAS.
- H. Zheng, J. Wang, S. E. Lofland, Z. Ma, L. Mohaddes-Ardabili, T. Zhao, L. Salamanca-Riba, S. R. Shinde, S. B. Ogale, F. Bai, D. Viehland, Y. Jia, D. G. Schlom, M. Wuttig, A. Roytburd and R. Ramesh, Science, 2004, 303, 661 CrossRef CAS PubMed.
- I. Ismayadi, M. Hashim, A. M. Khamirul and R. Alias, Am. J. Appl. Sci., 2009, 6, 1548 CrossRef CAS.
- C. Yao, Q. Zeng, G. F. Goya, T. Torres, J. Liu, H. Wu, M. Ge, Y. Zeng, Y. Wang and J. Z. Jiang, J. Phys. Chem. C, 2007, 111, 12274 CAS.
- S. H. Guo, S. C. Zhang, X. M. He, W. H. Pu, C. Y. Jiang and C. R. Wan, J. Electrochem. Soc., 2008, 155, A760 CrossRef CAS.
- A. R. West, H. Kawai, H. Kageyama, M. Tabuchi, M. Nagata and H. Tukamoto, J. Mater. Chem., 2001, 11, 1662 RSC.
- G. J. Wang, J. Gao, L. J. Fu, N. H. Zhao, Y. P. Wu and T. Takamura, J. Power Sources, 2007, 174, 1109 CrossRef CAS.
- W. Lei, W. Z. Lu, J. H. Zhu and X. H. Wang, Mater. Lett., 2007, 61, 4066 CrossRef CAS.
- K. P. Surendran, P. V. Bijumon, P. Mohanan and M. T. Sebastian, Appl. Phys. A, 2005, 81, 823 CrossRef CAS.
- C. L. Huang and J. Y. Chen, J. Am. Ceram. Soc., 2009, 92, 675 CrossRef CAS.
- S. George and M. T. Sebastian, J. Am. Ceram. Soc., 2010, 93, 2164 CrossRef CAS.
- H. F. Zhou, X. L. Chen, L. Fang and H. Wang, J. Mater. Res., 2010, 25, 1235 CrossRef CAS.
- B. Antic, G. F. Goya, V. Kusigerski, N. Jovic, M. Mitric and H. R. Rechenberg, J. Phys.: Condens. Matter, 2004, 16, 651 CrossRef CAS.
- L. Fang, D. Chu, H. Zhou, X. Chen and Z. Yang, J. Alloys Compd., 2011, 509, 1880 CrossRef CAS.
- H. F. Zhou, X. B. Liu, X. L. Chen and L. Fang, Mater. Res. Bull., 2012, 47, 1278 CrossRef CAS.
- I. Leonidov, N. Leonidova, R. F. Samigullina and V. Patrakeev, J. Struct. Chem., 2004, 45, 262 CrossRef CAS.
- C. M. Julien, F. Gendron, A. Amdouni and M. Massot, Mater. Sci. Eng., B., 2006, 130, 41 CrossRef CAS.
- N. Jovic, M. Vucinic-Vasic, A. Kremenovic, B. Antic, C. Jovalekic, P. Vulic, V. Kahlenberg and R. Kaindl, Mater. Chem. Phys., 2009, 116, 542 CrossRef CAS.
- M. Bouchard and A. Gambardella, J. Raman Spectrosc., 2010, 41, 1477 CrossRef.
- D. F. Rushman and M. A. Strivens, Proc. Phys. Soc., 1947, 59, 1011 CrossRef CAS.
- M. M. Mao, X. M. Chen and X. Q. Liu, J. Am. Ceram. Soc., 2011, 94, 3948 CrossRef CAS.
- M. Bieringer, S. M. Moussa, L. D. Noailles, A. Burrows, C. J. Kiely, M. J. Rosseinsky and R. M. Ibberson, Chem. Mater., 2003, 15, 586 CrossRef CAS.
|
This journal is © The Royal Society of Chemistry 2017 |