DOI:
10.1039/C7RA09671B
(Paper)
RSC Adv., 2017,
7, 45834-45842
Hydrothermal synthesis of narrow-band red emitting K2NaAlF6:Mn4+ phosphor for warm-white LED applications
Received
31st August 2017
, Accepted 20th September 2017
First published on 25th September 2017
Abstract
A series of Mn4+ activated aluminofluoride (K2NaAlF6) red phosphors were synthesized via a hydrothermal route. The structure, morphology and composition were characterized by X-ray diffraction (XRD), scanning electron microscopy (SEM) and energy-dispersive X-ray spectrometry (EDX). The photoluminescence properties were investigated by using emission and excitation spectra, temperature dependent luminescence spectra and decay curves. The obtained K2NaAlF6:Mn4+ can emit red light peaking at 633 nm under 460 nm excitation. The critical quenching concentration of Mn4+ was about 1%. The changes in Mn4+ emissions based on different ratios of KF to NaF, reaction temperature and reaction time were investigated in detail. Concentration and thermal quenching mechanisms were elucidated systematically. The white light-emitting diodes (WLED) fabricated with the as-prepared phosphor exhibit a low color temperature (4310 K), higher color rendering index (Ra = 78.7) and luminous efficacy of 60.22 lm W−1. The inherent advantage of K2NaAlF6:Mn4+ makes it a promising red phosphor for future WLED.
1 Introduction
In recent years, white light-emitting diodes (WLED), which are considered as energy-saving light source, have attracted more and more attention owing to their long operational life time, environmentally friendly properties and stability.1–5 Currently, the white light is mainly produced via a combination of leaked blue emission from an InGaN chip and the broad green-yellow emission of yellow phosphor Y3Al5O12:Ce3+ (YAG:Ce3+).6–8 However, this approach limits the application range to cool white light with poor color rendering and a correlated higher colour temperature of approximately 4500–8000 K.9,10 The innate deficiency in the red spectral region reduces the suitability for indoor illumination. It is therefore logical to supplement a red phosphor into this system to improve the color rendering. This idea has stimulated research on red phosphors activated by rare earth or transition metal ions.
Among the available red phosphors, Eu2+ or Ce3+ ions doped nitrides are the most widely known, such as Sr2Si5N18:Eu2+, CaAlSiN3:Eu2+ and M2Si5N8:Ce3+ (M = Ca, Sr, Ba).11–13 Eu2+ doped nitrides and oxides have the advantages of high quantum efficiency and good thermal stability. However, the preparation process and reaction conditions of nitrides phosphors are rigorous.14–16 These shortcomings limit the application in white LEDs. Compared with the rare earth ions doped nitrides red phosphor, tetravalent manganese ions (Mn4+) doped fluoride or oxide phosphors exhibit a series of obvious advantages.17,18 First of all, Mn4+ ions display the characteristics of narrow emission and high color purity, which overcome the defect of broad emission bands and serious photon reabsorption in Eu2+ doped nitrides phosphors.19 In addition, the low price of transition metals effectively reduces the producing cost of LEDs. It is another important advantage that the Mn4+ ions activated phosphors can be excited by blue light chip. At present, Mn4+ doped oxide phosphors have attracted much attention.20–22 The Jin's team reported a class of Mn4+ doped SrGe4O9 red phosphors by conventional solid-state reaction.23 The Mn4+ can replace Ge4+ sites in the host lattice owing to the same ionic radius of Mn4+ and Ge4+. SrGe4O9:Mn4+ phosphors exhibit red emission under excitation of 276 nm and 430 nm. In general, the near ultraviolet absorption peaks of Mn4+ doped oxide phosphors are higher than those of blue light, this property also limits the use of blue light chip. Therefore, numerous researchers have devoted their effort to investigate Mn4+-substituted fluoride phosphor containing AF62− (A = Ti4+, Ge4+, Si4+, Sn4+, Zr4+) octahedral environment.24,25 The outer 3d3 electron configurated Mn4+ activated phosphor shows two intense broad absorption bands ranged from 300 to 500 nm, attributing to spin-allowed 4A2g → 4T1g and 4A2g → 4T2g transition.26,27 The red emission near 600–700 nm under blue light excitation originates from the spin-forbidden 2Eg → 4A2g transition.28 For example, Zhou and his co-authors synthesized an efficient red phosphor BaGeF6:Mn4+ by hydrothermal method and obtained the optimized reaction conditions.29 In addition, BaGeF6:Mn4+ is favourable for decreasing correlated color temperature (CCT) in WLED devices. Nguyen's group reported a co-precipitation method to produce a class of Na2SiF6:Mn4+ red phosphor using NaOH/NaMnO4 as starting materials in SiF62−/HF system.30 The result displayed the relative luminescence intensity maintained to 92% at 423 K than that at room temperature. Recently, Zhu's group31 had successfully prepared novel red-emitting phosphors K2LiGaF6:Mn4+ with excellent thermal stability and luminescent properties, it was proved to be a potential commercial phosphor used in warm white LED devices. Huang's group32 had synthesized Mn4+ doped K2SiF6 phosphors using a novel HF-free hydrothermal synthetic. The synthetic route was more environmental friendly and the reaction mechanism of K2SiF6:Mn4+ was discussed in detail. According to the previous studies, it is not difficult to find that Mn4+ doped oxide or fluoride phosphors can exhibit red emission and effectively improve the performance of LEDs. K2NaAlF6 is a common elpasolite material. The crystal structure of K2NaAlF6 shows that Al3+ is surrounded by six F− ions. The AlF63− octahedron environment is beneficial for the emission of Mn4+.33 Furthermore, the ionic radius of Al3+ and Mn4+ are similar. Mn4+ ions may be achieved by replacing Al3+ after entering the crystal lattice. Thus, we believe that K2NaAlF6 can be used as a suitable matrix for luminescent materials.
In this paper, we report a kind of elpasolite structure red phosphor K2NaAlF6:Mn4+. The crystal structure and luminescence properties are investigated. Al3+ ions can be substituted by Mn4+ ions because of the smaller ionic radius. Meanwhile, the influences of synthesis conditions, such as raw material, reaction time, temperature and Mn4+ concentration on emission intensity have been discussed carefully in details. The experiment results show that K2NaAlF6:Mn4+ phosphor is a promising red phosphor for future WLED.
2 Experimental
2.1 Materials
All the chemical reagents in our experiment, including potassium fluoride (KF, >98%), sodium fluoride (NaF, >98%), aluminium fluoride (AlF3, >99%), hydrofluoric acid solution (HF, 40 wt%), potassium permanganate (KMnO4, >99.5%), potassium hydrogen difluoride (KHF2, >99%) and hydrogen peroxide aqueous solution (H2O2, 30.0%), were supplied by the Sinopharm Chemical Reagent Co. Ltd. All chemical reagents were used as obtained without further purification.
2.2 Preparation
Red phosphor K2NaAlF6:Mn4+ was prepared via a hydrothermal route with K2MnF6 as the Mn4+ source. The K2MnF6 crystals were prepared based on Bode's method.34 In a typical synthesis, AlF3 (0.2444 g) and K2MnF6 (0.0222 g) were added to the 20 mL 40 wt% HF solution in a 50 mL plastic breaker. The mixture was fully dissolved by magnetically stirring. Subsequently, NaF (0.1260 g) and KF (0.3487 g) were added into 20 mL distilled water until completely dissolved. Finally, a mixture of the NaF and KF was put into the above solution. After vigorous stirring for 30 min, the mixture solution was transferred into a 50 mL Teflon-lined autoclave and heated at 180 °C for 6 h, then cooled naturally to room temperature. The yellow solid products were collected by centrifugation, washed several times with distilled water and ethanol. The as-prepared products were dried for 12 h at 60 °C. Finally, the K2NaAlF6:Mn4+ red phosphor sample was obtained. The synthesis diagram of the preparation process is revealed in Fig. 1.
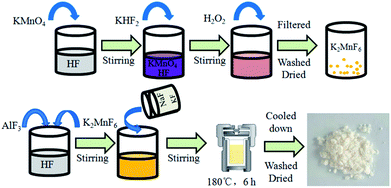 |
| Fig. 1 Synthesis diagram of K2NaAlF6:Mn4+ phosphors via a hydrothermal method. | |
2.3 Fabrication of LED devices
The WLED devices were fabricated by combining GaN chips (∼460 nm) with the mixture of obtained red phosphors K2NaAlF6:Mn4+ and yellow phosphor YAG:Ce3+. First, the phosphors were completely mixed with epoxy resin, and then the mixture was coated onto the superficies of the GaN chip. The device was packaged with epoxy resin and solidified at 140 °C for 12 h. The fabricated devices were used for subsequent testing.
2.4 Characterizations
The crystal structures of all samples were investigated using a RigakuD/max-RA X-ray diffractometer with Cu Kα radiation (λ = 0.15418 nm) in the 2θ range from 10° to 90°. The corresponding surface morphology and composition of the samples were examined by a FEI XL-30 field emission scanning electron microscope (FESEM) equipped with an attached energy dispersive X-ray (EDX) spectrometer. Photo-luminescence (PL), photoluminescence excitation (PLE) and luminescence decay curves of samples were measured on a HITACHI F-7000 fluorescence spectrophotometer equipped with a 150 W Xe lamp as the excitation source, scanning at 1200 nm min−1. The temperature dependent luminescence properties were examined using a JobinYvon fluoro Max-4 equipped with a 150 W xenon lamp as the excitation source. Quantum efficiency was also measured using a photoluminescence quantum efficiency measurement system (C9920-02, Hamamatsu Photonics K. K., Japan).
3 Results and discussion
3.1 Phase structure and morphology analysis
The phase purity and crystallinity of the as-prepared K2NaAlF6:Mn4+ samples were first characterized by XRD. Fig. 2(a) shows the XRD pattern of the K2NaAlF6:Mn4+ sample along with JCPDS card no. 22-1235 at the bottom. The diffraction peaks of the phosphors can be matched well with the standard pattern of pure K2NaAlF6. No extra peaks of impurity are detected in the pattern, indicating the doped Mn4+ ions do not change the crystal structure of K2NaAlF6. The K2NaAlF6 is crystallized in a cubic Fm
m space group with lattice parameter of a = b = c = 8.122 Å. Fig. 2(b) displays the crystal structure of the K2NaAlF6. Al3+ is coordinated with six F− to form a regular AlF63− octahedron. Mn4+ ions would prefer to replace Al3+ ions in octahedral sites. Because Mn4+ (0.53 Å, CN = 6) has highly close ionic radius with Al3+ (0.535 Å, CN = 6).
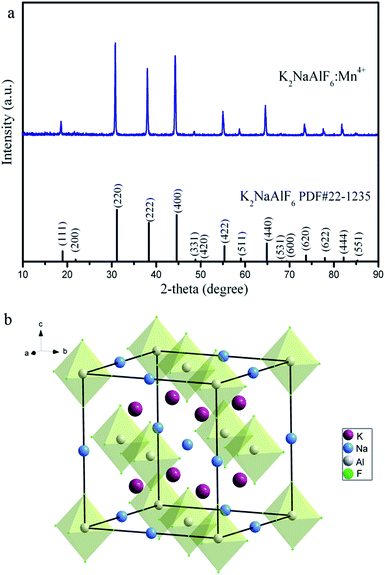 |
| Fig. 2 (a) XRD pattern of the as-synthesized K2NaAlF6:Mn4+ samples. The standard card (PDF#22-1235) is included for reference. (b) Schematic illustration of K2NaAlF6 crystal structure. | |
The morphology and composition of the as-prepared K2NaAlF6:Mn4+ were characterized by FESEM and EDX analysis, respectively. As show in Fig. 3(a), it can be seen that the as-prepared phosphor displays truncated octahedron morphology with smooth surface. A side length of truncated octahedron is about 1 μm. In the corresponding EDX spectrum (Fig. 3(b)), the results exhibit that the phosphors are composed of K, Na, Al, F and Mn element, respectively. The result also verifies that Mn element has been indeed doped into the lattice to occupy the Al lattice site. The detected C and Au element in Fig. 3(b) is ascribed to the conducting resin and metal spraying. The peak of the Si results from the silicon wafer used during the measurement.
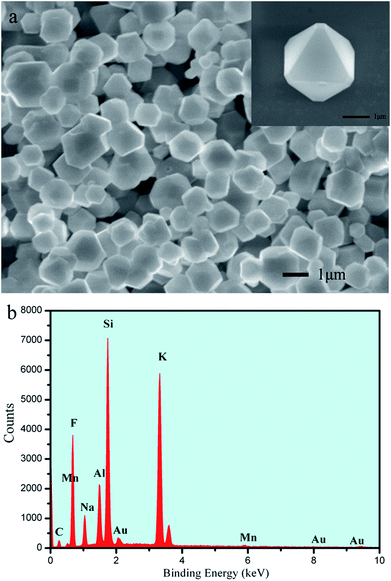 |
| Fig. 3 (a) SEM images (a) and EDX spectrum (b) of K2NaAlF6:3%Mn4+. | |
3.2 Luminescence properties of K2NaAlF6:Mn4+
To determine the luminescence behaviour of Mn4+ in K2NaAlF6, we choose the samples by hydrothermal treatment at 180 °C for 6 h as an example. Fig. 4(a) presents the typical excitation and emission spectra at room temperature. As the monitoring wavelength at 633 nm, the photoluminescence excitation spectrum of K2NaAlF6:Mn4+ could be recorded in the wavelength range of 300–550 nm. There are two excitation bands located in the near-UV and blue region. The shape and range of excitation spectrum are basically consistent with those reported in the literature.35 The weak excitation peak at 363 nm can be ascribed to the spin-allowed 4A2g → 4T1g transition of Mn4+ ions in K2NaAlF6. The dominating excitation peak at 460 nm is due to the transition from ground state 4A2g to 4T2g of Mn4+ ions. The results demonstrate the excitation of K2NaAlF6:Mn4+ can be well matched with the GaN blue chip and the prepared phosphors have a potential application for LEDs. Under excitation at 460 nm, the emission spectrum has series of narrow-line emission peaks ranged from 580 to 660 nm, with the maximum peak at 633 nm. The red emission line is attributed to 2Eg → 4A2g transition of Mn4+. The fluorescence decay curve for 2Eg → 4A2g of the Mn4+ ions in red phosphor K2NaAlF6:3%Mn4+ monitored at 633 nm with the excitation of 460 nm was measured at room temperature, as shown in Fig. 4(b). The decay curve can be well fitted to a single-exponential model by the following equation:
where I(t) is the luminescence intensity of K2NaAlF6:3%Mn4+ at time t, A is constant, I0 is the initial intensity and τ is the lifetime for exponential components. The fluorescence lifetime value is about 3.91 ms. Fig. 4(c) displays the CIE chromaticity diagram of K2NaAlF6:3%Mn4+. The K2NaAlF6:Mn4+ red phosphor has aquantum efficiency of 16%. The CIE chromaticity coordinates of as-prepared phosphor were calculated to be x = 0.6384, y = 0.3534, which is very close to the standard values of National Television Standard Committee (NTSC) (x = 0.67, y = 0.33). Therefore, K2NaAlF6:3%Mn4+ can exhibit a high red purity. The inset photographs in Fig. 4(c) represent the K2NaAlF6:3%Mn4+ phosphor in the daylight and blue light illumination, respectively. One can see obvious red emission light under blue light radiation.
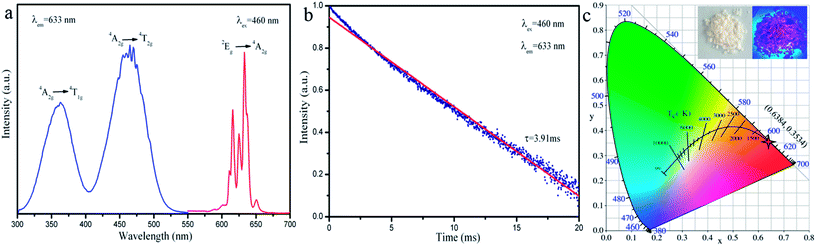 |
| Fig. 4 (a) Photoluminescence excitation (left) and emission (right) spectra, (b) decay curve of the K2NaAlF6:3%Mn4+ examined at room temperature by monitoring wavelength at 633 nm with a 460 nm light excitation and (c) CIE chromaticity coordinate of K2NaAlF6:3%Mn4+. The inset photographs represent the K2NaAlF6:3%Mn4+ phosphor in the daylight and under lamp (460 nm) irradiation. | |
The ratio of reactants is an important parameter for the phase purity and luminous intensity. In order to acquire better luminescent properties of the phosphors, we investigated the influence of different molar ratio of KF to NaF on crystal structure and luminescence properties. Fig. 5(a) shows the XRD patterns of K2NaAlF6:Mn4+ phosphors synthesized with different molar ratio of KF to NaF (1
:
1, 2
:
1, 4
:
1, 8
:
1, 12
:
1 and 20
:
1). When the molar ratio of KF to NaF is 1
:
1, the major diffraction peaks are close to the standard card PDF#22-1235. The three main diffraction peaks correspond to the lattice plane [220], [222] and [400], respectively, and the diffraction intensity is very close. However, a weak impurity peak also appears near the diffraction angle about 30.6°, which is accordance with the standard card no. 30-1144 (Na5Al3F14). The result implies that a part of impurity Na5Al3F14 can be generated in case of insufficient potassium source. When the molar ratio of KF to NaF gradually rises to 2
:
1–20
:
1, all the diffraction peaks of as-prepared samples can be in agreement with the JCPDS card no. 22-1235. No other impurity phases, such as Na5Al3F14 or Na3AlF6 are detected, indicating that the product is pure phase. Fig. 5(b) is the emission spectra of K2NaAlF6:Mn4+ prepared with different molar ratio of KF to NaF. It can be seen that all the PL spectra of K2NaAlF6:3%Mn4+ are almost identical except for the luminescence intensity. When molar ratio of KF to NaF is 1
:
1, the red emission intensity is weak. With increasing the mole ratio of KF to NaF from 2
:
1 to 12
:
1, the red emission can be enhanced, the red emission intensity of sample (KF
:
NaF = 12
:
1) is about 1.52 times higher than that of samples (KF
:
NaF = 2
:
1), suggesting that appropriate adding the ratio of KF to NaF into the reaction system may enhance the intensity of Mn4+ doped K2NaAlF6 phosphor. But further increasing the ratio of KF to NaF, a weakening trend of emission spectrum is observed, which might be due to excessive K+ exacerbated the charge imbalance when the tetravalent Mn4+ replaced the trivalent Al3+.
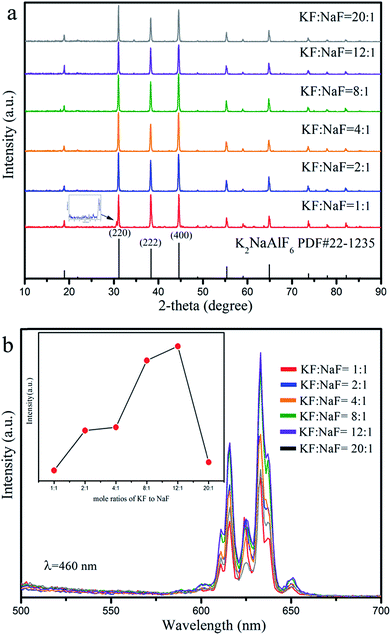 |
| Fig. 5 (a) XRD patterns and (b) emission spectra (λex = 460 nm) of the K2NaAlF6:Mn4+ phosphors synthesized with different molar ratios of KF to NaF (KF : NaF = 1 : 1, 2 : 1, 4 : 1, 8 : 1, 12 : 1 and 20 : 1). | |
In order to investigate the effect of the reaction temperature on the phase and photoluminescence properties of K2NaAlF6:Mn4+ phosphors. A series of K2NaAlF6:Mn4+ samples were synthesized at different temperatures for 6 h. Fig. 6(a) presents the XRD patterns of the phosphors obtained at different reaction temperatures. It is obviously seen that the diffraction peaks of these phosphors agree well with the cubic phase K2NaAlF6 [space group: Fm
m] (PDF#22-1235), without any impurity phase. The increasing of the reaction temperature did not cause the change of the crystal structure and phase purity. Fig. 6(b) displays a series of corresponding emission spectra of K2NaAlF6:Mn4+ prepared under the reaction temperatures from 25 °C to 180 °C. Noted that the emission spectra also share the similar shape. With increasing the reaction temperature from 25 °C to 100 °C, the emission intensity sharply increases. Improving the proper reaction temperature can be beneficial for Mn4+ into the lattice to substitute Al3+ sites. Further increasing reaction temperature, the luminescent intensity of the as-synthesized K2NaAlF6:Mn4+ phosphor decreases obviously under 460 nm blue light excitation. The possible reason is that the Mn4+ is not stable at very high temperature.
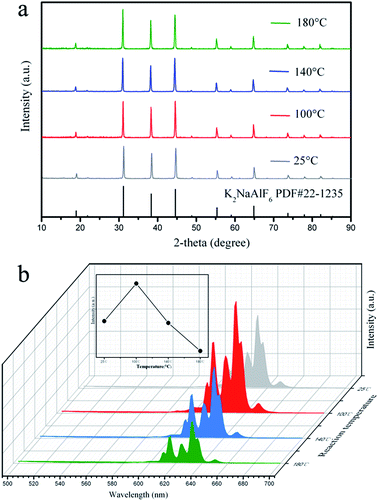 |
| Fig. 6 (a) XRD patterns and (b) emission spectra (λex = 460 nm) of the K2NaAlF6:Mn4+ samples synthesized at different reaction temperatures. | |
In addition to the molar ratio of raw materials and reaction temperature, the influence of reaction time on the luminescence of K2NaAlF6:Mn4+ was also discussed. Fig. 7(a) presents the XRD patterns of the products obtained by different hydrothermal reaction times. It demonstrates that all the diffraction peaks are consisted with the standard data (PDF#22-1235) of K2NaAlF6 with a cubic structure. The phase purity and crystal structure of samples exhibit no significant change with increasing reaction times. The emission spectra of K2NaAlF6:Mn4+ prepared at different reaction times are shown in Fig. 7(b). The K2NaAlF6:Mn4+ phosphors all display sharp red emission peaks covering from 550 nm to 700 nm with a maximum at 633 nm. It is clear that the emission intensity of as-prepared sample at 12 h is stronger than that of 6 h. As the reaction time further increases, the emission intensity of the K2NaAlF6:Mn4+ decreases. The phenomenon of emission intensity dropped with prolonging reaction time from 12 h to 36 h may be due to the Mn4+ ions in the phosphors tend to instability in a long period time.36 Therefore, fluorescence quenching results in lower luminous intensity.
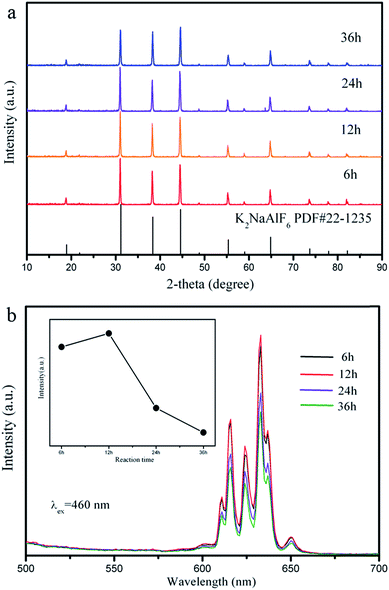 |
| Fig. 7 (a) XRD patterns and (b) emission spectra (λex = 460 nm) of the K2NaAlF6:Mn4+ samples synthesized at different reaction times. | |
The next part mainly concerns the influence of optical properties and structure for K2NaAlF6:Mn4+ prepared using various Mn4+ ions contents. As shown in Fig. 8(a), the XRD patterns of the obtained K2NaAlF6:xMn4+ (x = 0.5%, 1%, 3%, 5% and 7%) phosphors are collected to verify the phase purity. All the characteristic diffraction peaks are in good agreement with the corresponding standard card data of K2NaAlF6 (PDF#22-1235). The results show that the Mn4+ ions enter the lattice of K2NaAlF6 without altering the crystal structure. The high diffraction intensities indicate that the phosphors have good crystallinity. Fig. 8(b) shows the emission spectra of K2NaAlF6:Mn4+ with different Mn4+ doping concentrations. A series of emission peaks located at 611, 616, 625, 633 and 650 nm can be observed from the corresponding emission spectra of K2NaAlF6:Mn4+ under 460 nm blue light excitation. With increasing the concentration of Mn4+ ions, the emission intensity of as-prepared phosphor increases. When the manganese content reaches 1%, the sample obtains the strongest emission intensity. When further increasing Mn4+ ions concentration beyond 1%, the emission intensities of K2NaAlF6:Mn4+ have an obvious decreasing trend, which might be related to concentration quenching effect. Therefore, the optimum Mn4+ ions concentration in K2NaAlF6 host should be 1%, indicating that 1% is the quenching concentration in K2NaAlF6:Mn4+ phosphor. The phenomenon of the dropped emission intensity can be attributed to the occurrence of energy transfer within the adjacent Mn4+, which is finally end by a kill centre. Evidently, the energy transfer mechanism is not caused by radiation re-absorption. Because there is no overlap between PLE and PL spectra of K2NaAlF6:xMn4+. The exchange interaction or the dipole–dipole interaction may be responsible for the quenching. To clarify the point, the critical distance (Rc) was calculated with the following equation:37
where
V is the volume of unit cell,
xc means the critical concentration.
N is the number of the central cations in the unit cell. For K
2NaAlF
6:Mn
4+,
V = 534.99 Å,
xc = 0.01 and
N = 4. Therefore, the critical distance of
Rc is calculated to be 14.735 Å, which is larger than the typical critical distance (5 Å), so the possibility of exchange interaction is eliminated. The concentration quenching is related to multipolar energy transfer mechanism. According to the Dexter theory, the type of interaction between Mn
4+ ions can be determined by the equation
38 as follows:
where
I is the emission intensity at activator concentration
x,
K and
β are constants, the value of
θ = 6, 8, 10 represents the electric multipole index corresponding to the dipole–dipole (d–d), dipole–quadrupole (d–q) and quadrupole–quadrupole (q–q) interaction. The above equation can be predigested to
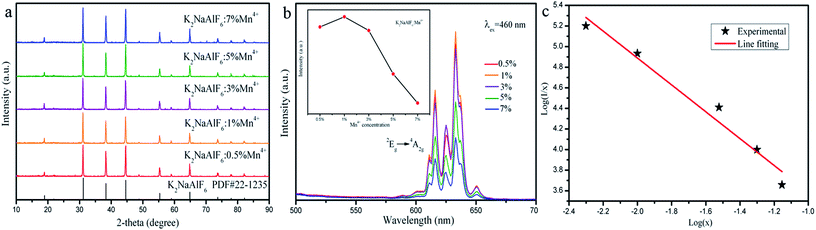 |
| Fig. 8 (a) XRD patterns, (b) emission spectra (λex = 460 nm) of the as-synthesized K2NaAlF6:xMn4+ (x = 0.5%, 1%, 3%, 5% and 7%), the inset photograph represents emission intensity of Mn4+ as a function of Mn4+ doping concentration, and (c) the relation plot of log(I/x) on log(x) of Mn4+ doping concentration x. | |
Fig. 8(c) shows the dependence of log(I/x) on log(x) with a slope of (−θ/3). A relative linear fitted can be obtained and the result of the slope is about 1.3. The value of θ is calculated approximately 6. Therefore, the mechanism of the concentration quenching of Mn4+ is d–d interaction in K2NaAlF6 host.
3.3 Temperature stability properties
The thermal stability of the phosphors is frequently used as an important parameter in LED applications. In order to investigate the influence of temperature on the luminescence of K2NaAlF6:Mn4+ phosphor, a series of emission spectra for K2NaAlF6:Mn4+ in the temperature range of 98–498 K was shown in Fig. 9(a). All the emission peaks are in same shape. As an increase of temperature, the positions of the emission peak have no obvious shift with the strongest emission peak at ∼633 nm. The luminous intensity displays a decreasing trend with an increase in temperature from 98 K to 498 K. Fig. 9(b) displays the relative intensity trend at 633 nm of K2NaAlF6:Mn4+. Increasing the temperature, the luminous intensity decreases gradually. Upon heating the phosphor to 448 K, the emission intensity remains approximately 65% of the emission intensity at 298 K. The phenomenon of thermal quenching is attributed to the non-radiative transition, which can be fitted by the following equation:39
where IT is the intensity at temperature T, I0 is the initial intensity, c is the frequency factor, ΔE is the activation energy of the thermal quenching and K is the Boltzmann constant (8.629 × 10−5 eV K−1). According to the above equation, the activation energy of the as-prepared K2NaAlF6:Mn4+ red phosphor is 0.3 eV, suggesting the excellent thermal stability. The phenomenon of thermal quenching can be illustrated by configuration coordinate diagram (Fig. 9(c)). Under near UV and blue light excitation, the electrons are excited to the excited state, from 4A2g to 4T1g and 4T2g levels of Mn4+ ions in K2NaAlF6 hosts. Subsequently, the electrons of 2Eg level transit to the ground state 4A2g level by radiative transition, which brings out the red emission of Mn4+ ions. As the temperature increases, a part of the electrons absorbing activation energy ΔE go back to the ground state via the crossover point of the states of 4T2g and 4A2g, resulting in non-radiation transition to ground state 4A2g and the formation of thermal quenching.
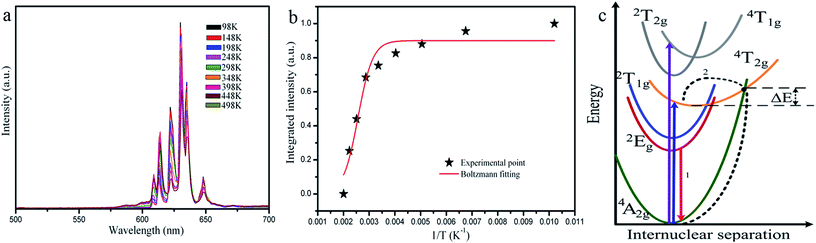 |
| Fig. 9 (a) Emission spectra of K2NaAlF6:Mn4+ upon excitation of 460 nm, (b) temperature dependence of the relative intensity of 2Eg → 4A2g transition of Mn4+ in K2NaAlF6, and (c) configurational coordinate diagram for illustration the possible thermal quenching process of Mn4+ in the K2NaAlF6 host. | |
3.4 Luminescence performance of WLEDs
To validate the availability of K2NaAlF6:Mn4+ for the blue-based white LED application, the synthesized red phosphor K2NaAlF6:Mn4+ was mixed with the YAG:Ce3+ and the mixture coupled with a 460 nm blue LED to fabricate a WLED. Fig. 10(a) displays the electroluminescence spectra of the blue light chip, LED composed of blue light chip and YAG:Ce3+, YAG:Ce3+–K2NaAlF6:Mn4+ under 20 mA current excitation. The emission peak of blue light chip is ∼460 nm, and it overlaps with the excitation spectrum of red phosphor K2NaAlF6:Mn4+. Compared with the LED prepared by combining with YAG:Ce3+, the prepared LEDs that blends YAG:Ce3+ and K2NaAlF6:Mn4+ shows a warm white light property by the naked eye. The CIE chromaticity diagram and the object photograph of the LEDsdevices are exhibited in Fig. 10(b). The related photoelectric parameters such as correlated color temperature (CCT), color rendering index (CRI) and luminous efficacy of these LEDs, are listed in Table 1. The corresponding CIE coordinates of LEDs shift from cool white light (0.3530, 0.3956) to warm white light (0.3759, 0.4075). The CCT of the WLEDs is dropped from 4882 K to 4310 K, and the CRI improves from 70.9 to 78.7. However, the luminous efficiency of the LEDs decreases from 108.23 lm W−1 to 60.22 lm W−1. These results indicate that the red phosphors can enhance LED performance and have potential applications in the field of indoor lighting.
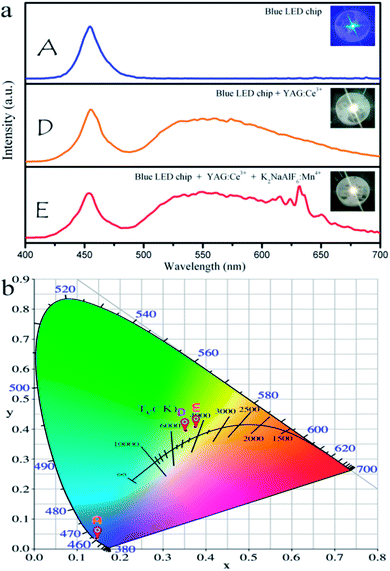 |
| Fig. 10 (a) Electroluminescence spectra of blue chip, LED assembling the blue light chip – YAG:Ce3+, and YAG:Ce3+–K2NaAlF6:Mn4+ under 20 mA current excitation. (b) The corresponding CIE chromaticity coordinates of LED devices. | |
Table 1 Measured photometric and chromaticity for the LEDs
Sample |
CIE coordinates (x, y) |
CCT |
Ra |
Luminous efficiency |
A |
0.1460, 0.0359 |
|
|
24.52 lm W−1 |
D |
0.3530, 0.3956 |
4882 K |
70.9 |
108.23 lm W−1 |
E |
0.3759, 0.4075 |
4310 K |
78.7 |
60.22 lm W−1 |
4 Conclusions
In summary, a class of red phosphor K2NaAlF6:Mn4+ was prepared by hydrothermal method, and its crystal structure, morphology, composition, optical properties were characterized. Under excitation of blue light, K2NaAlF6:Mn4+ can give a red emission located at 633 nm ascribed to the transition of 2Eg → 4A2g. The influence of synthesis conditions, including the ratio of reaction materials, reaction temperatures and times, the concentrations of Mn4+ ions, on the crystal structure and optical properties of the K2NaAlF6:Mn4+ had been discussed carefully. Moreover, the critical distance between adjacent Mn4+ in the K2NaAlF6 host was calculated. The concentration quenching phenomenon appearing in the K2NaAlF6:Mn4+ is attributed to dipole–dipole interaction. The temperature dependent emission intensity shows that K2NaAlF6:Mn4+ has excellent thermal stability and is suitable for the working temperature of LEDs. Furthermore, the warm white LED is fabricated by coupling the 460 nm blue chip with a mixture of yellow (YAG:Ce3+) and red (K2NaAlF6:Mn4+) phosphor, which has lower color temperature (4310 K), higher color rendering index (Ra = 78.7) and luminous efficacy of 60.22 lm W−1. Therefore, K2NaAlF6:Mn4+ is a promising red phosphor for warm white LEDs.
Conflicts of interest
There are no conflicts to declare.
Acknowledgements
This work was financially supported by the National Natural Science Foundation of P. R. China (NSFC) (Grant No. 51072026, 51573023) and the Development of science and technology plan projects of Jilin province (Grant No. 20170101185JC).
Notes and references
- Y. Wang, T. Wen, L. Tang, L. Yang, W. Yang and Y. Zhao, Dalton Trans., 2015, 44, 7578–7585 RSC.
- M. J. Lee, Y. H. Song, Y. L. Song, G. S. Han, H. S. Jung and D. H. Yoon, Mater. Lett., 2015, 141, 27–30 CrossRef CAS.
- L. Lv, X. Jiang, S. Huang, X. A. Chen and Y. Pan, J. Mater. Chem. C, 2014, 2, 3879–3884 RSC.
- S. Zhang, Y. Hu, H. Duan, Y. Fu and M. He, J. Alloys Compd., 2017, 693, 315–325 CrossRef CAS.
- Z. Wang, Y. Zhou, Y. Liu, Q. Zhou, L. Luo, H. Tan, Q. Zhang, G. Chen and J. Peng, RSC Adv., 2015, 5, 82409–82414 RSC.
- X. Gao, Y. Song, G. Liu, X. Dong, J. Wang and W. Yu, Dalton Trans., 2016, 45, 17886–17895 RSC.
- R. Cao, J. Huang, X. Ceng, Z. Luo, W. Ruan and Q. Hu, Ceram. Int., 2016, 42, 13296–13300 CrossRef CAS.
- H. Tan, M. Rong, Y. Zhou, Z. Yang, Z. Wang, Q. Zhang, Q. Wang and Q. Zhou, Dalton Trans., 2016, 45, 9654–9660 RSC.
- T. T. Deng, E. H. Song, J. Sun, L. Y. Wang, Y. Deng, S. Ye, J. Wang and Q. Y. Zhang, J. Mater. Chem. C, 2017, 5, 2910–2918 RSC.
- E. Song, J. Wang, J. Shi, T. Deng, S. Ye, M. Peng, J. Wang, L. Wondraczek and Q. Zhang, ACS Appl. Mater. Interfaces, 2017, 9, 8805–8812 Search PubMed.
- X. Ding, Q. Wang and Y. Wang, Phys. Chem. Chem. Phys., 2016, 18, 8088–8097 RSC.
- S. Zhang, Y. Hu, H. Duan, L. Chen, Y. Fu, G. Ju, T. Wang and M. He, RSC Adv., 2015, 5, 90499–90507 RSC.
- X. Jiang, Y. Pan, S. Huang, X. A. Chen, J. Wang and G. Liu, J. Mater. Chem. C, 2014, 2, 2301–2306 RSC.
- Y. Jin, Y. Hu, H. Wu, H. Duan, L. Chen, Y. Fu, G. Ju, Z. Mu and M. He, Chem. Eng. J., 2016, 288, 596–607 CrossRef CAS.
- S. P. Singh, M. Kim, W. B. Park, J.-W. Lee and K.-S. Sohn, Inorg. Chem., 2016, 55, 10310–10319 CrossRef CAS PubMed.
- J. Long, Y. Wang, R. Ma, C. Ma, X. Yuan, Z. Wen, M. Du and Y. Cao, Inorg. Chem., 2017, 56, 3269–3275 CrossRef CAS PubMed.
- X. Jiang, Z. Chen, S. Huang, J. Wang and Y. Pan, Dalton Trans., 2014, 43, 9414–9418 RSC.
- R. Cao, X. Ceng, J. Huang, H. Ao, G. Zheng, X. Yu and X. Zhang, Opt. Mater., 2016, 62, 706–710 CrossRef CAS.
- X. Ding, G. Zhu, W. Geng, Q. Wang and Y. Wang, Inorg. Chem., 2016, 55, 154–162 CrossRef CAS PubMed.
- X. Q. Li, X. Su, P. Liu, J. Liu, Z. Yao, J. Chen, H. Yao, X. Yu and M. Zhan, CrystEngComm, 2015, 17, 930–936 RSC.
- H. Chen, H. Lin, Q. Huang, F. Huang, J. Xu, B. Wang, Z. Lin, J. Zhou and Y. Wang, J. Mater. Chem. C, 2016, 4, 2374–2381 RSC.
- H. Zhu, C. C. Lin, W. Luo, S. Shu, Z. Liu, Y. Liu, J. Kong, E. Ma, Y. Cao, R.-S. Liu and X. Chen, Nat. Commun., 2014, 5, 4312 CAS.
- Y. Jin, Y. Fu, Y. Hu, L. Chen, H. Wu, G. Ju, M. He and T. Wang, Powder Technol., 2016, 292, 74–79 CrossRef CAS.
- Y. Jin, M.-H. Fang, M. Grinberg, S. Mahlik, T. Lesniewski, M. G. Brik, G.-Y. Luo, J. G. Lin and R.-S. Liu, ACS Appl. Mater. Interfaces, 2016, 8, 11194–11203 Search PubMed.
- L.-L. Wei, C.-C. Lin, M. H. Fang, M. G. Brik, S.-F. Hu, H. Jiao and R.-S. Liu, J. Mater. Chem. C, 2015, 3, 1655–1660 RSC.
- Q. Shao, L. Wang, L. Song, Y. Dong, C. Liang, J. He and J. Jiang, J. Alloys Compd., 2017, 695, 221–226 CrossRef CAS.
- B. Wang, H. Lin, F. Huang, J. Xu, H. Chen, Z. Lin and Y. Wang, Chem. Mater., 2016, 28, 3515–3524 CrossRef CAS.
- C. Yang, Z. Zhang, G. Hu, R. Cao, X. Liang and W. Xiang, J. Alloys Compd., 2017, 694, 1201–1208 CrossRef CAS.
- Q. Zhou, Y. Zhou, Y. Liu, L. Luo, Z. Wang, J. Peng, J. Yan and M. Wu, J. Mater. Chem. C, 2015, 3, 3055–3059 RSC.
- H.-D. Nguyen, C. C. Lin, M.-H. Fang and R.-S. Liu, J. Mater. Chem. C, 2014, 2, 10268–10272 RSC.
- Y. Zhu, J. Yu, Y. Liu, M. G. Brik, L. Huang, T. Xuan and J. Wang, RSC Adv., 2017, 7, 30588–30593 RSC.
- L. Huang, Y. Zhu, X. Zhang, R. Zou, F. Pan, J. Wang and M. Wu, Chem. Mater., 2016, 28, 1495–1502 CrossRef CAS.
- E. H. Song, J. Q. Wang, S. Ye, X. F. Jiang, M. Y. Peng and Q. Y. Zhang, J. Mater. Chem. C, 2016, 4, 2480–2487 RSC.
- H. Bode, H. Jenssen and F. Bandte, Angew. Chem., 1953, 65, 304 CrossRef CAS.
- T.-C. Lang, T. Han, L.-L. Peng and M.-J. Tu, Mater. Chem. Front., 2017, 1, 928–932 RSC.
- Y. Zhu, L. Huang, R. Zou, J. Zhang, J. Yu, M. Wu, J. Wang and Q. Su, J. Mater. Chem. C, 2016, 4, 5690–5695 RSC.
- G. Blasse and G. J. Dirksen, J. Solid State Chem., 1986, 65, 283–286 CrossRef CAS.
- X. Gao, Y. Song, G. Liu, X. Dong, J. Wang and W. Yu, CrystEngComm, 2016, 18, 5842–5851 RSC.
- S.-S. Liang, M.-M. Shang, H.-Z. Lian, K. Li, Y. Zhang and J. Lin, J. Mater. Chem. C, 2017, 5, 2927–2935 RSC.
|
This journal is © The Royal Society of Chemistry 2017 |
Click here to see how this site uses Cookies. View our privacy policy here.